Cobalt ferrite nanozyme for efficient symbiotic nitrogen fixation via regulating reactive oxygen metabolism†
Received
9th September 2020
, Accepted 19th November 2020
First published on 21st November 2020
Abstract
Biological nitrogen fixation is essential to crop production, but it can be inhibited by high concentrations of reactive oxygen species (ROS) due to the irreversible inactivation of the nitrogenase complex, thus posing a threat to food security and sustainable agricultural development. Here, we constructed an antioxidant cobalt ferrite (CoFe2O4) nanozyme as a bridge between nanotechnology and biological nitrogen fixation, which was shown to efficiently regulate the reactive oxygen metabolism and protect nitrogenase, thereby significantly enhancing the symbiotic nitrogen fixation efficiency by 260% in Glycine max (L.) Merr. (soybean). The CoFe2O4 nanozyme was also revealed to effectively reduce the concentration of ROS in the nodule by 56.6%, creating a superior environment for the proliferation of rhizobia and forming more effective nodules (larger nodules for an increase of 45.6% in the number of parasitic rhizobia). Furthermore, the CoFe2O4 nanozyme was shown to act as a synergist of leghemoglobin and increase its accumulation by 45.9%, where the high concentration of leghemoglobin in nodular cells can create a relatively hypoxic environment and protect nitrogenase, thus achieving a quantitative leap in nitrogen fixation capacity and simultaneously increasing the soybean photosynthesis by 67.2%. Our study has demonstrated that the CoFe2O4 nanozyme can efficiently regulate the intracellular ROS metabolism and serve as a promising strategy for enhancing symbiotic nitrogen fixation.
Environmental significance
As environmental pollution continues to intensify, soil crops are facing severe reactive oxygen stress. For nitrogen-fixing microorganisms, high concentrations of reactive oxygen species (ROS) irreversibly inactivate the nitrogenase complex and inhibit biological nitrogen fixation. Therefore, we use the excellent peroxidase-like activity of cobalt ferrite nanoparticles (CoFe2O4-NPs) to study their contribution to the improvement in a soybean symbiotic nitrogen fixation system. The results show that CoFe2O4-NPs can effectively enhance the nitrogenase activity of Glycine max (L.) Merr. (soybean) nodules and promote nodular development. Meanwhile, the accumulation level of leghemoglobin also increased significantly. This study has shed light on both the development of effective nano-agrochemicals and the synergistic measures of nano-agrochemicals in symbiotic nitrogen fixation.
|
Introduction
Nitrogen (N2) fixation by converting atmospheric nitrogen to ammonia (NH3) is considered as one of the most significant processes in the chemical industry, because NH3 plays a key role not only in fertilizer production and supporting a growing global population, but also as a carrier of green energy.1–4 Currently, industrial ammonia is synthesized mainly through the traditional Haber–Bosch process, and the iron-based catalyst is used at a high pressure (150–200 atm) and temperature (300–500 °C), which causes pollution and greenhouse gas emissions,5–7 suggesting the necessity of seeking a green, sustainable, and alternative technology for producing NH3 under milder conditions.
The integration of nanotechnology with biology can be an important driver for more sustainable and efficient agricultural systems.8 In nature, nitrogen fixation is catalysed by nitrogenase, an enzyme complex containing an iron–molybdenum cofactor (FeMo).9 It is estimated that about 90 million tons of nitrogen can be reduced (or repaired) by biological nitrogen fixation technology each year, which may support about half of the global population and provide a suitable blueprint for the future development of alternative nitrogen fixation methods.10,11 Although it works at room temperature (25 °C) and pressure (1 atm), nitrogenase requires a large amount of chemical energy input, and in the catalytic process, some electrons may escape from the electron transport chain, resulting in partially reduced oxygen intermediates (often called reactive oxygen species).12
It is well known that nitrogenase is very sensitive to oxygen and ROS,10 and ROS prevention plays an important role in the rhizobia–legume symbiosis,13 indicating that maintaining the activity of nitrogenase is a delicate balance in plant nodules. In the nitrogen reduction process, a higher respiration rate is required to meet the energy demand, but the nitrogenase complex is irreversibly inactivated by oxygen and ROS.14 As the permeability to oxygen is greatly limited by a diffusion barrier of the nodule cortex, the necessary oxygen is delivered by the plant oxygen carrier leghemoglobin present only in nodules. Although a low concentration of free oxygen can be ensured by this strategy, a high respiration rate inevitably leads to a large amount of ROS in nodules.15 Therefore, effective anti-oxidation measures are essential for efficient nitrogen fixation and delaying aging. Previous studies have shown that bacterial superoxide dismutase (SOD) plays a crucial role in protecting the nitrogen fixation process from oxidation. In bacteroids, enzymes in the nitrogenase complex contain oxidizable [4Fe–4S] clusters, which are potential targets for superoxidase, although their direct effect has not been demonstrated.13
In biological organisms, ROS, such as hydrogen peroxide (H2O2), OH˙, and O2˙−, are the by-products of oxygen metabolism.16 An appropriate amount of ROS can serve as the key secondary messenger for cellular signal transduction, pathogen defence, and homeostasis.17 However, a high ROS level can cause lipid peroxidation, protein denaturation, or DNA damage,18 which can adversely affect cells. Thus, maintaining the redox equilibrium against oxidative stress is key to maintaining cellular homeostasis, and artificial enzymes are needed to provide cytoprotection against various ROS-mediated diseases. Recently, many research efforts have been devoted to the development of highly effective antioxidant enzymes.19 Among them, nanozymes, as an emerging field bridging nanotechnology and biology, show the potential to substitute for traditional enzyme mimics and natural enzymes in practical applications, due to their versatility, high stability and easy batch preparation.20,21 Nanoparticles (NPs) can effectively scavenge ROS through a variety of enzyme-like activities, including SOD-, catalase (CAT)-, or peroxidase (POD)-like activities. Fan et al. reported that Fe3O4-NPs possess a CAT-like activity, which can efficiently reduce intracellular ROS stress, delay aging, and ameliorate neurodegeneration in a Drosophila model.20,22 Similarly, due to their excellent SOD- and CAT-mimic capacities, CeO2 (ref. 23) and Mn3O4 (ref. 24) NPs were shown to effectively inhibit ROS-induced cell damage. In addition, Mugesh et al. found that V2O5-NPs had a GPx-mimic capacity for cytoprotection.25 These reports indicate that nanoenzymes (nanozymes) can serve as a new strategy for scavenging ROS during nitrogen fixation, thus improving nitrogenase activity and nitrogen fixation efficiency.
Herein, we constructed a cobalt ferrite (CoFe2O4) nanozyme with antioxidant enzyme activity to efficiently promote nitrogen fixation in the Glycine max (L.) Merr. (soybean)–rhizobial symbiotic system (Scheme 1). Owing to the double oxidation states of Co2+ and Co3+, the CoFe2O4 nanozyme possessed remarkable peroxide/SOD mimicking activities, which could effectively reduce the concentration of active oxygen in the nodules and create a superior environment for the proliferation of rhizobia, thus promoting rhizobial colonization and protecting the function of nitrogenase. Furthermore, the CoFe2O4 nanozyme could act as a synergist of leghemoglobin and promote its accumulation, further enhancing the antioxidant activity of nodules and the efficiency of symbiotic nitrogen fixation.
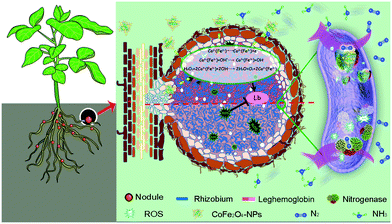 |
| Scheme 1 Proposed mechanisms of the CoFe2O4 nanozyme in promoting nitrogen fixation.26–28 | |
Experimental
Plant growth experiments
The plants of Glycine max (L.) Merr. (soybean) ‘Williams 82’ were used in this study. Briefly, the seeds were surface sterilized in sodium hypochlorite for 4 min under agitation, followed by rinsing 6 times with sterile water, inoculating the seeds into a blank agar dish and keeping the dish at 25 °C in a dark incubator for germination.
To evaluate the effects of different iron/cobalt-based (CoFe2O4-NPs, NiFe2O4-NPs, Co3O4-NPs, Fe2O3-NPs, Co3O4 (50%) + Fe2O3 (50%)-NPs, CoCl2) materials on plant growth, the better germinated soybean seedlings were selected and planted into a matrix, which was river sand without any nutrition (especially free of nitrogen) and was adequately blended with different iron/cobalt-based materials through a soil mixer, and the samples without any additive were used as the control in this experiment. Next, the seeds were cultured in a greenhouse (14 h-light/10 h-dark cycle at a light intensity of 150 μmol m−2 s−2) for 28 days. At day 5 during growth, all the seedlings were inoculated with Bradyrhizobium japonicum strain USDA110 for nodulation assays. Rhizobia were cultivated in YMA liquid medium for 72 h at 28 °C in a shaker (150 rpm), and the bacterial cultures were collected by centrifugation at 5000 rpm for 3 min and washed twice with sterile water. Subsequently, the cells were resuspended in water and adjusted to OD600 = 0.5, followed by adding 1 mL bacterial suspension for each seedling into the mixed matrix and Broughton and Dilworth (B&D) nitrogen-free medium irrigation every three days. At days 14, 21, and 28, the ROS content (histochemical localization and quantitative) and leghemoglobin content were determined for each treatment. At day 28, the stem length and diameter of the seedlings were measured. Meanwhile, the plants were gently removed from the soil and washed, followed by measuring the nodules, above- and under-ground as well as collecting the fresh total roots for the nitrogenase activity test and the fresh nodules for microstructure observation. Finally, tissues of different parts (roots, nodules, stems, and leaves) were oven-dried at 60 °C, ground into powder, and passed through a 60 mesh sieve for total nitrogen analysis, hysteresis loop measurement and inductively coupled plasma-mass spectrometry (ICP-MS).
