Does triclosan adsorption on polystyrene nanoplastics modify the toxicity of single contaminants?†
Received
18th September 2020
, Accepted 1st December 2020
First published on 8th December 2020
Abstract
The physical and chemical properties of nanoplastics make them potential carriers for some environmental contaminants, modifying their biological effects. Nevertheless, the change in toxicity caused by pollutant adsorption on nanoplastics is still controversial, depending on the interactions between chemical and physical pollutants, the consequent change in bioavailability, the modification of intake, transport and accumulation in the organisms and also on the characteristics of contaminants. In this context, the aim of the present study was the evaluation of combined effects made by 0.5 μm nanobeads of polystyrene and triclosan adsorbed on their surface in comparison with those caused by single contaminants. The systemic effects of 7 day exposure to nanoplastics, triclosan alone and to the nanoplastic–triclosan complex have been analyzed by employing zebrafish larvae and using a multi-tier approach from the evaluation of cellular and molecular effects to the impact at organism level. Results highlighted by confocal microscopy evidenced nanobead ingestion and translocation in several tissues and organs to guarantee the goodness of the exposure results. Behavioral assays were then conducted to highlight larval swimming defects as a ‘real-time’ readout of the potential effects on the whole organism, while a suite of several biomarkers and functional proteomics was applied to investigate the effects at both cellular and molecular levels. The whole data set pointed out a clear modification in the toxicological effects of the nanoplastic–triclosan complex in comparison with single contaminants, proved by opposite behaviours in the larval swimming activity and modulation of diverse protein classes as well as by different effects on several biochemical endpoints. This means that the interaction between chemical and physical pollutants leads to more complicated responses than additive, synergistic or antagonist models, resulting in a modification of toxicity instead of its increase or decrease.
Environmental significance
The physicochemical properties of nanoplastics (NPs) make them very suitable for the transfer of chemical contaminants, potentially enhancing their risk to aquatic organisms. Nevertheless, a few controversial pieces of evidence that exist relied on the toxic effects caused by NP adsorption of environmental pollutants. To the best of our knowledge, this is the first study assessing the capability of polystyrene nanoparticles to adsorb triclosan (TCS), a known toxic chemical compound, and investigating the effect of the NP–TCS complex and each individual contaminant on a freshwater model. The results pointed out a clear shift in the toxicity when chemical and physical pollutants are bound together, rather than a simple additive, synergistic or competitive effect, suggesting the need to employ more complex interpretative models.
|
1. Introduction
The invention of plastic surely represented one of the major technological innovations that changed our lifestyle and improved our wellness. Their versatile and unique characteristics (e.g. light weight, durability) allowed the application of plastic materials in several sectors, from the construction and transport industries to electrical and electronic applications as well as food conservation and healthcare. The incorrect disposal of plastic products is causing their increasing release in terrestrial and aquatic environments, since 79% of the plastic waste ever produced was accumulated in the natural environments or landfills, while about 12% was incinerated and only 9% was recycled.1
Once in the environment, larger plastic items can be fragmented into smaller debris by sunlight, mechanical abrasion, salinity and temperature variations.2 These modifications can profoundly change the fate and toxicity of plastics, being more easily ingested and accumulated in organisms. These smaller plastic pieces are called microplastics (MPs) and nanoplastics (NPs), which have been recently redefined as debris with a dimension between 1 μm to <1 mm and 1 nm to <1 μm, respectively.3 Due to their smaller dimension, these plastic debris represents the most dangerous fraction, since they can be potentially ingested by all aquatic and terrestrial organisms. Indeed, there is much evidence of the negative effects caused by MPs and NPs at various levels of the biological organization.4–10 The next challenge is represented by the joint effects due to the interaction between MPs, NPs and chemical pollutants present in the environment, which can be adsorbed on the surface of plastic debris. Indeed, their high surface/volume ratio increases the sorption of other contaminants present in the surrounding matrix, making MPs and NPs suitable as physical carriers for these pollutants through all the environmental compartments, biota included. Since MPs and NPs have properties similar to natural suspended matter, they possess a higher sorption capability for hydrophobic contaminants, such as polychlorinated biphenyls (PCBs), polycyclic aromatic hydrocarbons (PAHs) and other benzene-ring derivatives.11 Many studies demonstrated the capability of MPs and NPs to be a carrier also for some hydrophilic pollutants due to the generation of oxidative groups during plastic debris weathering that increase their polarity, roughness and porosity,12,13 as demonstrated for the adsorption on some MPs of perfluoro-octane-sulfonate (PFOS) and perfluoro-octane-sulfonamide (PFOA)14 as well as of many antibiotics.15,16 This means that smaller plastic debris can modify the overall bioavailability of the environmental contaminants for the organisms, magnifying or even decreasing the toxicity of pollutants bound together, as a consequence of the possible different relations in the chemical–plastic–organism ternary system.17
In this context, the aim of our study was the evaluation of the possible change in toxicity after the adsorption of triclosan (TCS) on 0.5 μm PS NPs (PNPs) in comparison with the effects evaluated after the exposure to single contaminants, by using zebrafish (Danio rerio) larvae as aquatic model organisms. TCS is one of the current most investigated environmental pollutants due to its wide use in many consumer products (soaps, toothpastes, deodorants, textiles, shoes, toys, cosmetics) and the increasing evidence of its negative effects on the health of organisms, including humans. Being a hydrophobic chemical (log
Kow = 4.76), TCS is a persistent environmental pollutant still present in natural environments in a concentration up to 5.2 μg L−1 in surface waters worldwide.18 From the (eco)toxicological point of view, TCS and its by-products were reported to be endocrine disruptors in several model organisms, to affect immune responses, reactive oxygen species (ROS) production and also cardiovascular functions.19 The ubiquitous presence of TCS in all the aquatic ecosystems worldwide makes this lipophilic contaminant prone to be transported by MPs and NPs, changing not only its environmental fate but also the intake, accumulation and probably toxicity towards organisms.
Since the possible interaction between MPs, NPs and environmental contaminants has been conducted up to now using only the chemicals described above, our study represents a novelty for TCS, whose adsorption on plastic debris (polyethylene) has already been detected by Wu et al.20
Our experimental design comprised three different steps: (1) we firstly planned the sample preparation in order to eliminate the interference caused by TCS and PNPs freely present in the water when in co-exposure, through a preliminarily adsorption of an environmental concentration of TCS (0.6 μg L−1) on PNPs (200 μg L−1); (2) we then evaluate the ingestion, uptake and accumulation of virgin and doped PNPs in tissues and organs of zebrafish larvae by confocal microscopy; (3) lastly, we exposed zebrafish larvae to PNPs and TCS administered alone and to the PNP–TCS complex for 7 days, measuring many endpoints belonging to different levels of the biological organization. The multi-tier approach was based on the evaluation of possible alterations in swimming behavior as the endpoint to assess the pollutants' effects at a whole organism level, while a biomarker suite and functional proteomics were carried out to investigate the effects at cellular and molecular levels.
To the best of our knowledge, this is the first multidisciplinary study aimed to assess the potential of NPs to be a carrier for this antibacterial chemical, evaluating at the same time the possible change in toxicity, since there is only the study by Syberg et al.21 describing the combined effect of a mixture of TCS and polyethylene microbeads administered to the marine copepod Acartia tonsa, based however only on mortality as the measured endpoint.
2. Experimental
2.1 PNP characterization
PNPs within the nanosize range of 0.4–0.6 μm (10% w/v) and fluorescent pink PNPs (1% w/v) with a comparable size (0.5 μm) were purchased from Spherotech Inc. (Lake Forest, IL, USA). Fluorescent nanobeads were only administered to larvae designed for confocal microscopy observations. Detailed information about PNP characterization (size distribution, Z-potential, excitation and emission spectra) are shown in the ESI† (Table S1 and Fig. S1). Both PNP stock solutions contained sodium azide (0.02%) to prevent bacterial growth, whose concentration decreased to 0.00001% in the working solution used for the exposure assays. Nevertheless, we previously certified that this very low concentration of bacteriostatic additive did not affect the investigated endpoints.9
2.2 TCS adsorption on PNPs and selection of concentrations
Before the exposures, both the non-fluorescent and fluorescent PNPs were doped with TCS to obtain the TCS-contaminated plastic suspensions, thus creating a PNP–TCS single complex which was then administered to zebrafish larvae. This experimental plan allowed the elimination from the PNP–TCS exposure solution of the two interfering fractions constituted by the TCS and PNPs alone.
TCS contamination was performed by adding PNPs (25 mg) and TCS (250 μg) in 3 glass bottles with 50 mL of deionized water and kept in constant mixing by a stirrer at 4 °C for 3 days in the dark. Then, the PNP–TCS suspension was centrifuged for 15 min at 3000g and 25 °C to precipitate the nanobeads. The supernatants were collected and used to evaluate the fraction of TCS not adsorbed on the PNPs, while the pellets containing the doped PNPs were gently dried under a nitrogen flow and kept in glass vials until the exposure assays.