Nitrogenase activity assay
Nitrogenase activity was measured as acetylene reduction activity (ARA) and the effects of the CoFe2O4 nanozyme on the nitrogenase activity in soybean nodules were quantified by measuring the reduction of acetylene (C2H2) to ethylene (C2H4). The C2H4 content was measured using an Agilent 7820A gas chromatograph (GC) under the following configuration (injection: manual injection, injector temperature: 180 °C, column: GS-Q 30 m × 0.320 mm, carrier gas: pure N2, column flow: 5 mL min−1, oven temperature: 100 °C, detector: FID, detector temperature: 250 °C, H2: 30 mL min−1, air: 400 mL min−1, makeup (N2): 25 mL min−1). C2H2 was prepared by mixing a certain amount of solid calcium carbide (CaC2) with ultra-pure water in a 1 L Buchner flask. The gas was collected by the draining method, and the root with nodules from individual plants was transferred to a sterile 40 mL headspace bottle which was covered tightly with a rubber pad. Next, a syringe was used to draw 4 mL of air from the headspace bottle and inject 4 mL of C2H2 into it to obtain 40 mL of 10% C2H2 mixture, followed by inverting the sample bottles in a 28 °C incubator for 2 h and then manually injecting 100 μL of gas from the headspace bottle into the GC equipment. Finally, two distinct peaks could be observed, and C2H4 showed a shorter retention time than C2H2. After the reaction, the nodules were collected and weighed. Peak areas were estimated and converted into C2H4 concentrations using data from standard curves (Fig. S1†). The C2H4 production rate (μmol min−1) was normalized to the quality (μmol h−1 g−1).
Real-time PCR analysis
Total RNAs were extracted from soybean nodules using a column plant total RNA extraction purification kit (Sangon Biotech). Briefly, a cDNA synthesis kit (Thermo) was used to eliminate the genomic contamination of total RNAs and synthesize the first strand cDNAs. qRT-PCR was performed using the SYBR Green Master Mix reagent. All PCRs were performed using an Analytic Jena (qTOWER 2.2) real-time PCR system in the standard cycling mode: 5 min at 95 °C, followed by 40 cycles of 10 s at 95 °C, 20 s at 60 °C and 20 s at 72 °C. All of the expression levels were analysed and normalised using the ubiquitin gene, which is constitutively expressed in soybean. To compare the expression of nitrogen fixation genes (GmnifA, GmnifD, GmnifH, GmnifK), nodules under exposure to the CoFe2O4 nanozyme and control for 28 d were harvested separately for total RNA isolation and qRT-PCR analysis. The primer sequences used in the expression analysis are shown in Table S1.†
Nanoenzyme verification in vitro
The amount of O2˙− was measured as follows: O2˙− was prepared by mixing 0.4 mL xanthine (X) (0.01 mg mL−1) and 0.4 mL xanthine oxidase (XO) (0.1 U mL−1) in phosphate buffer (0.1 M, pH 7.4) at 37 °C for 40 min, followed by adding 0.2 mL of each CoFe2O4 nanozyme (0.01, 0.05, 0.1, 0.5, and 1 mg mL−1) to the solution for another 40 min reaction and then adding 2′,7′-dichlorodihydrofluorescein diacetate (DCFH-DA) (0.2 mL). After centrifugation, the fluorescence intensity was measured immediately, with DCFH-DA being excited at 488 nm and emitted at 525 nm. Briefly, 60 mM H2O2 was mixed with 1.2 mM 3,3′,5,5′-tetramethylbenzidine (TMB), 0.25 mM horseradish peroxidase (HRP) or 0.4 mL CoFe2O4 nanozyme (0.005, 0.0075, 0.01, 0.015, 0.02, 0.025, 0.05, 0.075, 0.1, 0.15, 0.2, 0.25, 0.5, 0.75, and 1 mg mL−1), vortexed and shocked. After five minutes of reaction, the mixture was centrifuged and the absorbance was measured at 652 nm.
Statistical analysis
All the results were presented as the mean ± standard deviation (±SD) from at least three independent experiments. The experimental data were statistically analysed using IBM SPSS Statistics 22. Comparison between treatments was performed by the independent-sample t-test or one-way ANOVA based on Duncan's multiple range test. The significance levels were determined at *P < 0.05, **P < 0.01, and ***P < 0.001.
Results and discussion
Design and characterization of the CoFe2O4 nanozyme
The morphological features of the CoFe2O4 nanozyme were revealed by transmission electron microscopy (TEM) (Fig. 1a), scanning electron microscopy (SEM) (Fig. 1b) and atomic force microscopy (AFM) (Fig. 1d and S5). The distribution of O, Fe, and Co sites on the nanomaterial was confirmed by in situ energy-dispersive X-ray (EDX) spectroscopy (Fig. S6†), together with elemental mapping (Fig. 1c). In the high-resolution (HR) TEM image (Fig. 1e), the clear lattice fringes with interplanar spacings of 0.48 and 0.24 nm can be attributed to the (111) and (311) planes of the CoFe2O4 nanozyme. Additionally, the nanozyme exhibited a uniform size of ∼50 nm in diameter and a mesoporous structure on the surface as characterized by the particle size test (Fig. S7†) and Brunauer–Emmett–Teller (BET) analysis (Fig. 1f and S8†). In the XRD pattern (Fig. 1g), the CoFe2O4 nanozyme was shown to have characteristic peaks at 18.2°, 30.1°, 35.5°, 43.5°, 53.9°, 57.2°, and 62.7° corresponding to the (111), (220), (311), (400), (422), (511), and (440) planes of spinel CoFe2O4, respectively (JCPDS card No. 22-1086). The related infrared spectra are shown in Fig. 1h and S9.†
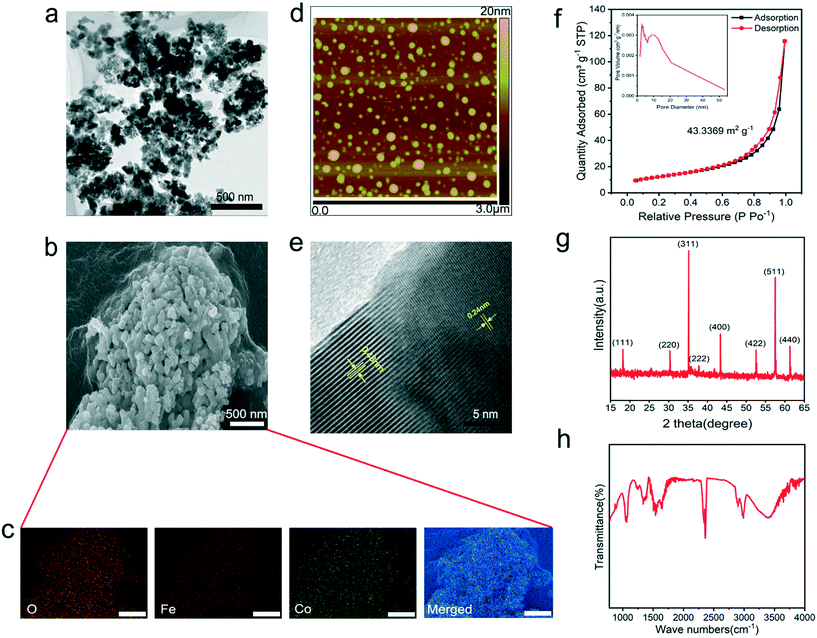 |
| Fig. 1 Characterization of the CoFe2O4 nanozyme. a TEM image of the CoFe2O4 nanozyme. b SEM image of the CoFe2O4 nanozyme. c Mapping of the CoFe2O4 nanozyme. d AFM image of the CoFe2O4 nanozyme. e High-resolution transmission electron microscopy image of the CoFe2O4 nanozyme. f Nitrogen adsorption–desorption isotherms and pore size distribution of CoFe2O4. g XRD pattern of the CoFe2O4 nanozyme. h Fourier transform infrared (FTIR) spectrum of CoFe2O4. | |
In vitro verification of the CoFe2O4 nanozyme ROS-scavenging activity and dynamic stability analysis
Active oxygen is an important stress factor for plants, and the ability of the CoFe2O4 nanozyme to scavenge ROS was evaluated under in vitro conditions. In Fig. 2a and S10,† the CoFe2O4 nanozyme was seen to have catalase activity and present an upward trend at the beginning of the reaction and a downward trend at the end of the reaction, which can be attributed to catalyst poisoning. The subsequent in vitro experiments with the nanozyme confirmed the peroxidase activity and revealed a Michaelis–Menten constant of Km (hydrogen peroxide) = 6.55 ± 0.441 μM (mean ± SD) and Vmax = 0.0226 (Fig. 2b). Meanwhile, active oxygen was generated by mixing xanthine and xanthine oxidase,29 with DCFH-DA as an indicator of active oxygen degradation in the presence of different concentrations of CoFe2O4 nanozyme (0.01, 0.05, 0.1, 0.5, and 1 mg mL−1) (Fig. 2c and d). The results indicated that the nanozyme can degrade active oxygen, with a significant gradient dependence.
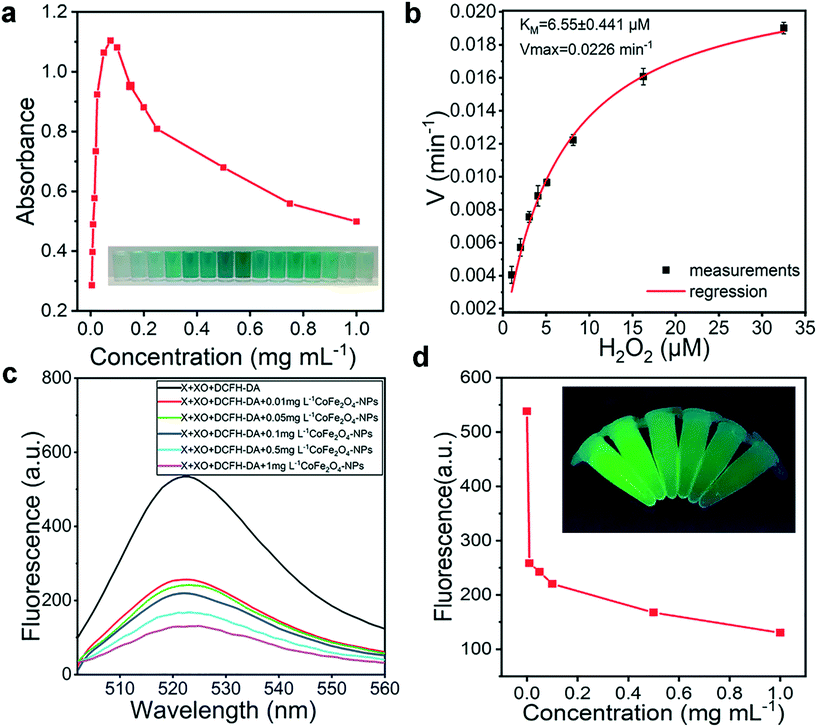 |
| Fig. 2
In vitro verification of CoFe2O4 nanozyme activity. a Verification of the catalase activity of the CoFe2O4 nanozyme. b Hydrogen peroxide kinetics of the CoFe2O4 nanozyme. The Michaelis–Menten kinetic model for hydrogen peroxide was fitted to the nonlinear regression and revealed a Km (hydrogen peroxide) = 6.55 ± 0.441 μM (mean ± SD), with Vmax = 0.0226 (n = 3 technically independent samples; measurements were plotted individually). c Fluorescence spectra of DCFH-DA after the reaction with X and XO in the absence and presence of the CoFe2O4 nanozyme. X for xanthine and XO for xanthine oxidase, respectively. d Corresponding to the maximum fluorescence intensity in c. | |
As the ability of the matrix to remove active oxygen is determined by the dynamic dispersibility of nanomaterials, we evaluated the stability and dispersibility through the changes in the concentration of water-soluble metal ions in the matrix (Fig. S11†) and scanning mapping (Fig. S12†). In the water-soluble iron extracts (Fig. S11a†), CoFe2O4-NPs, NiFe2O4-NPs, Fe2O3-NPs, and Co3O4 + Fe2O3-NPs all showed a significant upward trend in 7–35 days, with a similar content difference. The Co3O4-NPs and control group did not have significant changes in iron accumulation. Meanwhile, in the water-soluble cobalt extracts (Fig. S11b†), CoFe2O4-NPs, Co3O4 + Fe2O3-NPs, and Co3O4-NPs all showed an increasing trend in cobalt accumulation, while NiFe2O4-NPs, Fe2O3-NPs and the control group remained constant. The dispersibility of CoFe2O4-NPs in the mixed matrix is shown in the scanning electron microscopy mapping, and the three elements iron, cobalt and oxygen are uniformly distributed based on sampling analysis at days 10, 20, and 30, indicating that the dispersibility has a mechanism to ensure active oxygen scavenging.
In terms of activity, the CoFe2O4 nanozyme composed of bimetallic transition elements30,31 (a 79.7% yield of styrene oxide (SO) with a total styrene conversion of 96.4%) significantly surpassed other potential nanozymes.32 Due to its relatively small particle size and large surface area (43.34 m2 g−1), the CoFe2O4 nanozyme can promote the adsorption of the substrate on the nanozyme surface as well as the exposure of more active sites for the catalytic reaction, thus allowing a more efficient contact between the substrate and the nanozyme active sites. Consistent with previous studies,32,33 the X-ray photoelectron spectroscopy (XPS) analysis (Fig. S13a–d†) showed that the synergistic interactions between the surface Co2+/Co3+ redox pair and Fe2+/Fe3+ redox pair promoted the kinetics of the surface redox reactions, thus favouring oxidation reactions,34 and based on these mechanisms, nano-reactive oxygen scavengers35 have been developed and are currently applied in the fields of biotherapy36 and plant photosynthesis.27 Nanomaterials, such as Mn3O4,24 CeO2,37 and movable heme-containing mesoporous silica NPs,35 are used as active oxygen scavengers in many materials.
Additionally, the CoFe2O4 nanozyme has been studied intensively as a peroxidase.38 In this study, the CoFe2O4 nanozyme was used to remove H2O2 and oxygen free radicals in vitro, and considerable results were achieved at ppm levels, thus ensuring the function of low-concentration nanozymes in plants. The changes in the concentration of water-soluble iron and cobalt show the leaching kinetics of iron and cobalt in the nanoparticles. Despite the leaching of a large amount of iron and cobalt in the matrix compared to the control, they do not show obvious stress in the plant growth phenotype, and iron or cobalt ion leaching was observed in all the above-mentioned nanoparticles, with exactly the same leaching solution for CoFe2O4-NPs and Co3O4 + Fe2O3-NPs. These results indicate that the positive peroxidase activity of CoFe2O4-NPs does not come from the action of iron or cobalt ions.
Effects of the CoFe2O4 nanozyme on the physiological indices of soybeans (Glycine max)
In order to explore the effects of different nanomaterials on plant growth, several cobalt nanomaterials and ionic chemical drugs were used as screening targets, and their effects on the physiological indicators of soybean plants were statistically analysed during a 28 day growth period. The soybean plants treated with the CoFe2O4 nanozyme at concentrations of 100 and 500 mg kg−1 showed significant improvement (optimal increase by 20.65%) in plant height (Fig. 3a and c, and S14†). Particularly, NiFe2O4-NPs greatly increased the plant height at 10 mg kg−1, but with little effect on the stem diameter. However, the growth in height was not improved by Co3O4-NPs, Fe2O3-NPs, Co3O4 + Fe2O3-NPs and CoCl2 and was obviously inhibited by a high concentration of CoCl2 (553.33 mg kg−1).
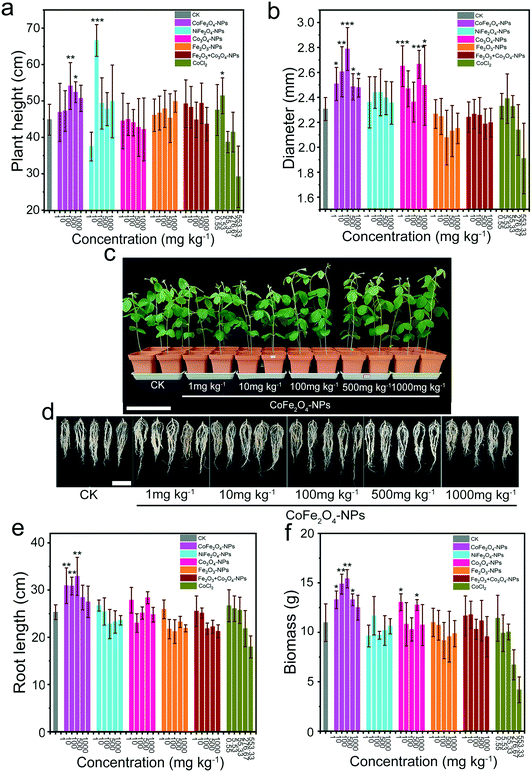 |
| Fig. 3 Effects of different nanomaterials on the growth index of soybean plants. a Plant height when treated with different nanomaterials. b Stem diameter. c Phenotypes when grown in a matrix treated with/without the CoFe2O4 nanozyme for 28 d. Plants were treated without any material (control) or with the CoFe2O4 nanozyme (NiFe2O4-NPs, Co3O4-NPs, Fe2O3-NPs, Co3O4 + Fe2O3-NPs and CoCl2 are shown in Fig. S14†). Scale bar = 330 mm. d Root system of soybeans treated without any material (control) or with the CoFe2O4 nanozyme for 28 d (NiFe2O4-NPs, Co3O4-NPs, Fe2O3-NPs, Co3O4 + Fe2O3-NPs and CoCl2 are shown in Fig. S15†). Scale bar = 100 mm. e Length of the root. f Biomass treated with different nanomaterials. Results are shown as average ± SE using n = 9 individual plants for each condition. Bars with different asterisks represent significant differences (p < 0.05). *p < 0.05, **p < 0.01, and ***p < 0.001 when compared with the control group. | |
The CoFe2O4 nanozyme was shown to promote (optimal increase by 20.86%) the stem diameter growth (Fig. 3b) almost at any concentration. Meanwhile, Co3O4-NPs increased the stem diameter at low (1 mg kg−1) and high (500 and 1000 mg kg−1) concentrations, but with no obvious effect on height. Meanwhile, no increase was observed in the stem diameter after treatment with NiFe2O4-NPs, Fe2O3-NPs, Co3O4 + Fe2O3-NPs and CoCl2, with CoCl2 showing an inhibitory effect on the growth in diameter at high (276.67 and 553.33 mg kg−1) concentrations.