In order to check the fraction of TCS adsorbed on PNPs, each pellet was resuspended in methanol and the suspension was ultrasonicated for 30 min to re-separate the adsorbed TCS from the PNPs. The solution was then filtered (mesh = 0.2 μm) to completely remove the remaining suspended PNPs. This solution, containing the TCS re-separated from PNPs, and the supernatants previously sampled, were analyzed by liquid–liquid extraction (isooctane/methanol 4
:
1 v/v and hexane/water 2
:
1 v/v) followed by a gas chromatographic analysis (TRACE GC coupled with a PolarisQ ion trap mass spectrometer, Thermo-Electron, Texas, USA). We calculated a concentration of 82 μg L−1 of TCS in the final solution, which was equal to 36% of the initial concentration (250 μg L−1), representing the percentage of TCS adsorbed on PNPs. Therefore, to remain within the environmental concentrations of this chemical, the PNP amount for the exposure was set to 200 μg L−1, following the calculation shown in Table 1. This concentration corresponded to 0.6 μg L−1 adsorbed TCS. Consequently, the same TCS concentration was used when the chemical was administered alone to zebrafish larvae.
Table 1 Concentrations of PNPs and TCS used for the preparation of TCS-contaminated particle suspensions
|
Preparation solutions |
Final concentration of solution |
Exposure concentrations |
Stock solution |
Working solution |
Extract |
|
PNPs |
100 g L−1 |
2.5 g L−1 |
— |
200 μg L−1 |
TCS |
1 mg L−1 |
250 μg L−1 |
82 μg L−1 |
0.6 μg L−1 |
2.3 Exposure of zebrafish larvae
AB strain adult zebrafish were reared in the facility at the Department of Biosciences, University of Milan, following Italian laws, rules and regulations (Legislative Decree no. 116/92). In accordance with the Italian Legislative Decree 26/2014, the project was carried out under the authorization of the Italian Ministry of Health (Aut. Min. no. 6/2019-PR).
We performed three independent experiments using different clutches of larvae from different fish to increase biological variability. Zebrafish larvae were exposed for 7 days, starting from the age of 4 days post fertilization (dpf) inside 800 mL glass beakers (approximately 100 larvae in 500 mL of zebrafish water in each beaker) and maintained at 28 °C on a 14 h light
:
10 h dark cycle. The exposures were carried out under semi-static conditions, changing the medium every 24 h and maintaining the water in constant mixing. Larvae were fed with commercial artificial diets (particle size <100 μm) from 6 dpf. Control larvae (CTRL) were maintained in fish water (0.1 g L−1 Instant Ocean, 0.1 g L−1 NaHCO3, 0.2 g L−1 CaSO4 in deionized water with 0.1% methylene blue), while the three treatments consisted of (i) 0.6 μg L−1 TCS in zebrafish water, (ii) 200 μg L−1 PNPs (corresponding to about 3 × 109 nanobeads per L), and (iii) 200 μg L−1 of the complex PNP–TCS.
At the end of exposures, larvae were sacrificed in tricaine (300 mg L−1) and immediately processed for the following microscopy observations, genotoxicity assessments and behavioral tests, or stored at −80 °C for further biomarker and proteomic analyses.
In the meantime, PNP–TCS suspensions and TCS solutions collected after 24 h in the exposure beakers were collected and analyzed in order to check for any TCS release from the particles as well as the eventual reduction in TCS concentration after 24 h of exposure. We used the same method applied for the quantification of TCS adsorption (liquid–liquid extraction in hexane/water 2
:
1 v/v followed by a gas chromatographic analysis).
2.4 Confocal microscopy
The ingestion and accumulation of fluorescent PNPs (alone and combined with TCS) in zebrafish larvae were investigated by confocal microscopy technique. At the end of exposures, 20 larvae for each independent experiment were sacrificed as described above and fixed in paraformaldehyde (4% in PBS), then stored at 4 °C. Larvae selected for whole-mount observations were treated with protease K (P2308, Sigma Aldrich), stained with DAPI (4′,6-diamidino-2-phenylindole) and directly mounted on microscope slides, while larvae designed for cryo-sectioning were washed in PBS (0.1 M) and sucrose (15% and 30%), included in the mounting medium (Bio Optica), frozen in dry ice and stored at −80 °C. Longitudinal and transversal sections (15 μm) were prepared using a Cryostat CM 1850 (Leica, Wetzlar, Germania) at −20 °C. Cell nuclei were stained with DAPI.
For each experimental group (CTRL, PNPs and PNP–TCS) we observed 10 whole-mount larvae and 10 slides of larval sections using a confocal microscope (laser scanning confocal microscope, Nikon A1) to localize the internalized fluorescent PNPs in the exposed organisms. Assessment of the orthogonal projections of Z-stack pictures guaranteed that PNPs were effectively inside the tissues and not a simple artefact due to the transport made by the blade of the cryostat.
2.5 Larval swimming activity
Potential behavioral alterations induced by the three different treatments were evaluated on 12 larvae for each experimental group (36 total larvae for each treatment) using the DanioVision™ tracking system (Noldus IT, Wageningen, Netherlands).
Each larva was put in a single well of a 24-multiwell plate and submitted to an alternating dark/light test based on 10 minutes of adaptation followed by 2 cycles of 20 min of dark and 10 min of light,9 recording their swimming activity at 30 frames per s. During the entire test, the temperature was maintained at 28 °C by the DanioVision temperature control unit.
The acquired data were analyzed using the software EthoVision XT (Noldus IT, Wageningen, Netherlands) by measuring the following parameters: total distance moved (mm), turn angle (degree) and thigmotaxis (wall-hugging behavior). The software was also applied to create and visualize graphical representations of larval swimming activity, such as tracks (Fig. S3A†) and heatmaps (Fig. S3B†), where different colors represented the frequency of a specific position (hotspots) in the well.
2.6 Biomarker
Biomarker methods are thoroughly described in the ESI.† Briefly, cell viability was assessed by the trypan blue dye exclusion method before the genetic damage tests to guarantee a viability higher than 80%.22 Genotoxicity assessments were performed according to Parolini et al.23 by using pools of 7 larvae for each experimental group (3 pools of 7 individuals for each treatment). Genotoxicity was evaluated by measuring the frequency of apoptotic and necrotic cells as well as micronuclei (MN) which pointed out fixed and irreversible DNA damage.
The effects on detoxification systems were assessed by ethoxyresorufin-O-deethylase (EROD) and glutathione S-transferase (GST) activities, while the oxidative stress level was evaluated by superoxide dismutase (SOD), catalase (CAT) and glutathione peroxidase (GPx) activities and by the measurement of reactive oxygen species (ROS) production on pools of 35 larvae from each experimental group (3 pools of 35 individuals for each treatment).
Lastly, the presence of neurotoxic effects was assessed by the level of acetylcholinesterase (AChE) activity, using 5,5′-dithiobis-2-nitrobenzoic acid (DTNB) as reagent.7 Due to the strong connection between neuronal alterations and behavior, for this test we pooled together larvae previously tested for behavioral changes (36 total larvae for each treatment).
All data resulting from enzymatic analyses and ROS evaluation were normalized to the protein total content of each sample measured by the Bradford method.24
2.7 Functional proteomics
The modulation of protein expression in control and treated larvae was investigated by the gel-free proteomic technique.8 At the end of the exposure, pools of 20 larvae for each experimental group (3 pools of 20 individuals for each treatment) were homogenized in 300 μL of lysis buffer (described in the ESI†). Proteins were precipitated, reduced, alkylated and then digested by adding trypsin. Peptides were then purified by reverse phase chromatography, using Zip Tips (μ-C18; Millipore, Milan, Italy),8 and analyzed with a mass spectrometer as reported by Magni et al.8 with some modifications (described in the ESI†).
2.8 Statistical analysis
Statistical analyses on behavior and biomarker data were performed using STATISTICA 7.0 software. After having checked the normality and homoscedasticity of biomarker data, the significance was evaluated by one-way analysis of variance (ANOVA) followed by Duncan's multiple range post hoc test (DMRT), taking p < 0.05 as the significance cut-off. Regarding the proteomic results, a Student t-test was performed between control and treated samples.
3. Results
3.1 Check of the experimental conditions
The check of the possible release of TCS from the doped PNPs by gas chromatographic analyses showed the complete absence of the chemical in the exposure beakers after 24 h of exposure, pointing out that TCS did not detach from the particles. This means that the ecotoxicological effects due to the TCS–PNP complex presented below are certainly due to the combination of the two contaminants because our experimental design was able to eliminate the interference due to TCS and PNPs present alone in the exposure suspensions. Furthermore, the analyses of TCS concentration in the exposure solutions after 24 h revealed a decrease of about 30% (0.4 μg L−1) in the beakers containing 0.6 μg L−1 TCS alone, confirming the need to perform the exposures under semi-static conditions by a daily renewal of chemical.