The phenotype images of 28 day-old soybean roots treated with different materials are shown in Fig. 3d and e, and S15.† The roots were seen to be 22.21%, 21.90%, and 30% larger when treated with the CoFe2O4 nanozyme (1, 10, and 100 mg kg−1, respectively) relative to the control, with no significant difference or toxicity observed in the growth of the soybean roots, but larger effects on the root length at higher (1000 mg kg−1 or 553.33 mg kg−1) concentrations of NiFe2O4-NPs, Fe2O3-NPs, Co3O4 + Fe2O3-NPs and CoCl2. The average fresh weights (FWs) of the parts (above- and under-ground) from the 28-day-old soybean seedlings were calculated following exposure to various concentrations of NPs. Changes in nitrogen fixation can be reflected to a certain extent by the total biomass as an indirect indicator (Fig. 3f). Under exposure to the CoFe2O4 nanozyme, the soybean FW gradually increased and reached the maximal value (15.41 ± 0.93 g) at the NP concentration of 100 mg kg−1 relative to the control group (10.97 ± 1.90 g), in contrast to a reduction in the biomass at 500 and 1000 mg kg−1. This pattern was similar for all the tested plant parts. The FW measurement revealed that both the above- (Fig. S16a†) and under-ground (Fig. S16b†) parts contributed to the increase in total biomass, and so did the addition of Co3O4-NPs (1 and 500 mg kg−1).
In the comprehensive analysis of physiological indicators, the cobalt-ferrite-exposed plants showed the optimal growth status, with better above- and under-ground tissue growth than the control group, probably due to the positive impact of the increase in plant nitrogen content, demonstrating the effectiveness of developing an improved nitrogen-fixing symbiotic system. Despite the most significant improvement in height growth, nickel ferrite (10 mg L−1) exhibited no positive effects on the biomass and diameter, which remains to be further elucidated.
Currently, most nanomaterials are synthesized through the bonding of metallic and non-metallic elements, leading to a negative effect in the process of symbiosis with plants due to high salt stress.39–41 Previous studies42 have shown that high concentrations of salt can also cause an increase in ROS, leading to serious damage to the normal growth of plants. In the present study, several nanomaterials were shown to produce stress at high concentrations, especially the ionic reagent CoCl2, because of the presence of various free ions. In contrast, the CoFe2O4 nanozyme could protect normal growth by spontaneously scavenging ROS. Nevertheless, the physiological parameters showed that the CoFe2O4 nanozyme could cause weak stress at the highest (1000 mg kg−1) concentration, despite no significant difference in phenotype.
CoFe2O4 nanozyme promotes nodule enlargement and nitrogen fixation
Based on the growth phenotype, the CoFe2O4 nanozyme showed excellent biocompatibility with soybean growth and development, which was further investigated in terms of nodular enlargement and nitrogen fixation. For this purpose, the number of nodules was evaluated (Fig. 4a), and no significant correlation was found between the total number of nodules and the exposure to the CoFe2O4 nanozyme. The availability of nodules was further evaluated by counting the nodules of each soybean plant through an eight-mesh sieve (the evaluation criteria are shown in Fig. 4b, and the number of larger nodules is shown in Fig. 4c). The exposure to the CoFe2O4 nanozyme was shown to significantly promote the enlargement of nodules, implying that the number of symbiotic rhizobia in the nodules of a soybean can be increased. The effect of the CoFe2O4 nanozyme on the soybean–rhizobia symbiotic nitrogen fixation system was evaluated by analysing the interaction between the CoFe2O4 nanozyme and nodules, with a focus on the effects of the NPs on the nitrogenase activity after a 28 day growth period. The CoFe2O4 nanozyme-exposed plants exhibited more vigorous development than the control, suggesting that the CoFe2O4 nanozyme might positively affect nitrogen fixation in nodules. As shown in Fig. 4d, nitrogen fixation was enhanced in the root nodules exposed to the CoFe2O4 nanozyme (100 and 1000 mg kg−1), with the optimal nitrogenase activity observed at 100 mg kg−1, which was 2.6 times higher than that of the control. The activity of nitrogenase was based on its conversion of C2H2 to C2H4, which means that the enzyme activities of the systems containing the nanomaterials may be derived from the interference of C2H2 by the nanomaterials. To further test this result, we determined the nitrogen content of the whole soybean plant (Fig. 4e) and found that the plant nitrogen levels could be significantly increased by the CoFe2O4 nanozyme at all concentrations, displaying the best effect on the nitrogen content at 100 mg kg−1, with an increase of 33.46% relative to the control.
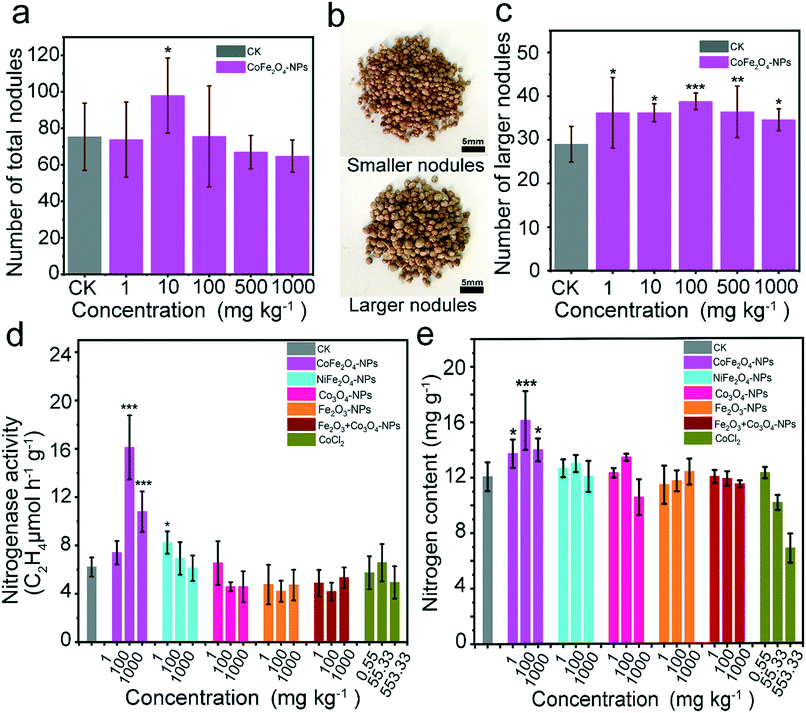 |
| Fig. 4 Evaluation of the nitrogen fixation system for soybean nodules treated with different nanomaterials. a Total number of nodules (n = 5). b Comparison of larger nodules and smaller nodules (screened through an eight-mesh sieve) (n = 5). c Number of larger nodules. d Nitrogenase activity of nodules grown in soils treated with/without nanomaterials for 28 d (n = 5). e Whole plant nitrogen content (n = 3). Results are shown as average ± SD. Bars with different asterisks represent significant differences (p < 0.05). *p < 0.05, **p < 0.01, and ***p < 0.001 when compared with the control group. | |
Previous related studies have demonstrated a negative effect of metal NPs on the nodulation of legumes. ZnO-NPs have been reported to affect root nodulation, delay the onset of nitrogen fixation, and cause early senescence of nodules.40,41,43 TiO2-NP exposure was shown to disrupt the interaction between Rhizobium and soybean while delaying nodule development and the subsequent onset of nitrogen fixation.44 In our previous study, multi-walled carbon nanotubes were shown to significantly up-regulate the early nodulation genes of Lotus japonicus, which promoted the increase of nodulation and nitrogen fixation. In this study, we evaluated the effects of several cobalt nanomaterials and conventional materials on the symbiotic system of leguminous rhizobia, and CoFe2O4-NPs were found to promote nodular expansion and nitrogen fixation. Based on the measurements of the nodule size, the CoFe2O4 nanozyme could promote the nodular enlargement, probably because the low concentrations of ROS create a more favourable condition for this expansion. Overall, the CoFe2O4 nanozyme shows excellent biocompatibility in the interaction with nodules and can be distinguished from other nanomaterials that cause negative effects.
CoFe2O4 nanozyme promotes colonization with rhizobia
Based on the slice observation of mature nodules following 28 days of exposure to the CoFe2O4 nanozyme at 100 mg kg−1, the proportions of the cortex and infected areas in the nodules were generally comparable (Fig. 5a), with multiple vascular bundles between the cortex and infected areas (Fig. 5b), whose structure and cell morphology were intact. Meanwhile, both infected cells and non-infected cells were present in the infected areas, with an intact cell structure (Fig. 5c). To verify the activity of rhizobia in the presence or absence of the CoFe2O4 nanozyme, we observed the distribution of dead or live rhizobia by fluorescence imaging with PI/STYO9 dye staining (Fig. 5d and e). The stress of the CoFe2O4 nanozyme exhibited no specific Rhizobium fatality, and when observing the p-toluidine blue-stained sections, the groups exposed to the CoFe2O4 nanozyme were shown to have a deeper coloration (Fig. 5a–c), probably because the bacteroids are parasitic in the symbiont of rhizobia and nodules, including polyphosphate particles and poly-β-hydroxybutyrate particles, which were stained blue in the toluidine blue-stained sections.