3.2 PNP uptake and accumulation in zebrafish larvae
Whole-mount observations by confocal microscopy confirmed the PNP uptake by larvae, both for virgin PNPs and the PNP–TCS complex (Fig. 1). On the other hand, the capability of PNPs to pass through biological membranes and accumulate in different tissues was already demonstrated also for zebrafish embryos.9 Nevertheless, new findings have emerged from larval section observations, since we visualized PNPs both in the eye (Fig. 2) and in the trunk (Fig. 3). Furthermore, we did not observe any differences in the amount of ingested PNPs between virgin ones and the PNP–TCS complex, suggesting that TCS adsorption did not modify the particle intake.
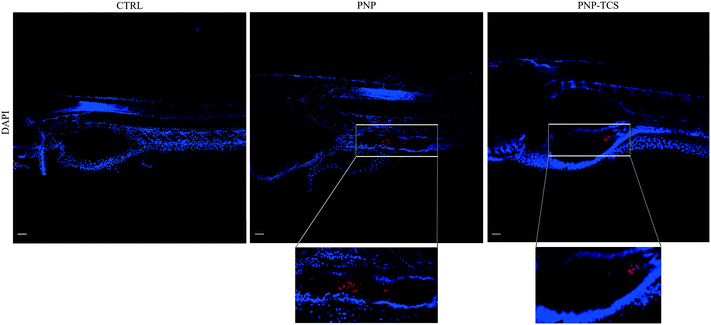 |
| Fig. 1 Whole-mount images of larvae at the end of the exposure (scale bar 50 μm). Nanobeads are shown in red. Cell nuclei are stained with DAPI. | |
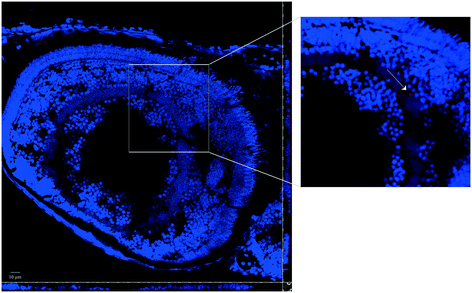 |
| Fig. 2 Detail of a cryostat section (11 Z-steps of 1 μm) showing the eye of a zebrafish larva exposed to PNP–TCS. Nanobeads are shown in red. Cell nuclei are stained with DAPI. Orthogonal projections of Z-stack evidencing the nanobead at cellular level. | |
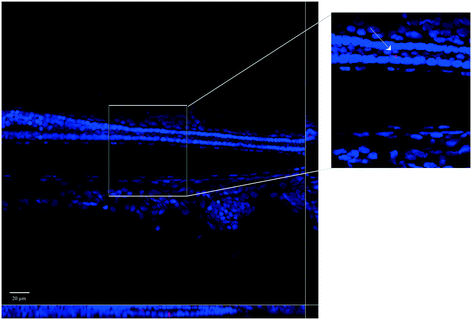 |
| Fig. 3 Detail of a cryostat section (14 Z-steps of 1 μm) showing the tail of a zebrafish larva exposed to PNPs. Nanobeads are shown in red. Cell nuclei are stained with DAPI. Orthogonal projections of Z-stacks evidencing the nanobead at cellular level. | |
3.3 Effects at individual level
At the end of exposures, the effects of the three treatments on fish behavior were evaluated by measuring their swimming activity. Results highlighted an opposite effect of TCS when administered alone or combined with PNPs. Indeed, we observed both a hypo- and a hyperactivity of zebrafish larvae compared to controls, respectively (Fig. 4A). In detail, the variation was significant both for the dark (F3,156 = 21.28 and p < 0.001) and the light phase (F3,74 = 50.15 and p < 0.001; Fig. 4B). By contrast, PNPs administered alone did not significantly affect the total distance travelled by larvae.
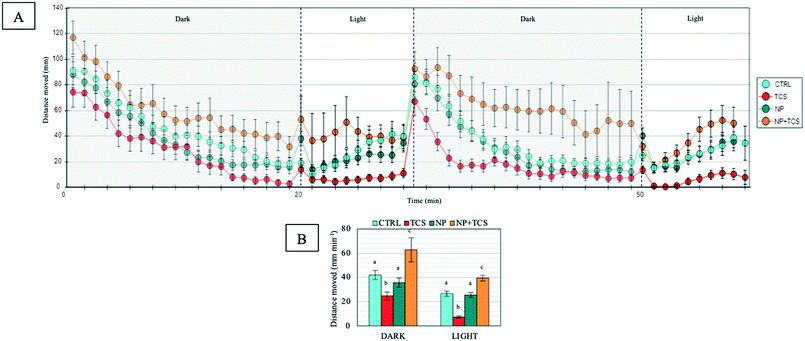 |
| Fig. 4 Swimming activity (distance moved over time) measured in zebrafish larvae exposed to PNPs (200 μg L−1), TCS (0.6 μg L−1) and PNP–TCS, compared to control. (A) Total distance moved per minute (mean ± S.E.); (B) total distance moved during dark and light conditions (mean ± S.E.). Different letters correspond to values significantly different (one-way ANOVA and DMRT post hoc test, p < 0.05). | |
Regarding the other considered parameters, such as turn angle (Fig. S2A†) and thigmotaxis (Fig. S2B†), no significant differences were observed in all exposure conditions.
3.4 Effects at sub-organism level
Biomarker analyses pointed out significant variations of MN frequency (F3,20 = 4.92 and p < 0.05), as well as GST (F3,19 = 5.75 and p < 0.01) and GPx activities (F3,20 = 3.64 and p < 0.05) (Fig. 5). In detail, we observed a significant increase (p < 0.01) of MN frequency due to TCS administered alone compared to controls, while a significant decrease was found in PNPs (p < 0.05) and PNP–TCS (p < 0.05) compared to TCS. Regarding oxidative endpoints, a significant increase of GST and GPx activity was detected in organisms exposed to TCS compared to controls (p < 0.01 and p < 0.05, respectively). The measured activities of these enzymes were also significantly different between PNP-treated and TCS-treated groups. In detail, GST activity decreased in PNPs and PNP–TCS compared to TCS (p < 0.01 and p < 0.05, respectively), while GPx activity decreased in PNP–TCS compared to TCS (p < 0.05). Lastly, any significant effects of PNPs, both virgin and contaminated, were pointed out by biomarker analyses.
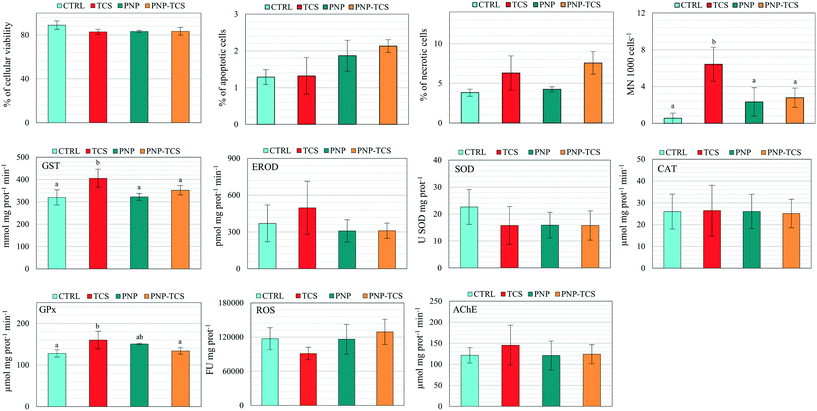 |
| Fig. 5 Cellular effects measured in zebrafish larvae exposed to PNPs (200 μg L−1), TCS (0.6 μg L−1) and PNP–TCS, compared to control (pool of 3 independent experiments). Different letters correspond to values significantly different (one-way ANOVA and DMRT post hoc test, p < 0.05). | |
3.5 Effects on proteome
We identified 1807 proteins, 1480 of which were subsequently quantified. In detail, PNPs modified 43 proteins, 8 of which were significantly different (p < 0.05) from controls, but not above the 2-fold change threshold (Fig. S4A†). The remaining 35 proteins were quite equally divided between up-regulated (16) and down-regulated (19) proteins (Fig. 6A).