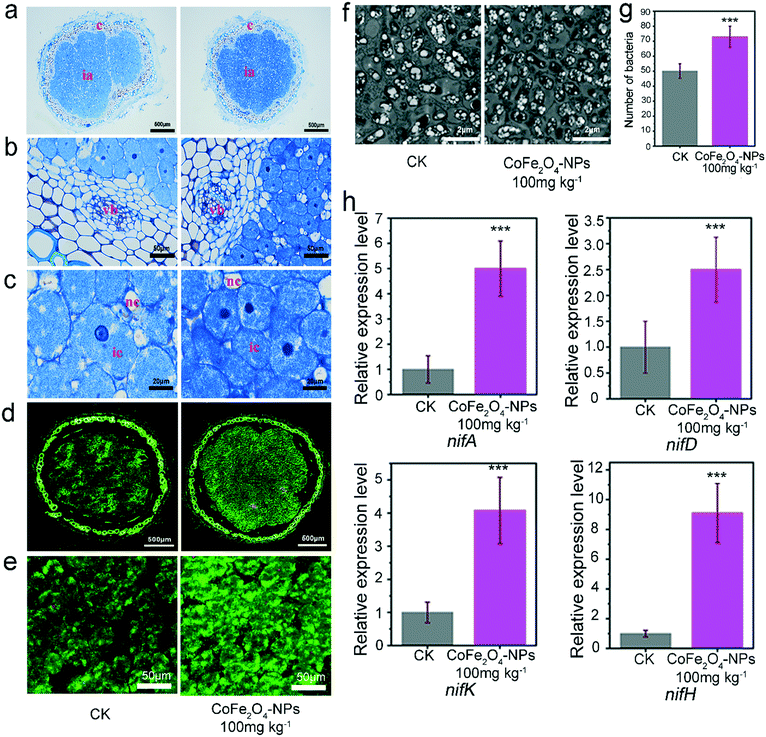 |
| Fig. 5 The difference in the nodular phenotype and rhizobial symbiosis between the control and plants treated with the CoFe2O4 nanozyme at 100 mg kg−1. a Nodular sections, proportion of the cortex (c), and infected areas (ia). Nodules were stained with toluidine blue. Scale bars correspond to 500 μm. b Nodular transduction tissue and vascular bundles (vb) stained with toluidine blue. Scale bars correspond to 50 μm. c Rhizobium-infected cells (ic) and non-infected cells (nc) stained with toluidine blue. Scale bars correspond to 20 μm. d and e Distribution of dead (red)/live (green) bacteria in root nodules stained with STYO9. f Rhizobium distribution. g Rhizobium infection under the same multiple conditions (n = 100). h qRT-PCR analysis of transcript levels in controls and plants treated with the CoFe2O4 nanozyme at 100 mg kg−1. Expression levels were normalized against the reference gene elongation factor (ubiquitin). The RNA was extracted from three individual plants. Bars with different asterisks represent significant differences (p < 0.05). *p < 0.05, **p < 0.01, and ***p < 0.001 when compared with the control group. | |
Based on the staining results of STYO9/PI with more fluorescence inducers (Fig. 5d and e), the group exposed to the CoFe2O4 nanozyme showed more rhizobial colonization. The results from the TEM of soybean nodules (Fig. 5f and S17†) and the number of rhizobial bacteria in 100 randomly selected fields of view further indicated that the CoFe2O4 nanozyme increased the rhizobial colonization by 45.57% (Fig. 5g). To verify this result, we analysed the expression of several genes related to nitrogenases (GmnifA, GmnifD, GmnifH, and GmnifK) in the nodules and their expression was significantly promoted by the exposure to the CoFe2O4 nanozyme (Fig. 5h), which is consistent with the aforementioned results, further confirming that exposure to the CoFe2O4 nanozyme can promote rhizobial colonization.
ROS may stress many biological metabolic processes, including differentiation, transformation, aging, and programmed cell death,45 which have been described as early targets of oxidative modification during nodular senescence.46 Nitrogen fixation is one of the most significant ROS-producing processes,47 mainly due to the elevated respiration rate in high-density bacteroids and the mitochondria of nodular cells as a major source of ROS, particularly O2˙− and H2O2.48 The infected zone of legume nodules is under rigorous physiological stress with respect to O2. Rhizobia are aerobic bacteria that can obtain energy from respiratory metabolism while operating a nitrogenase enzyme system which is extremely sensitive to O2-induced denaturation. Nodules have been reported to produce ROS at free O2 concentrations of 5–60 nM in the central zone.49,50 In the present study, cobalt ferrite nanomaterials were shown to effectively reduce the concentration of active oxygen in the nodules and create a favourable environment for the proliferation of rhizobia.
Distribution of the CoFe2O4 nanozyme in root and nodule tissues
The distribution of the CoFe2O4 nanozyme in the soybean roots and nodular tissues was investigated by measuring the uptake of the CoFe2O4 nanozyme at 100 mg kg−1. The interaction between the CoFe2O4 nanozyme and plants (mainly roots and nodules) was investigated by TEM, ICP-MS, and a hysteresis loop test to show the complete population of the CoFe2O4 nanozyme in the Rhizobium-legume symbiotic system. TEM analysis revealed the ultra-structures of root and nodular cells grown with the CoFe2O4 nanozyme versus the control (without the CoFe2O4 nanozyme) (CK-root and CK-nodule; Fig. 6a). The presence of the clustered CoFe2O4 nanozyme can be clearly observed in the root cells of CoFe2O4 nanozyme-treated plants, accumulating in the root cells in a bare or coated form. NPs may be transported in the form of endocytic membranes during transportation, with the clustered and dispersed CoFe2O4 nanozyme in the nodules, leading to its wide distribution in the rhizobial cells. Iron and cobalt are the hallmark elements of the CoFe2O4 nanozyme, and ICP-MS can reveal their existence in the root, stem, leaf, and nodular cells. In Fig. 6b, the control group showed a wide distribution of iron elements in different tissues, a slight distribution of cobalt in the roots, and no cobalt accumulation in the other tissues. In contrast, the iron ferrite nanomaterial treatment group was significantly (p < 0.001) higher than the control group in iron content in different tissues, particularly the cobalt content. Additionally, the treatment group showed a significant (p < 0.001) increase over the control in iron and cobalt in both stems (Fig. S18a and c†) and leaves (Fig. S18b and d†).
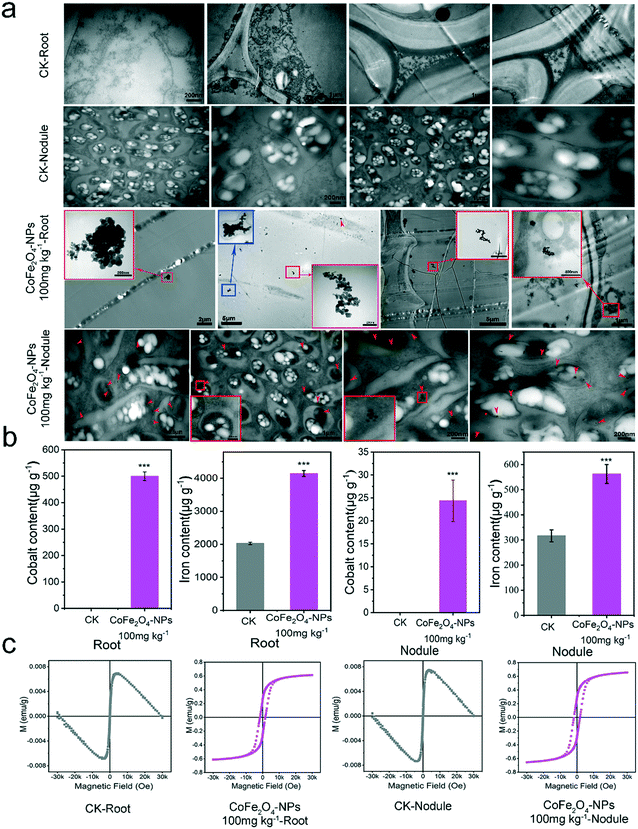 |
| Fig. 6 Distribution of the CoFe2O4 nanozyme in root and nodule tissues. a Low-magnification and high-magnification views of roots and nodules, which show the existence of the CoFe2O4 nanozyme in root and nodule cells. Red signs indicate the CoFe2O4 nanozyme, and red boxes indicate the enlarged regions (n = 3). b Concentration of iron and cobalt ions in roots and nodules at day 28. c Magnetic strength of plants treated with/without the CoFe2O4 nanozyme at 100 mg kg−1. Bars with different asterisks represent significant differences (p < 0.05). *p < 0.05, **p < 0.01, and ***p < 0.001 when compared with the control group. | |
Hysteresis loop measurement analysis is a widely used characterization method for magnetic NPs.51,52 To determine the hysteresis loop, a vibrating sample magnetometer (VSM) was applied. The magnetic properties were shown to be negligible in the control (roots and nodules) (Fig. 6c and S19†), while significant magnetic properties were shown in the soybean roots and nodules exposed to the CoFe2O4 (100 mg kg−1) nanozyme. The hysteresis loop results demonstrated that the CoFe2O4 nanozyme may enter plant tissues with intact magnetic NPs. Studies have shown that artificial nanomaterials can penetrate the cell membranes of animals and plants and enter the protoplasts,39,53 such as nano-CeO2,41,54 nano-ZnO2 (ref. 41) and nano-TiO2 (ref. 55) materials, which can be observed in plant tissues by TEM. The uptake and translocation of nanomaterials have also been reported in different plant species, such as soybean,51 wheat,56 cucumber,54 and alfalfa.52 Magnetic ferrite nanomaterials can scavenge active oxygen in soybean tissues (Fig. 6c).
In vivo verification of active oxygen scavenging by the CoFe2O4 nanozyme
In this study, we detected the leghemoglobin content of soybeans at days 21 and 28 (Fig. 7b), but not at day 14. At day 21, the leghemoglobin showed a significant increase at CoFe2O4 nanozyme concentrations of 500 and 1000 mg kg−1 and reached a significant level at day 28 at 100, 500, and 1000 mg kg−1. A notable enhancement in red colour was also seen in the images at days 21 and 28 (Fig. 7a). Meanwhile, we evaluated the fluorescence intensity in nodules treated or untreated with the CoFe2O4-NP nanozyme by fluorescence imaging of DCFH-DA and ROS using DCFH-DA as a fluorescent probe. Samples were taken at days 14, 21, and 28, respectively (Fig. 7c). At day 14, we observed a significant decrease in fluorescence intensities at 100, 500, and 1000 mg kg−1, and all treatment groups showed a significant reduction in fluorescence intensity at days 21 and 28, with the optimal result at 100 mg kg−1. The optimal ROS scavenging efficiency reached 56.62%, with an inhibitory effect observed under high concentration conditions, which is consistent with the catalyst poisoning observed in the above ex vivo studies (Fig. 2a). In the three different periods, the active oxygen content showed a significant downward trend and was the lowest at maturity (day 28), which was inversely proportional to the leghemoglobin content of the nodules, indicating that this change was attributed to leghemoglobin. For a more intuitive observation of ROS accumulation, we performed in situ slice staining with DCFH-DA (Fig. 7d) and 3,3′-diaminobenzidine (DAB) (Fig. 7e). The green fluorescence area indicated a significant increase in ROS, and the DAB pair dyeing of H2O2 showed the accumulation of a darker colour in the control group, indicative of a higher concentration of reactive H2O2.