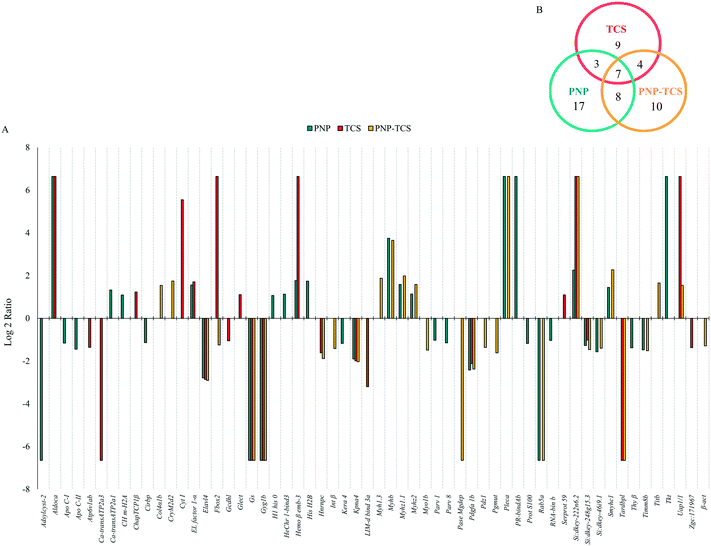 |
| Fig. 6 (A) Protein modulation measured in zebrafish larvae exposed to PNPs (200 μg L−1), TCS (0.6 μg L−1) and PNP–TCS, compared to control. (B) Venn diagram. Adsylcyst-2: S-adenosylhomocysteine hydrolase-like 2; aldoca: fructose-bisphosphate aldolase; APO C-I: apolipoprotein C-I; APO C-II: apolipoprotein C-II; Atp6v1ab: ATPase H+-transporting V1 subunit ab; CA-transATP2a3: calcium-transporting ATPase gn = ATP2a3; CA-transATP2a1: calcium-transporting ATPase gn = ATP2a1; ch m-h2a: core histone macro-h2a; ChapTCP1β: chaperonin containing TCP1, subunit 2 (beta); Cirbp: Cirbp protein; Col4α1b: collagen, type XIV, alpha 1b; CryM2d2: crystallin, gamma M2d2; Cyt i: type I cytokeratin; El factor 1-α: elongation factor 1-alpha; Elavl4: Elav-like protein; Fbxo2: F-box protein 2; Gcdhl: glutaryl-CoA dehydrogenase b; Glect: galectin; Gs: glutamine synthetase; Gyg1b: glycogenin 1b; H1 his 0: H1 histone family, member 0; Hechr 1-bind3: heterochromatin protein 1, -binding protein 3; hemo β Emb-3: hemoglobin beta embryonic-3; his H2b: histone H2b; Hnrnpc: heterogeneous nuclear ribonucleoprotein C1/C2; int β: integrin beta; Kera 4: keratin 4; Kpna4: importin subunit α; Lim-d bind 3a: Lim-domain binding factor 3a; Myh1.3: myosin, heavy polypeptide 1.3, skeletal muscle; Myhb: myosin, heavy chain b; Myhz1.1: myosin, heavy polypeptide 1, skeletal muscle; Myhz2: myosin, heavy polypeptide 2, fast muscle-specific; myo1b: myozenin 1b; Parv 1: parvalbumin 1; parv 8: parvalbumin 8; pase Mgdep: protein phosphatase, Mg2+/Mn2+-dependent, 1aa; Pdgfa 1b: Pdgfa associated protein 1b; Pdz1: Pdz domain-containing 1; Pgmut: phosphoglycerate mutase; pleca: plectin a; Pr-bindab: purine-rich element-binding protein ab; Prot S100: protein S100; Rab5a: Rab5a protein; RNA-bin b: cold-inducible RNA-binding protein b; Serprot 59: serine protease 59, tandem duplicate 2; Smyhc1: slow myosin heavy chain 1; Tardbpl: Tar DNA-binding protein, -like; thy β: thymosin beta; Timm8b: translocase of inner mitochondrial membrane 8 homolog b; Titb: titin b; TKT: TKT protein; Uap1/1: Udp-n-acetylhexosamine pyrophosphorylase-like protein 1; β-act: beta-actin. | |
TCS was able to modulate 68 different proteins, 45 of which were significantly different (p < 0.05) from controls, but not above the 2-fold change threshold (Fig. S4B†). Then, we found 10 proteins up-regulated and 13 down-regulated (Fig. 6A).
The PNP–TCS complex modified a total of 29 proteins, all above the significance and the biological effect threshold of the 2-fold change (Fig. S4C†). In particular, we measured 11 up-regulated and 18 down-regulated proteins (Fig. 6A).
The entire proteomic data set revealed a number of proteins modulated by PNPs (35) higher than those changed by the PNP–TCS complex (29) and TCS (23), with different biological functions: PNPs mainly modified proteins involved in genetic processes, which represent 25% of the total changed proteins, followed by cytoskeleton (22%), energy (17%) and catalytic activity (11%). Calcium metabolism and transport accounted for 8%, while proteins involved in the immune system were only 3%. Six percent of all the misregulated proteins do not have known functions (Fig. 7A).
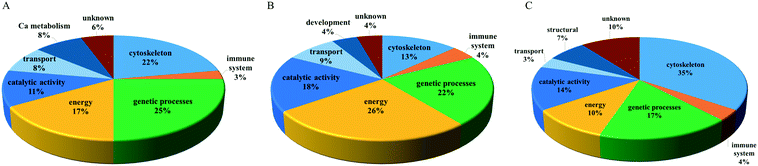 |
| Fig. 7 Molecular functions of modified proteins in zebrafish larvae exposed to (A) PNPs (200 μg L−1), (B) TCS (0.6 μg L−1) and (C) PNP–TCS. | |
More than a quarter of proteins modulated by TCS belongs to the energy group (26%) followed by genetic processes (22%) and catalytic activities (18%), while cytoskeleton dropped to 13% only (Fig. 7B). The remaining 17% included proteins entangled in transport, development and the immune system, while 4% of identified proteins have no known annotated function.
The PNP–TCS complex modulated many proteins unrelated either to PNPs or TCS. The cytoskeleton proteins were the most affected by the complex (35%), while the second most modified group (genetic processes) accounted for 17% of the changed proteins (Fig. 7C). Catalytic activity (14%) and energy (10%) were less represented than in the other treatments, while modifications of structural proteins (7%) appeared.
The Venn diagram (Fig. 6B) confirmed the different effects due to pollutants, since only 7 proteins were in common among the three treatments (Table S2†), highlighting the lack of similar effects on common biological pathways. This is expected for the physical and chemical pollutants, with different routes of intake, accumulation and mechanism of actions (MoA), as confirmed by the only 3 proteins in common between these treatments (Fig. 6B). The MoA of the PNP–TCS complex seemed to be more similar to PNPs than TCS, as indicated by the 8 different proteins in common between the two treatments versus the only 4 changed proteins in common between TCS and the PNP–TCS complex (Fig. 6B).
4. Discussion
The aim of this study is a novelty in the NP scenario since we investigated both the role played by NPs as a vector for TCS and the possible ecotoxicological consequences of the intake of the PNP–TCS complex in relation to the administration of the two single pollutants.
4.1 TCS adsorption and microscopy observations
The first step to certify the following ecotoxicological results was the confirmation of the adsorption of TCS on PNPs and the intake of PNPs and the complex in the biological model.
The high amount of TCS adsorbed on PNPs, corresponding to 36% of the starting TCS concentration added in the suspension, confirmed the high sorption capability of PS for hydrophobic contaminants. This is due to the presence of several benzene rings in its polymeric structure, which increase the distance between the chains, thus facilitating contaminant attachment and integration.25 On the other hand, the sorption behavior of TCS for PS was well described by Li et al.,26 who showed that the proportion of the adsorbed TCS increases with decreasing PS particle size.
The smaller size of NPs not only increases their sorption capability but also enhances their ability to bioaccumulate contaminants inside organism tissues.27 Our study confirmed this behavior since we observed the presence of PNPs in the gastrointestinal tract of zebrafish larvae, yet an external compartment, but also discovered that some PNPs, both pristine and contaminated by TCS, were distributed in other organs, such as the eye (Fig. 2) and the trunk (Fig. 3).
The role played by NPs as a carrier for some environmental contaminants was already noticed in a study performed on adult zebrafish exposed to NPs (1 mg L−1), bisphenol A (BPA; 1 μg L−1) and their mixture, that showed the presence of both contaminants in the viscera, gills, head and muscles, leading also to a higher BPA accumulation in the brain, which was related to a dopaminergic neurotoxic effect.28 Furthermore, an increased bioaccumulation of phenanthrene (0.1 mg L−1) was observed in Daphnia magna when co-exposed to NPs (5 mg L−1) for 14 days, highlighting their action as vectors of PAHs.29
4.2 Effects at organism level
It has been observed that zebrafish larvae, when exposed only to an alternating light/dark period, showed a pattern of increased movement in the dark phase, probably due to decreased predator threat, followed by a resting state in the light phase.30 Thus, the possible alteration of this natural behavior has been used to assess the effects of any substance that might be able to generate neurotoxic effects, such as drugs, metals and nanoparticles.31
We applied this concept to our experiments, in which both TCS and the PNP–TCS complex significantly affected larval swimming activity, as opposed to PNPs administered alone (Fig. 4). Interestingly, we observed that TCS and the PNP–TCS complex had an opposite effect on larval swimming since the total distance moved significantly decreased during both dark and light phases in the larvae exposed to TCS, while it increased for those exposed to the PNP–TCS complex.
The alteration of locomotor behavior of zebrafish larvae caused by the exposure to environmental contaminants has been already observed. For instance, polybrominated diphenyl ethers (PBDEs) and their metabolites caused an altered swimming behavior, showing both an increased and decreased locomotion, depending on the molecules, concentrations and exposure conditions.32,33 The evidence that diverse treatments can differently affect movements was also reported in a study by Ali et al.,34 in which zebrafish embryos were exposed to a wide range of toxic compounds with different MoAs.
In our study, the TCS exposure induced significant larval hypoactivity, which could be attributable to affected locomotor system development,35 while the hyperactivity observed in larvae exposed to the PNP–TCS complex could be attributed to visual defects35 and/or an increased stress/anxiety level.36
Although these hypotheses should be in-depth investigated since the differences in behavioral patterns strictly depend on the neuronal targets of the tested contaminants,37 some results from the proteomic approach seemed to explain the opposite swimming behaviour noticed after the TCS and PNP–TCS complex treatments, as shown below. Moreover, we might suggest that the opposite effect of TCS and the PNP–TCS complex can be attributable to the accumulation of the chemical in different body regions. Indeed, when TCS is administered alone, being a lipophilic compound, it bioaccumulates in lipid tissues, such as the yolk, but when adsorbed on PNPs, it is forced to follow the physical pollutants that accumulate in other tissues and organs, modifying its MoA against different cellular pathways, as previously demonstrated in our study.38 This could not be confirmed in our experiments due to the small size of the zebrafish larvae. Another possible hypothesis could be related to the metabolization of TCS by the CYP 450 detoxification system, mainly present in the liver, producing the more toxic metabolite methyl-TCS.39 Thus, the PNP–TCS complex might modify the accumulation pathway, eliminating or simply decreasing the amount of TCS that ends in the liver and, consequently, reducing the methyl-TCS production and changing its (eco)toxicological effects.
4.3 Effects at sub-organism level
The evaluation of sub-individual effects on exposed organisms was performed to further understand the different toxicity behaviors observed between TCS, PNPs and the PNP–TCS complex. The biomarker approach did not show any significant effect of the PNP treatment, both pristine and contaminated, pointing out only a few effects for TCS administered alone. On the other hand, our results are comparable to those shown in a recent study, in which the evaluation of biomarker responses was applied to test the effects of pristine PE fragments on zebrafish larvae, showing the lack of alteration on both the oxidative stress and the AChE activity.40 However, the reported effects of NPs on aquatic organisms are varied and sometimes controversial, depending on particle concentration, physical and chemical properties, and composition.41 Moreover, our data represent the first evidence concerning the effects of TCS-contaminated NPs on zebrafish, making it difficult to directly compare our results to those from other studies. The only existing studies reported an increased toxicity of NPs when combined with phenanthrene in Daphnia magna,29 and Chen et al.28 pointed out that a mixture of NPs and BPA led to an increase of neurotoxic effects in zebrafish compared to NPs and BPA alone.
Our results did not highlight any effects on AChE activity (Fig. 5), but the measurement of this unique endpoint is not sufficient to identify possible neurotoxic effects in the zebrafish cholinergic system, as also reported by Chen et al.28 Thus, further analyses are needed to exclude the presence of neurological damage which could have been responsible for the locomotion alterations we observed.
Unlike PNPs, the slight and non-homogeneous effects caused by the TCS exposure on zebrafish larvae were only related to a significant (p < 0.05) increase of GST, GPx and micronuclei formation (Fig. 5), confirming the results obtained in our previous study on zebrafish embryos in which we found that environmental concentrations of TCS (0.1–1 μg L−1) were able to induce the activation of detoxification systems and generate cytotoxicity.42 The lack of effects when TCS is bound to PNPs suggested a decrease of the toxicological profile of the complex that can be explained by two different hypotheses: (1) the chemical was not desorbed from PNPs, making it impossible to exploit its effect on the selected endpoints; (2) the complex formation decreased the bioavailability of TCS, reducing its concentration in D. rerio larvae.
As a final remark, the biomarker data set gave another evidence about the modification of the ecotoxicological effects for the 3 different exposures and the extreme complexity of the relationship between physical and chemical pollutants, as well as the need to measure many different endpoints along the biological organization able to obtain a wider ecotoxicological picture.
4.4 Effects on the proteome
The last step of the multi-tier approach was proteomics, a high-throughput technology which complements existing techniques on revealing the MoA of toxic substances. We focused the discussion only on the changed proteins whose functions can be related to results revealed by the other two applied approaches, while the description of the other classes of modulated proteins is shown in the ESI.†
4.4.1 Structural proteins.
Unlike the other two treatments, the PNP–TCS complex treatment selectively modified two specific structural proteins (Fig. 6A), namely the crystallin γ M2d2 (CryM2d2) and collagen type XIV α1b (Col4α1b), confirming the zebrafish eye as a target for NPs, as also pointed out by microscopy (Fig. 2). This evidence is of particular interest as it may be closely related to the hyperactivity of zebrafish larvae observed after the exposure to the PNP–TCS complex (Fig. 4) since visual defects have been identified precisely as one of the possible causes of behavioral changes.35
CryM2d2 is a structural constituent of the vertebrate lens crystallin which is mainly composed by α-, β- and γ-crystallin proteins.43 Although the γM-crystallin class represents more than 70% of the eye lens proteins in zebrafish,44 their functional role is not completely clear. Interestingly, Pande et al.45 reported that γ-crystallins are essential for maintaining lens transparency since their aggregation decreases the lens homogeneity and leads to cataract. Thus, the over-production of CryM2d2 noticed after the PNP–TCS complex exposure could be a signal of eye defects due to an increased crystallization of the γ-crystallins, whose deposits in the lens are typical in several non-genetic forms of cataract.46
A different function is related to Col4α1b, the other structural protein modulated only by the PNP–TCS complex, whose change can be related to eye function. Col4α1b belongs to the superfamily of fibril-associated collagens with interrupted triple helices (FACIT)47 which is present in some connective tissues, such as the skin, tendons, and cornea.48 Its main function is the regulation of the formation and size of the collagens fibrils to guarantee the stability and integrity of the extracellular matrix and its fibrillar collagen network.49 Young et al.50 found that type XIV collagen is highly expressed in the early stage of development of chicken embryos and it is involved in the regulation of fibrillogenesis in the corneal stroma.
The clear evidence that the two structural proteins modulated exclusively by the PNP–TCS complex are directly related to eye development and that they can be the possible reason for the hyperactivity noticed pointed out the need of future in-depth studies on the effect exerted by plastics and their adsorbed environmental pollutants in the early stages of eye development. This represents another proof about the change of the ecotoxicological behaviour when the two pollutants were administered bound together and probably due to the capability of PNPs to have the eye as a carrier, but at the same time to exert these specific effects only when in combination with TCS. On the other hand, previous studies identified the eye as one of the preferential targets for NPs: van Pomeren et al.51 demonstrated the deposition of 25 and 50 nm PNPs in the zebrafish eye and Lee et al.52 observed the accumulation of 50 nm PNPs in the retina of zebrafish embryos at 24 hours post-fertilization (hpf).
4.4.2 Proteins of energetic pathways.
This was the biggest group of proteins modulated by TCS (Fig. 7), suggesting a direct effect of the chemical on the energy stock and energetic pathways able to determine the hypo-activity observed after the behavioral tests (Fig. 4).
The KEGG (Kyoto Encyclopedia of Genes and Genomes) PATHWAY database highlighted that 4 proteins (fructose-bisphosphate aldolase C-A, aldoca; glycogenin 1b, Gyg1b; glutaryl-CoA dehydrogenase, Gcdhl; ATPase H+-transporting V1, Atp6v1ab) out of 6 modulated by TCS were involved in some energetic pathways. In detail, aldoca is directly involved in one of the essential sub-pathways of glycolysis that synthesizes D-glyceraldehyde 3-phosphate and glycerone phosphate from D-glucose. Since several previous studies on fish species53–56 showed an up-regulation of aldoca during conditions reflecting a strong energy demand, the increased production of this glycolytic enzyme noticed in our experiments suggested the need of an energy over-production for zebrafish larvae through the formation of glycolytic ATP molecules, probably to counteract the effects due to TCS. One of the possible effects of TCS could thus be related to a rise of energy demand and the subsequent decrease in the energy stock available to other activities. Therefore, the hypo-activity revealed after the TCS exposure (Fig. 4) can be an indirect consequence of this energy lowering. This hypothesis seemed to be also confirmed by the downregulation of Gyg1b (Fig. 6A) which is involved in glycogen biosynthesis, fundamental as an energetic reserve. Unfortunately, no previous studies are available for Gyg in Danio rerio but, from the ecotoxicological point of view, since the skeletal muscles use glycogen as the main source of energy for anaerobic metabolism to fuel short and intense activity, the downregulation of Gyg1b suggested once more a deficit in the energy storage at muscular level that could explain the movement decrease observed during the behavioral assays.