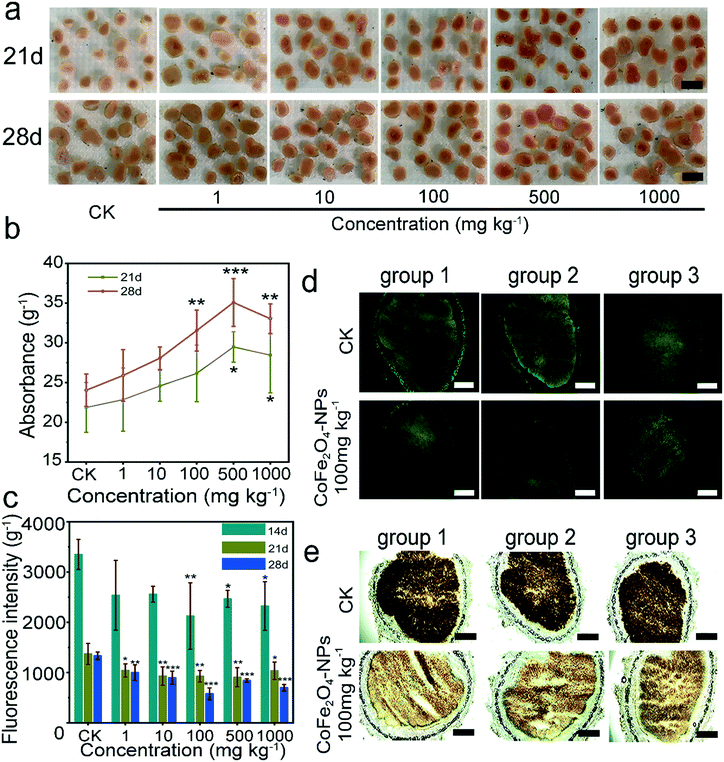 |
| Fig. 7 Evaluation of ROS and leghemoglobin accumulation. a One-half section of a nodule. The accumulation of leghemoglobin under different concentrations (1, 10, 100, 500, and 1000 mg kg−1) at different growth stages (days 21 and 28; bar = 2 mm). b The accumulation of leghemoglobin under different concentrations (1, 10, 100, 500, and 1000 mg kg−1) at different growth stages (days 21 and 28; n = 3). c The accumulation of ROS under different concentrations of CoFe2O4 nanozyme (1, 10, 100, 500, and 1000 mg kg−1) at different growth stages (days 14, 21, and 28; n = 3). d In situ fluorescence staining (DCFH-DA) of reactive oxygen in nodules observed with a fluorescence microscope (bar = 500 μm). e In situ colour development (DAB) of H2O2 in root nodules photographed using an optical microscope (bar = 500 μm). Results are shown as average ± SD. Bars with different asterisks represent significant differences (p < 0.05). *p < 0.05, **p < 0.01, and ***p < 0.001 when compared with the control group. | |
In plant cells, low concentrations of ROS can act as secondary messengers to mediate multiple responses,57 while high concentrations of ROS may cause oxidative damage and even cell death.58 In microorganisms, ROS are a strong oxidant with a wide range of microbicidal effects.59 In this study, the CoFe2O4 nanozyme was shown to create a more favourable condition for the survival of rhizobia and significantly promote the rhizobial colonization, which can also improve the ability of soybeans to fix nitrogen.
Nitrogenase, the core of the nitrogen-fixing ability of legume root nodules, is present in nitrogen-fixing bacteria, and its nitrogen-fixing function is inseparable from the antioxidant protection of leghemoglobin. The active oxygen is fatal to both rhizobia and nitrogenase.60 In the present study, the antioxidant CoFe2O4 nanozyme acted as a synergist of leghemoglobin and promoted its accumulation to a certain extent, thus achieving a quantitative leap in the nitrogen fixation capacity of soybeans.
CoFe2O4 nanozyme enhances the photosynthetic index of soybean leaves
Since chlorophyll is an important indicator of nitrogen content, the contents of chlorophyll a, chlorophyll b, carotenoids, and total chlorophyll in soybean leaves were determined in this study (Fig. 8a). After CoFe2O4 nanozyme exposure, the content of several pigments showed an increase in a highly concentration-dependent manner. The chlorophyll a content showed a significant increase at all concentrations of CoFe2O4 nanozyme, with a concentration-dependent trend and a significant difference between 1000 mg kg−1 and 1 mg kg−1. The chlorophyll b was also seen to increase relative to the control at 100 and 1000 mg kg−1, with a significant difference observed between 1 and 1000 mg kg−1. The carotenoids only showed a significant increase at a higher (1000 mg kg−1) concentration, and the total chlorophyll content also showed a significant and gradual upward trend.
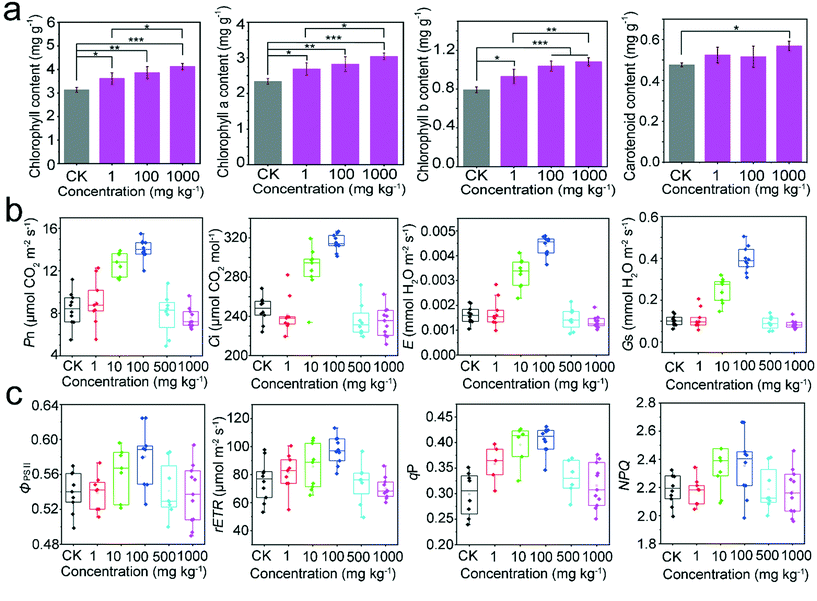 |
| Fig. 8 Plant nano-cooperative enhancement of physiological parameters under exposure to different concentrations of CoFe2O4 nanozyme for 28 d. a Contents of photosynthetic pigments (chlorophyll, chlorophyll a, chlorophyll b, and carotenoid; n = 3). b Photosynthetic parameters. Net photosynthetic rate (Pn), intercellular carbon dioxide concentration (Ci), transpiration rate (E), and stomatal conductance (Gs). c Chlorophyll fluorescence parameters. Actual PSII photochemical efficiency (ΦPSII) relative to the electron transport rate (rETR) of PSII, photochemical quenching coefficient (qP), and non-photochemical quenching coefficient (NPQ). Results are shown as average ± SD and n ≥ 8 individual plants for each condition. Bars with different asterisks represent significant differences (p < 0.05). *p < 0.05, **p < 0.01, and ***p < 0.001 when compared with the control group. | |
In this study, we also explored the variations in nitrogen abundance after exposure to the CoFe2O4 nanozyme at different concentrations by measuring the conventional photosynthetic indicators in soybean leaves, such as the net photosynthetic rate (Pn), intercellular carbon dioxide concentration (Ci), stomatal conductance (Gs), and transpiration rate (E) (Fig. 8b). The results revealed a linear growth trend between 1 and 100 mg kg−1, and the net photosynthetic rate reached the maximum at 100 mg kg−1, with a value 1.67 times higher than that of the control. With a further increase in the concentration, the net photosynthetic rate was significantly suppressed. At 100 mg kg−1, a similar trend was observed in the intercellular carbon dioxide concentration, stomatal conductance, and transpiration rate, which showed a 1.28-, 3.87-, and 2.73-fold increase over the control, respectively.
We detected the relevant chlorophyll fluorescence parameters (Fig. 8c). When compared with the control, the actual photochemical efficiency of PSII (ΦPSII) of soybeans increased first and then declined with the increase of the CoFe2O4 nanozyme concentration, consistent with the concentration effects on the net photosynthetic rate and chlorophyll content, also with the optimal concentration at 100 mg kg−1. With a further increase in the concentration of CoFe2O4 nanozyme, the photochemical quenching coefficient (qP) and PSII relative electron transport rate (rETR) displayed the same trends and were higher than those of the control to varying degrees, which also reached the maximum values at 100 mg kg−1. However, the non-photochemical quenching coefficient (NPQ) showed no significant difference between the control and the CoFe2O4 treatment.
Nitrogen directly participates in chlorophyll synthesis and plays an indispensable role in the synthesis of plant photosynthetic pigments and their photosynthesis-related enzymes. In this study, the concentrations of chlorophyll and CoFe2O4 nanozyme showed an excellent linear relationship, which may be related to the increase in nitrogen content. Additionally, the CoFe2O4 nanozyme was shown to significantly promote soybean photosynthesis at 100 mg kg−1, which can be attributed to an increase in nitrogen content or removal of ROS. This can be supported by a previous study,27 which used cerium oxide to scavenge ROS in Arabidopsis thaliana and found its positive effect on photosynthesis in the plants. However, the exact mechanism remains to be elucidated. Photosynthesis is the main source of carbon fixation. As the level of nitrogen fixation increases, plants will respond quickly to changes in metabolites and synergistically regulate the molecular mechanisms of carbon and nitrogen metabolism, which must reach equilibrium before plants can grow and develop normally.
Conclusions
In this study, a CoFe2O4 nanomaterial with enzyme-like activities was shown to regulate the reactive oxygen metabolism and enhance the symbiotic nitrogen fixation in Glycine max (L.) Merr. (soybean) with the following advantages: (1) the CoFe2O4 nanozyme can enhance the active oxygen metabolism and create a favourable environment for rhizobia to colonize and proliferate in the nodules, thereby generating more effective nodules. (2) The nanozyme may act as a synergist of leghemoglobin and promote its accumulation, thus effectively reducing the concentration of ROS in the nodules, preventing the oxidative damage of nitrogenase and enhancing the symbiotic nitrogen fixation by 260%. (3) The nanozyme could significantly promote the soybean photosynthesis efficiency by increasing the plant nitrogen content and scavenging ROS. Overall, the CoFe2O4 nanozyme acts as a synergist in the soybean nitrogen fixation system and has great potential to improve the efficiency of natural symbiotic nitrogen fixation. However, the application of nanomaterials to large-scale agricultural production may still be limited by costs, and with the development of refined facility agriculture and the innovation of nanomaterial synthesis technology, all of these nanomaterials can be practically used. Further cost–benefit evaluation is needed in future studies.
Conflicts of interest
All authors declare no competing interests.
Acknowledgements
We are grateful to the National Natural Science Foundation of China (21807036, 21778020), the Fundamental Research Funds for the Central Universities (2662016QD027), the Sci-tech Innovation Foundation of Huazhong Agriculture University (2662017PY042, 2662018PY024), and the Science and Technology Major Project of Guangxi (Gui Ke AA18118046) for financial support. We are also thankful to Yang Wu for guidance on drawing.
References
- J. G. Chen, R. M. Crooks, L. C. Seefeldt, K. L. Bren, R. M. Bullock, M. Y. Darensbourg, P. L. Holland, B. Hoffman, M. J. Janik and A. K. Jones, Beyond fossil fuel–driven nitrogen transformations, Science, 2018, 360, eaar6611 CrossRef.
- H. Wang, Y. Li, C. Li, K. Deng, Z. Wang, Y. Xu, X. Li, H. Xue and L. Wang, One-pot synthesis of bi-metallic PdRu tripods as an efficient catalyst for electrocatalytic nitrogen reduction to ammonia, J. Mater. Chem. A, 2019, 7, 801–805 RSC.
- J. S. Anderson, J. Rittle and J. C. Peters, Catalytic conversion of nitrogen to ammonia by an iron model complex, Nature, 2013, 501, 84–88 CrossRef CAS.
- M.-A. Légaré, G. Bélanger-Chabot, R. D. Dewhurst, E. Welz, I. Krummenacher, B. Engels and H. Braunschweig, Nitrogen fixation and reduction at boron, Science, 2018, 359, 896–900 CrossRef.
- C. Lv, Y. Qian, C. Yan, Y. Ding, Y. Liu, G. Chen and G. Yu, Defect Engineering Metal-Free Polymeric Carbon Nitride Electrocatalyst for Effective Nitrogen Fixation under Ambient Conditions, Angew. Chem., Int. Ed., 2018, 57, 10246–10250 CrossRef CAS.
- A. Banerjee, B. D. Yuhas, E. A. Margulies, Y. Zhang, Y. Shim, M. R. Wasielewski and M. G. Kanatzidis, Photochemical nitrogen conversion to ammonia in ambient conditions with FeMoS-chalcogels, J. Am. Chem. Soc., 2015, 137, 2030–2034 CrossRef CAS.
- L. Han, X. Liu, J. Chen, R. Lin, H. Liu, F. Lü, S. Bak, Z. Liang, S. Zhao and E. Stavitski, Atomically dispersed molybdenum catalysts for efficient ambient nitrogen fixation, Angew. Chem., Int. Ed., 2019, 58, 2321–2325 CrossRef CAS.
- G. V. Lowry, A. Avellan and L. M. Gilbertson, Opportunities and challenges for nanotechnology in the agri-tech revolution, Nat. Nanotechnol., 2019, 14, 517–522 CrossRef CAS.
- J. Yang, X. Xie, M. Yang, R. Dixon and Y. P. Wang, Modular electron-transport chains from eukaryotic organelles function to support nitrogenase activity, Proc. Natl. Acad. Sci. U. S. A., 2017, 114, E2460–E2465 CrossRef CAS.
- M. M. M. Kuypers, H. K. Marchant and B. Kartal, The microbial nitrogen-cycling network, Nat. Rev. Microbiol., 2018, 16, 263–276 CrossRef CAS.
- K. Tao, S. Kelly and S. Radutoiu, Microbial associations enabling nitrogen acquisition in plants, Curr. Opin. Microbiol., 2019, 49, 83–89 CrossRef CAS.
- A. Horn, J. H. Van der Meulen, A. Defour, M. Hogarth, S. C. Sreetama, A. Reed, L. Scheffer, N. S. Chandel and J. K. Jaiswal, Mitochondrial redox signaling enables repair of injured skeletal muscle cells, Sci. Signaling, 2017, 10, eaaj1978 CrossRef.
- R. Santos, D. Hérouart, A. Puppo and D. Touati, Critical protective role of bacterial superoxide dismutase in Rhizobium–legume symbiosis, Mol. Microbiol., 2000, 38, 750–759 CrossRef CAS.
- R. L. Robson and J. R. Postgate, Oxygen and hydrogen in biological nitrogen fixation, Annu. Rev. Microbiol., 1980, 34, 183–207 CrossRef CAS.
- B. Halliwell and J. M. Gutteridge, Free radicals in biology and medicine, Free Radical Biol. Med., 1985, 331–332 CrossRef.
- C. C. Winterbourn, Reconciling the chemistry and biology of reactive oxygen species, Nat. Chem. Biol., 2008, 4, 278–286 CrossRef CAS.
- M. Schieber and N. S. Chandel, ROS function in redox signaling and oxidative stress, Curr. Biol., 2014, 24, R453–R462 CrossRef CAS.
- X. D. Sun, X. Z. Yuan, Y. Jia, L. J. Feng, F. P. Zhu, S. S. Dong, J. Liu, X. Kong, H. Tian, J. L. Duan, Z. Ding, S. G. Wang and B. Xing, Differentially charged nanoplastics demonstrate distinct accumulation in Arabidopsis thaliana, Nat. Nanotechnol., 2020, 15, 755–760 CrossRef CAS.
- L. A. Sena and N. S. Chandel, Physiological roles of mitochondrial reactive oxygen species, Mol. Cell, 2012, 48, 158–167 CrossRef CAS.
- L. Gao, J. Zhuang, L. Nie, J. Zhang, Y. Zhang, N. Gu, T. Wang, J. Feng, D. Yang and S. Perrett, Intrinsic peroxidase-like activity of ferromagnetic nanoparticles, Nat. Nanotechnol., 2007, 2, 577–583 CrossRef CAS.
- D. Jiang, D. Ni, Z. T. Rosenkrans, P. Huang, X. Yan and W. Cai, Nanozyme: new horizons for responsive biomedical applications, Chem. Soc. Rev., 2019, 48, 3683–3704 RSC.
- K. Fan, J. Xi, L. Fan, P. Wang, C. Zhu, Y. Tang, X. Xu, M. Liang, B. Jiang, X. Yan and L. Gao,
In vivo guiding nitrogen-doped carbon nanozyme for tumor catalytic therapy, Nat. Commun., 2018, 9, 1440 CrossRef.
- H. Wang, K. Wan and X. Shi, Recent advances in nanozyme research, Adv. Mater., 2018, 31, 1805368 CrossRef.
- J. Yao, Y. Cheng, M. Zhou, S. Zhao, S. Lin, X. Wang, J. Wu, S. Li and H. Wei, ROS scavenging Mn3O4 nanozymes for in vivo anti-inflammation, Chem. Sci., 2018, 9, 2927–2933 RSC.
- S. Ghosh, P. Roy, N. Karmodak, E. D. Jemmis and G. Mugesh, Nanoisozymes: Crystal-Facet-Dependent Enzyme-Mimetic Activity of V2O5 Nanomaterials, Angew. Chem., Int. Ed., 2018, 130, 4600–4605 CrossRef.
- L. Su, W. Qin, H. Zhang, Z. U. Rahman, C. Ren, S. Ma and X. Chen, The peroxidase/catalase-like activities of MFe2O4 (M=Mg, Ni, Cu) MNPs and their application in colorimetric biosensing of glucose, Biosens. Bioelectron., 2015, 63, 384–391 CrossRef CAS.
- H. Wu, N. Tito and J. P. Giraldo, Anionic Cerium Oxide Nanoparticles Protect Plant Photosynthesis from Abiotic Stress by Scavenging Reactive Oxygen Species, ACS Nano, 2017, 11, 11283–11297 CrossRef CAS.
- J. Mu, L. Zhang, M. Zhao and Y. Wang, Catalase mimic property of Co3O4 nanomaterials with different morphology and its application as a calcium sensor, ACS Appl. Mater. Interfaces, 2014, 6, 7090–7098 CrossRef CAS.
- J. J. Hu, N. K. Wong, S. Ye, X. Chen, M. Y. Lu, A. Q. Zhao, Y. Guo, A. C. Ma, A. Y. Leung, J. Shen and D. Yang, Fluorescent Probe HKSOX-1 for Imaging and Detection of Endogenous Superoxide in Live Cells and In Vivo, J. Am. Chem. Soc., 2015, 137, 6837–6843 CrossRef CAS.