The functions of the other two proteins belonging to the energy group (Gcdhl and Atp6v1ab) that were downregulated by TCS also supported this hypothesis. Indeed, Gcdhl is a mitochondrial protein involved in the pathway of fatty acid degradation that catalyzes the transformation of glutaryl-CoA into crotonyl-CoA.57 This is one of the final steps to produce acetyl-CoA, the entry molecule for the citric acid cycle that represents the main energy supply for animals. In humans, GCDH deficiency creates a neurodegenerative disease (glutaric aciduria type 1) characterized by an irreversible dystonic–dyskinetic movement disorder due to striatal neuronal death.58
Atp6v1ab, previously known as Atp6v1a, is indirectly related to glycolysis and pentose phosphate pathways involved in the ATP metabolic process and hydrogen ion transmembrane transport. Besides representing a proton transporter for all the steps of the energy pathways, this protein is also involved in the crucial role of ammonia excretion in zebrafish larvae. Indeed, Shih et al.59 demonstrated that the H+-ATPase of the apical membrane of the pump-rich cells (HRCs) of the Danio rerio gills contribute to more than 70% of skin acid secretion and that the knockdown of the H+-pump gene Atp6v1a significantly decreased the NH4+ secretion.
4.4.3 Cytoskeleton proteins.
Five out of the 8 modulated proteins in common between PNPs and the PNP–TCS complex belong to the cytoskeleton (myosin heavy polypeptide 1, skeleton muscle, Myhz1.1; myosin heavy polypeptide 2, fast muscle-specific, Myhz2; myosin heavy chain b, Myhb, slow myosin heavy chain 1, Smyhc1; plectin a, Pleca). These 5 up-regulated proteins are mainly related to the skeletal muscle system development and organization in zebrafish60–63 (Table S2†). Very interestingly, the KEGG PATHWAY database revealed that three of them (Myhz2, Myhb and Smyhc1) are also functional elements of the paracellular tight junctions (TJs) of skeletal muscles that connect the absorptive epithelial cells at the apical membrane in the gastrointestinal tract.64 TJs are multiprotein complexes whose main function is to assemble a paracellular barrier, allowing a selective diffusion on the basis of size and charge of ions and molecules.65 According to most recent data, ions and molecules are hypothesized to permeate through the TJs by two different routes: pore and leak pathways.66,67 The first one is the preferential pathway for molecules smaller than 0.4 nm,68 while the leak pathway is a nonspecific way of entrance for macromolecules with a diameter up to 6 nm.69 Although the PNPs administered to zebrafish in our study, with a size of 0.5 μm, should not enter through the TJs, they modified the amount of three of the structural proteins of this paracellular barrier which regulate actin in order to control the cell polarity. Thus, the same co-regulation noticed both for PNPs and the PNP–TCS complex might point out a possible alteration in the intercellular defence mechanism related just to PNPs.
At a more general level of discussion, the great impact on cytoskeleton proteins highlighted for PNPs and the PNP–TCS complex confirmed that the increase of oxidative stress is the main effect of these physical contaminants. Indeed, several studies have shown that the proteins of the cytoskeleton are one of the first targets of oxidative stress,70–72 as also found in our previous study in which we administered two different mixtures of MPs to the freshwater bivalve D. polymorpha.8
4.4.4 Final remarks for proteomics.
Proteomics has proven to be a very useful and sensitive tool in the evaluation of ecotoxicity due to NPs, whose infiltration in the organism's tissues follows different pathways than chemicals, passing through the biological barriers and/or exploiting the transmembrane canals and TJs, depending on their size. The logical consequence is that the number of NPs that enters the organism tends to be lower than the number of chemicals which simply follow a concentration gradient, constraining the use of very sensitive analytical methodologies to observe the presence of NPs in the tissues and also to evaluate their toxicological effects. The high-throughput technology based on proteomics is surely one of the possible solutions because of its sensitivity that allows the evaluation of the impact made on different cellular pathways and suggests possible MoAs, as demonstrated in this study. Indeed, proteomics confirmed that the combined effect of chemical and physical pollutants should be considered more as a modification of toxicological behavior rather than a mere increase or decrease in toxicity, since the PNP–TCS complex not only modulated both proteins in common with TCS and PNPs but also changed several other proteins not modulated by the two contaminants administered alone. The most evident case is the one related to the two structural proteins up-regulated by the PNP–TCS complex alone, thus suggesting a complementary effect on the early stages of eye development only when the two contaminants were bound together.
Fig. 8 summarizes the network of proteins related to the same biological function and the relative effect of the three different contaminants. This toxicological behavior explains exactly what was said previously, namely that the PNP–TCS complex intrinsically possesses the ability to act both on protein targets modulated by PNP and TCS alone and to acquire new toxicological characteristics.
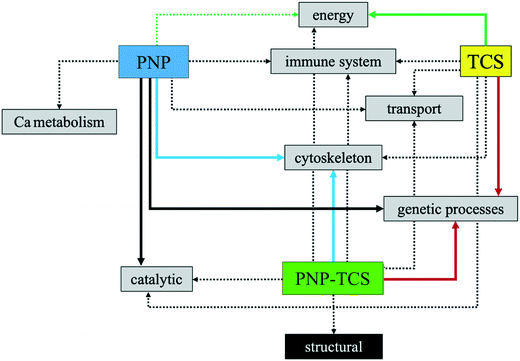 |
| Fig. 8 Outline of proteomic results: dotted lines = low to medium effect of the contaminant; solid lines = high effect of the contaminant; same colour = similar effect of contaminants (high number of co-modulated proteins). | |
5. Conclusions
The purpose of this study was to open a new perspective on investigating the risk associated to the action of NPs as carriers of environmental pollutants. Overall, the whole data set obtained with the multi-step approach made a clear response to the main goal of this study, since it highlighted that the formation of the PNP–TCS complex heavily modified the toxicological behaviour of the single contaminants. It almost seems that the bond between chemical and physical compounds creates a kind of new pollutant with emerging and unpredictable characteristics that are able to change the toxicological pathways and targets. From the ecotoxicological point of view, our multiple results highlighted how the PNP–TCS complex showed a change in toxicological behavior with respect to individual contaminants instead of a simple decrease or increase of toxicity, suggesting the need to go beyond classical models based on additive, synergistic or antagonist effects that regulate interactions between chemical pollutants.
Another crucial result obtained is related to the need for a multilevel approach that covers many steps of biological organization. Indeed, differences found in the responses clearly showed that its application is critical, especially in this kind of studies, in which the relation between chemical and physical compounds is unknown and leads to unpredictable effects.
Ethical statement
All animal procedures were performed in accordance with the Guidelines for Care and Use of Laboratory Animals of the University of Milan and approved by the Animal Ethics Committee of the Italian Ministry of Health (Aut. Min. no. 6/2019-PR).
Conflicts of interest
There are no conflicts to declare.
References
- R. Geyer, J. R. Jambeck and K. L. Law, Production, use, and fate of all plastics ever made, Sci. Adv., 2017, 3, e1700782 CrossRef.
- T. O'Brine and R. C. Thompson, Degradation of plastic carrier bags in the marine environment, Mar. Pollut. Bull., 2010, 60, 2279–2283 CrossRef.
- N. B. Hartmann, T. Huffer, R. C. Thompson, M. Hasselov, A. Verschoor, A. E. Daugaard, S. Rist, T. Karlsson, N. Brennholt, M. Cole, M. P. Herrling, M. C. Hess, N. P. Ivleva, A. L. Lusher and M. Wagner, Are we speaking the same language? Recommendations for a definition and categorization framework for plastic debris, Environ. Sci. Technol., 2019, 53, 1039–1047 CrossRef CAS.
- K.-W. Lee, W. J. Shim, O. Y. Kwon and J.-H. Kang, Size-dependent effects of micro polystyrene particles in the marin copepod Tigriopus japonicus, Environ. Sci. Technol., 2013, 47, 11278–11283 CrossRef CAS.
- Y. Lu, Y. Zhang, Y. Deng, W. Jiang, Y. Zhao, J. Geng, L. Ding and H. Ren, Uptake and accumulation of polystyrene microplastics in zebrafish (Danio rerio) and toxic effects in liver, Environ. Sci. Technol., 2016, 50, 4054 CrossRef CAS.
- L. Lei, S. Wu, S. Lu, M. Liu, Y. Song, Z. Fu, H. Shi, K. M. Raley-Susman and D. He, Microplastic particles cause intestinal damage and other adverse effects in zebrafish Danio rerio and nematode Caenorhabditis elegans, Sci. Total Environ., 2018, 619–620, 1–8 CrossRef CAS.
- S. Magni, F. Gagné, C. André, C. Della Torre, J. Auclair, H. Hanana, C. C. Parenti, F. Bonasoro and A. Binelli, Evaluation of uptake and chronic toxicity of virgin polystyrene microbeads in freshwater zebra mussel Dreissena polymorpha (Mollusca: Bivalvia), Sci. Total Environ., 2018, 631–632, 778–788 CrossRef CAS.
- S. Magni, C. Della Torre, G. Garrone, A. D'Amato, C. C. Parenti and A. Binelli, First evidence of protein modulation by polystyrene microplastics in a freshwater biological model, Environ. Pollut., 2019, 250, 407–415 CrossRef CAS.
- C. C. Parenti, A. Ghilardi, C. Della Torre, S. Magni, L. Del Giacco and A. Binelli, Evaluation of the infiltration of polystyrene nanobeads in zebrafish embryo tissues after short-term exposure and the related biochemical and behavioural effects, Environ. Pollut., 2019, 254, 112947 CrossRef CAS.
- M. S. S. Silva, M. Oliveira, D. Lopéz, M. Martins, E. Figueira and A. Pires, Do nanoplastics impact the ability of the polychaeta Hediste diversicolor to regenerate?, Ecol. Indic., 2020, 110, 105921 CrossRef CAS.
- X. Liu, J. Xu, Y. Zhao, H. Shi and C. H. Huang, Hydrophobic sorption behaviors of 17β-Estradiol on environmental microplastics, Chem, 2019, 226, 726–735 CAS.
- K. N. Fotopoulou and H. K. Karapanagioti, Surface properties of beached plastic pellets, Mar. Environ. Res., 2012, 81, 70–77 CrossRef CAS.
- F. Yu, C. Yang, Z. Zhu, X. Bai and J. Ma, Adsorption behavior of organic pollutants and metals on micro/nanoplastics in the aquatic environment, Sci. Total Environ., 2019, 694, 133643 CrossRef CAS.
- F. Wang, K. M. Shih and X. Y. Li, The partition behavior of perfluoro-octanesulfonate (PFOS) and perfluoro-octanesulfonamide (PFOA) on microplastics, Chem, 2015, 119, 841–847 CAS.
- X. C. Shen, D. C. Li, X. F. Sima, H. Y. Cheng and H. Jiang, The effects of environmental conditions on the enrichment of antibiotics on microplastics in simulated natural water column, Environ. Res., 2018, 166, 377–383 CrossRef CAS.
- J. Li, K. Zhang and H. Zhang, Adsorption of antibiotics on microplastics, Environ. Pollut., 2018, 237, 460–467 CrossRef CAS.
- B. Nowack and T. D. Bucheli, Occurrence, behavior and effects of nanoparticles in the environment, Environ. Pollut., 2007, 150, 5–22 CrossRef CAS.
- S. Jagini, S. Konda, D. Bhagawan and V. Himabindu, Emerging contaminant (triclosan) identification and its treatment: A review, SN Appl. Sci., 2019, 1, 640 CrossRef.
- L. M. Weatherly and J. A. Gosse, Triclosan exposure, transformation, and human health effects, J. Toxicol. Environ. Health, Part B, 2017, 20(8), 447–469 CAS.
- C. Wu, K. Zhang, X. Huang and J. Liu, Sorption of pharmaceuticals and personal care products to polyethylene debris, Environ. Sci. Pollut. Res., 2016, 23, 8819–8826 CrossRef CAS.
- K. Syberg, A. Nielsen, F. R. Khan, G. T. Banta, A. Palmqvist and P. M. Jepsen, Microplastic potentiates triclosan toxicity to the marine copepod Acartia tonsa (Dana), J. Toxicol. Environ. Health, Part A, 2017, 80(23–24), 1369–1371 CrossRef CAS.
- R. R. Tice, E. Agurell, D. Anderson, B. Burlinson, A. Hartmann, H. Kobayashi, Y. Miyamae, E. Rojas and Y. Sasaki, Single cell gel/Comet assay: guidelines for in-vitro and in-vivo genetic toxicology testing, Environ. Mol. Mutagen., 2000, 35, 206–221 CrossRef CAS.
- M. Parolini, A. Ghilardi, C. Della Torre, S. Magni, L. Prosperi, M. Calvagno, L. Del Giacco and A. Binelli, Environmental concentrations of cocaine and its main metabolites modulated antioxidant response and caused cyto-genotoxic effects in zebrafish embryo cells, Environ. Pollut., 2017, 226, 504–514 CrossRef CAS.
- M. M. Bradford, A rapid and sensitive method for the quantification of microgram quantities of protein using the principle of protein-dye binding, Anal. Biochem., 1976, 72, 248–254 CrossRef CAS.
- O. S. Alimi, J. F. Budarz, L. M. Hernandez and N. Tufenkji, Microplastics and nanoplastics in aquatic environments: Aggregation, deposition, and enhanced contaminant transport, Environ. Sci. Technol., 2018, 52, 1704–1724 CrossRef CAS.
- Y. Li, M. Li, Z. Li, L. Yang and X. Liu, Effects of particle size and solution chemistry on triclosan sorption on polystyrene microplastic, Chemosphere, 2019, 213, 308–314 CrossRef.
- J. P. da Costa, P. S. M. Santos, A. C. Duarte and T. Rocha-Santos, (Nano)plastics in the environment - Sources, fates and effects, Sci. Total Environ., 2016, 566–567, 15–26 CrossRef.
- Q. Chen, D. Yin, Y. Jia, S. Schiwy, J. Legradi, S. Yang and H. Hollert, Enhanced uptake of BPA in the presence of nanoplastics can lead to neurotoxic effects in adult zebrafish, Sci. Total Environ., 2017, 609, 1312–1321 CrossRef CAS.
- Y. Ma, A. Huang, S. Cao, F. Sun, L. Wang, H. Guo and R. Ji, Effects on nanoplastics and microplastics on toxicity, bioaccumulation, and environmental fate of phenanthrene in fresh water, Environ. Pollut., 2016, 219, 166–173 CrossRef CAS.
- R. C. MacPhail, J. Brooks, D. L. Hunter, B. Padnos, T. D. Irons and S. Padilla, Locomotion in larval zebrafish: influence of time of day, lighting and ethanol, Neurotoxicology, 2009, 30, 52–58 CrossRef CAS.
- R. M. Basnet, D. Zizioli, S. Taweedet, D. Finazzi and M. Memo, Zebrafish larvae as behavioural model in neuropharmacology, Biomedicines, 2019, 7, 23 CrossRef.
- L. J. Macaulay, J. M. Bailey, E. D. Levin and H. M. Stapleton, Persisting effects of a pbde metabolite, 6-oh-bde-47, on larval and juvenile zebrafish swimming behavior, Neurotoxicol. Teratol., 2015, 52, 119–126 CrossRef CAS.
- C. Y. Usenko, E. M. Robinson, S. Usenko, B. W. Brooks and E. D. Bruce, PBDE developmental effects on embryonic zebrafish, Environ. Toxicol. Chem., 2011, 30(8), 1865–1872 CrossRef CAS.
- S. Ali, D. L. Champagne and M. K. Richardson, Behavioral profiling of zebrafish embryos exposed to a panel of 60 water-soluble compounds, Behav. Brain Res., 2012, 228, 272–283 CrossRef CAS.
- S. Ali, D. L. Champagne, A. Alia and M. K. Richardson, Large-scale analysis of acute ethanol exposure in zebrafish development: a critical time window and resilience, PLoS One, 2011, 6(5), e20037 CrossRef CAS.
- T. D. Irons, P. E. Kelly, D. L. Hunter, R. C. Macphail and S. Padilla, Acute administration of dopaminergic drugs has differential effects on locomotion in larval zebrafish, Pharmacol., Biochem. Behav., 2013, 103, 792–813 CrossRef CAS.
- L. D. Ellis, J. Seibert and K. H. Soanes, Distinct models of induced hyperactivity in zebrafish larvae, Brain Res., 2012, 1449, 46–59 CrossRef CAS.
- A. Binelli, L. Del Giacco, N. Santo, L. Bini, S. Magni, M. Parolini, L. Madaschi, A. Ghilardi, D. Maggioni, M. Ascagni, A. Armini, L. Prosperi, L. Landi, C. La Porta and C. Della Torre, Carbon nanopowder acts as a Trojan-horse for benzo(α)pyrene in Danio rerio embryos, Nanotoxicology, 2017, 11(3), 371–381 CrossRef CAS.
- Y. Peng, Y. Luo, X. P. Nie, W. Liao, Y. F. Yang and G. G. Ying, Toxic effects of triclosan on the detoxification system and breeding of Daphnia magna, Ecotoxicology, 2013, 22, 1384–1394 CrossRef CAS.
- A. Karami, B. D. Groman, S. P. Wilson, P. Ismail and V. K. Neela, Biomarker responses in zebrafish (Danio rerio) larvae exposed to pristine low-density polyethylene fragments, Environ. Pollut., 2017, 223, 466–475 CrossRef CAS.
- A. Haegerbaeumer, M.-T. Mueller, H. Fueser and W. Traunspurger, Impacts of micro- and nano-sized plastic particles on benthic invertebrates: A literature review and gap analysis, Front. Environ. Sci., 2019, 7, 17 CrossRef.