- Y. Liu, Y. Su, X. Quan, X. Fan, S. Chen, H. Yu, H. Zhao, Y. Zhang and J. Zhao, Facile Ammonia Synthesis from Electrocatalytic N2 Reduction under Ambient Conditions on N-Doped Porous Carbon, ACS Catal., 2018, 8, 1186–1191 CrossRef CAS.
- P. J. Hill, L. R. Doyle, A. D. Crawford, W. K. Myers and A. E. Ashley, Selective Catalytic Reduction of N2 to N2H4 by a Simple Fe Complex, J. Am. Chem. Soc., 2016, 138, 13521–13524 CrossRef CAS.
- J. Liu, R. Meng, J. Li, P. Jian, L. Wang and R. Jian, Achieving high-performance for catalytic epoxidation of styrene with uniform magnetically separable CoFe2O4 nanoparticles, Appl. Catal., B, 2019, 254, 214–222 CrossRef CAS.
- M. Xu, J. Li, Y. Yan, X. Zhao, J. Yan, Y. Zhang, B. Lai, X. Chen and L. Song, Catalytic degradation of sulfamethoxazole through peroxymonosulfate activated with expanded graphite loaded CoFe2O4 particles, Chem. Eng. J., 2019, 369, 403–413 CrossRef CAS.
- S. Lei, Q. H. Li, Y. Kang, Z. G. Gu and J. Zhang, Epitaxial growth of oriented prussian blue analogue derived well-aligned CoFe2O4 thin film for efficient oxygen evolution reaction, Appl. Catal., B, 2019, 245, 1–9 CrossRef CAS.
- M. Lian, Z. Xue, X. Qiao, C. Liu, S. Zhang, X. Li, C. Huang, Q. Song, W. Yang, X. Chen and T. Wang, Movable Hollow Nanoparticles as Reactive Oxygen Scavengers, Chem, 2019, 5, 2378–2387 CAS.
- Y. Fan, P. Li, B. Hu, T. Liu, Z. Huang, C. Shan, J. Cao, B. Cheng, W. Liu and Y. Tang, A Smart Photosensitizer-Cerium Oxide Nanoprobe for Highly Selective and Efficient Photodynamic Therapy, Inorg. Chem., 2019, 58, 7295–7302 CrossRef CAS.
- J. Yang, X. Xie, M. Yang, R. Dixon and Y. P. Wang, Modular electron-transport chains from eukaryotic organelles function to support nitrogenase activity, Proc. Natl. Acad. Sci. U. S. A., 2017, 114, E2460–E2465 CrossRef CAS.
- W. Shi, X. Zhang, S. He and Y. Huang, CoFe2O4 magnetic nanoparticles as a peroxidase mimic mediated chemiluminescence for hydrogen peroxide and glucose, Chem. Commun., 2011, 47, 10785–10787 RSC.
- H. Zhang, W. Du, J. R. Peralta-Videa, J. L. Gardea-Torresdey, J. C. White, A. Keller, H. Guo, R. Ji and L. Zhao, Metabolomics Reveals How Cucumber (Cucumis sativus) Reprograms Metabolites To Cope with Silver Ions and Silver Nanoparticle-Induced Oxidative Stress, Environ. Sci. Technol., 2018, 52, 8016–8026 CrossRef CAS.
- J. H. Priester, S. C. Moritz, K. Espinosa, Y. Ge, Y. Wang, R. M. Nisbet, J. P. Schimel, A. Susana Goggi, J. L. Gardea-Torresdey and P. A. Holden, Damage assessment for soybean cultivated in soil with either CeO2 or ZnO manufactured nanomaterials, Sci. Total Environ., 2017, 579, 1756–1768 CrossRef CAS.
- J. H. Priester, Y. Ge, R. E. Mielke, A. M. Horst, S. C. Moritz, K. Espinosa, J. Gelb, S. L. Walker, R. M. Nisbet, Y. J. An, J. P. Schimel, R. G. Palmer, J. A. Hernandez-Viezcas, L. Zhao, J. L. Gardea-Torresdey and P. A. Holden, Soybean susceptibility to manufactured nanomaterials with evidence for food quality and soil fertility interruption, Proc. Natl. Acad. Sci. U. S. A., 2012, 109, E2451–E2456 CrossRef CAS.
- A. Oukarroum, F. Bussotti, V. Goltsev and H. M. Kalaji, Correlation between reactive oxygen species production and photochemistry of photosystems I and II in Lemna gibba L. plants under salt stress, Environ. Exp. Bot., 2015, 109, 80–88 CrossRef CAS.
- Y. C. Huang, R. Fan, M. A. Grusak, J. D. Sherrier and C. P. Huang, Effects of nano-ZnO on the agronomically relevant Rhizobium-legume symbiosis, Sci. Total Environ., 2014, 497–498, 78–90 CrossRef CAS.
- R. Fan, Y. C. Huang, M. A. Grusak, C. P. Huang and D. J. Sherrier, Effects of nano-TiO2 on the agronomically-relevant Rhizobium-legume symbiosis, Sci. Total Environ., 2014, 466–467, 503–512 CrossRef CAS.
- G. Noctor, J. P. Reichheld and C. H. Foyer, ROS-related redox regulation and signaling in plants, Semin. Cell Dev. Biol., 2018, 80, 3–12 CrossRef CAS.
- A. Saiz, M. A. Matamoros, N. Fernández-García, S. Wienkoop, J. Loscos and M. Becana, Mitochondria are an early target of oxidative modifications in senescing legume nodules, New Phytol., 2013, 197, 873–885 CrossRef.
- M. A. Matamoros, D. A. Dalton, J. Ramos, M. R. Clemente, M. C. Rubio and M. Becana, Biochemistry and molecular biology of antioxidants in the rhizobia-legume symbiosis, Plant Physiol., 2003, 133, 499–509 CrossRef CAS.
- I. M. Møller, Plant mitochondria and oxidative stress: Electron Transport, NADPH Turnover, and Metabolism of Reactive Oxygen Species, Annu. Rev. Plant Physiol. Plant Mol. Biol., 2001, 52, 561–591 CrossRef.
- C. A. Appleby, Leghemoglobin and rhizobium respiration, Annu. Rev. Plant Physiol., 1984, 35, 443–478 CrossRef CAS.
- D. B. Layzell and S. Hunt, Gas exchange of legume nodules and the regulation of nitrogenase activity, Annu. Rev. Plant Physiol. Plant Mol. Biol., 1993, 44, 483–511 CrossRef.
- M. H. Ghafariyan, M. J. Malakouti, M. R. Dadpour, P. Stroeve and M. Mahmoudi, Effects of magnetite nanoparticles on soybean chlorophyll, Environ. Sci. Technol., 2013, 47, 10645–10652 CAS.
- J. H. Kim, D. Kim, S. M. Seo and D. Kim, Physiological effects of zero-valent iron nanoparticles in rhizosphere on edible crop, Medicago sativa (Alfalfa), grown in soil, Ecotoxicology, 2019, 28, 869–877 CrossRef CAS.
- F. Li, T. Li, C. Sun, J. Xia, Y. Jiao and H. Xu, Selenium-Doped Carbon Quantum Dots for Free-Radical Scavenging, Angew. Chem., Int. Ed., 2017, 56, 9910–9914 CrossRef CAS.
- Y. H. Ma, P. Zhang, Z. Zhang, X. He, J. Zhang, Z. Guo, R. Tai, Y. Zhao and Z. Chai, Biotransformation of Ceria Nanoparticles in Cucumber Plants, ACS Nano, 2012, 6, 9943–9950 CrossRef.
- S. Timmusk, G. Seisenbaeva and L. Behers, Titania (TiO2) nanoparticles enhance the performance of growth-promoting rhizobacteria, Sci. Rep., 2018, 8, 617–630 CrossRef.
- A. Avellan, J. Yun, Y. Zhang, E. Spielman-Sun, J. M. Unrine, J. Thieme, J. Li, E. Lombi, G. Bland and G. V. Lowry, Nanoparticle Size and Coating Chemistry Control Foliar Uptake Pathways, Translocation, and Leaf-to-Rhizosphere Transport in Wheat, ACS Nano, 2019, 13, 5291–5305 CrossRef CAS.
- M. Yamada, X. Han and P. N. Benfey, RGF1 controls root meristem size through ROS signalling, Nature, 2020, 577, 85–88 CrossRef CAS.
- Y. Zhao, H. Yu, J. M. Zhou, S. M. Smith and J. Li, Malate Circulation: Linking Chloroplast Metabolism to Mitochondrial ROS, Trends Plant Sci., 2020, 25, 446–454 CrossRef.
- F. Xiao, B. Cao, C. Wang, X. Guo, M. Li, D. Xing and X. Hu, Pathogen-Specific Polymeric Antimicrobials with Significant Membrane Disruption and Enhanced Photodynamic Damage To Inhibit Highly Opportunistic Bacteria, ACS Nano, 2019, 13, 1511–1525 CrossRef CAS.
- L. Wang, M. C. Rubio, X. Xin, B. Zhang, Q. Fan, Q. Wang, G. Ning, M. Becana and D. Duanmu, CRISPR/Cas9 knockout of leghemoglobin genes in Lotus japonicus uncovers their synergistic roles in symbiotic nitrogen fixation, New Phytol., 2019, 224, 818–832 CrossRef CAS.
Footnotes |
† Electronic supplementary information (ESI) available. See DOI: 10.1039/d0en00935k |
‡ These authors contributed equally to this work: Jun Ma, Zhiyong Song. |
|
This journal is © The Royal Society of Chemistry 2021 |
Click here to see how this site uses Cookies. View our privacy policy here.