- C. C. Parenti, A. Ghilardi, C. Della Torre, M. Mandelli, S. Magni, L. Del Giacco and A. Binelli, Environmental concentrations of triclosan activate cellular defence mechanism and generate cytotoxicity on zebrafish (Danio rerio) embryos, Sci. Total Environ., 2019, 650, 1752–1758 CrossRef CAS.
- H. Bloemendal, W. De Jong, R. Jaenicke, N. H. Lubsen, C. Slingsby and A. Tardieu, Ageing and vision: Structure, stability and function of lens crystallins, Prog. Biophys. Mol. Biol., 2004, 86, 407–485 CrossRef CAS.
- Y. R. Lin, H. K. Mok, Y. H. Wu, S. S. Liang, C. C. Hsiao, C. H. Huang and S. H. Chiou, Comparative proteomics analysis of degenerative eye lenses of nocturnal rice eel and catfish as compared to diurnal zebrafish, Mol. Vision, 2013, 19, 623–637 CAS.
- A. Pande, J. Pande, N. Asherie, A. Lomakin, O. Ogun, J. King and G. B. Benedek, Crystal cataracts: Human genetic cataract caused by protein crystallization, Proc. Natl. Acad. Sci. U. S. A., 2001, 98(11), 6116–6120 CrossRef CAS.
- A. Mumford, I. Cree, J. Arnold, M. Hagan, K. Rixon and J. Harding, The lens in hereditary hyperferritinaemia cataract syndrome contains crystalline deposits of L-ferritin, Br. J. Ophthalmol., 2000, 84, 697–700 CrossRef CAS.
- H. L. Bader, E. Lambert, A. Guiraud, M. Malbouyres, W. Driever, M. Koch and F. Ruggiero, Zebrafish collagen XIV is transiently expressed in epithelia and is required for proper function of certain basement membranes, J. Biol. Chem., 2013, 288, 6777–6787 CrossRef CAS.
-
T. Manon-Jensen and M. A. Karsdal, in Biochemistry of collagens, laminins and elastin: Structure, function and biomarkers, ed. M. A. Karsdal, ScienceDirect, 2016, vol. 272 Search PubMed.
- J.-B. Oudart, J.-C. Monboisse and F. X. Maquart, Type XIX collagen: A new partner in the interactions between tumor cells and their microenvironment, Matrix Biol., 2017, 57–58, 169–177 CrossRef CAS.
- B. B. Young, G. Zhang, M. Koch and D. E. Birk, The roles of types XII and XIV collagen in fibrillogenesis and matrix assembly in the developing cornea, J. Cell. Biochem., 2002, 87, 208–220 CrossRef CAS.
- M. van Pomeren, N. R. Brun, W. J. G. M. Peijnenburg and M. G. Vijver, Exploring uptake and biodistribution of polystyrene (nano)particles in zebrafish embryos at different developmental stages, Aquat. Toxicol., 2017, 190, 40–45 CrossRef CAS.
- W. S. Lee, H.-J. Cho, E. Kim, J. H. Hu, H.-J. Kim, B. Kim, J.-S. Lee and J. Jeong, Bioaccumulation of polystyrene nanoplastics and their effect on the toxicity of Au ions in zebrafish embryos, Nanoscale, 2019, 11, 3173 RSC.
- S. A. M. Martin, O. Vilhelmsson, F. Médale, P. Watt, S. Kaushik and D. F. Houlihan, Proteomic sensitivity to dietary manipulations in rainbow trout, Biochim. Biophys. Acta, 2003, 1651, 17–29 CrossRef CAS.
- O. T. Vilhelmsson, S. A. M. Martin, F. Mèdale, S. J. Kaushik and D. F. Houli-han, Dietary plant-protein substitution affects hepatic metabolism in rainbow trout (Oncorhynchus mykiss), Br. J. Nutr., 2004, 92, 71–80 CrossRef CAS.
- R. W. Smith, P. Cash, S. Ellefsen and G. E. Nilsson, Proteomic changes in the crucian carp brain during exposure to anoxia, Proteomics, 2009, 9, 2217–2229 CrossRef CAS.
- P. Gómez-Requeni, L. E. C. Conceição, A.-E. O. Jordal and I. Rønnestad, A reference growth curve for nutritional experiments in zebrafish (Danio rerio) and changes in whole body proteome during development, Fish Physiol. Biochem., 2010, 36, 1199–1215 CrossRef.
- K. S. Rao, M. Albro, T. M. Dwyer and F. E. Frerman, Kinetic Mechanism of Glutaryl-CoA Dehydrogenase, Biochemistry, 2006, 45–51, 15853–15861 CrossRef.
- J. Gao, C. Zhang and X. Luo, Effects of targeted suppression of glutaryl-CoA dehydrogenase by lentivirus-mediated shRNA and excessive intake of lysine on apoptosis in rat striatal neurons, PLoS One, 2013, 8(5), e63084 CrossRef CAS.
- T.-H. Shih, J.-L. Horng, P.-P. Hwang and L.-Y. Lin, Ammonia excretion by the skin of zebrafish (Danio rerio) larvae, Am. J. Physiol., 2008, 295, 1625–1632 CrossRef.
- M. Codina, J. Li, J. Gutiérrez, J. P. Y. Kao and S. J. Du, Loss of Smyhc1 or Hsp90alpha1 function results in different effects on myofibril organization in skeletal muscles of zebrafish embryos, PLoS One, 2010, 5, e8416 CrossRef.
- H. Nord, A. C. Burguiere, J. Muck, C. Nord, U. Ahlgren and J. von Hofsten, Differential regulation of myosin heavy chains defines new muscle domains in zebrafish, Mol. Biol. Cell, 2014, 25, 1384–1395 CrossRef.
- J. B. Buhrdel, S. Hirth, M. Kessler, S. Westphal, M. Forster, L. Manta, G. Wiche, B. Schoser, J. Schessl, R. Schroder, C. S. Clemen, L. Eichinger, D. O. Furst, P. F. van der Ven, W. Rottbauer and S. Just, In vivo characterization of human myofibrillar myopathy genes in zebrafish, Biochem. Biophys. Res. Commun., 2015, 461, 217–223 CrossRef.
- K. Henke, J. M. Daane, M. B. Hawkins, C. M. Dooley, E. M. Busch-Nentwich, D. L. Stemple and M. P. Harris, Genetic screen for postembryonic
development in the zebrafish (Danio rerio): Dominant mutations affecting adult form, Genetics, 2017, 207, 609–623 CAS.
- G. As
onaite, H. Sundh, N. Asker and B. C. Almroth, Rainbow trout maintain intestinal transport and barrier functions following exposure to polystyrene microplastics, Environ. Sci. Technol., 2018, 52, 14392–14401 CrossRef.
-
J. Hou, in The Paracellular Channel: Biology, Physiology and Disease, Elsevier, 2019, vol. 248 Search PubMed.
- C. T. Capaldo and A. Nusrat, Claudin switching: Physiological plasticity of the tight junction, Semin. Cell Dev. Biol., 2015, 42, 22–29 CrossRef CAS.
- A. Tervonen, T. O. Ihalainen, S. Nymark and J. Hyttinen, Structural dynamics of tight junctions modulate the properties of the epithelial barrier, PLoS One, 2019, 0214876 Search PubMed.
- C. M. Van Itallie, J. Holmes, A. Bridges and J. M. Anderson, Claudin-2-dependent changes in noncharged solute flux are mediated by the extracellular domains and require attachment to the PDZ-scaffold, Ann. N. Y. Acad. Sci., 2009, 1165, 82–87 CrossRef CAS.
- M. M. Buschmann, L. Shen, H. Rajapakse, D. R. Raleigh, Y. Wang, Y. Wang, A. Lingaraju, J. Zha, E. Abbott, E. M. McAuley, L. A. Breskin, L. Wu, K. Andreson, J. R. Turner and C. R. Weber, Occludin OCEL-domain interactions are required for maintenance and regulation of the tight junction barrier to macromolecular flux, Mol. Biol. Cell, 2013, 24, 3056–3068 CrossRef CAS.
- C. Riva, S. Cristoni and A. Binelli, Effects of triclosan in the freshwater Dreissena polymorpha: A proteomic investigation, Aquat. Toxicol., 2012, 118–119, 62–71 CrossRef CAS.
- C. Wilson and C. Gonzalez-Billault, Regulation of cytoskeletal dynamics by redox signaling and oxidative stress: Implications for neuronal development and trafficking, Front. Cell. Neurosci., 2015, 9, 381 Search PubMed.
- E. Belcastro, W. Wu, I. Fries-Raeth, A. Corti, A. Pompella, P. Leroy, I. Larteaud and C. Gaucher, Oxidative stress enhances and modulates protein S-nitrosation in smooth muscle cells exposed to S-nitrosoglutathione, Nitric Oxide, 2017, 69, 10–21 CrossRef CAS.
Footnote |
† Electronic supplementary information (ESI) available. See DOI: 10.1039/d0en00961j |
|
This journal is © The Royal Society of Chemistry 2021 |