Surface modification mediates the interaction between fullerene and lysozyme: protein structure and antibacterial activity†
Received
19th June 2020
, Accepted 12th October 2020
First published on 13th October 2020
Abstract
Chemical functionalization is widely adopted to increase the hydrophilicity of fullerene for various applications. Protein–nanomaterial (NM) interactions are one of the very first issues after NMs enter biological systems, which determines their bio-effects and toxicity. To better understand the biological and environmental effects of fullerene, we investigated the influence of surface modification on the fullerene–protein interaction by experimental and computational approaches. Pristine fullerene (C60) was functionalized to obtain esterified fullerene (C60–COOR), carboxylated fullerene (C60–COOH) and fullerenol (C60–OH), resulting in higher hydrophilicity and dispersibility following the sequence C60–OH > C60–COOH > C60–COOR > C60. The docking results suggested that good hydrophilic functionalization largely reduced the π–π interaction between aromatic residues and the fullerene cage, resulting in a weaker interaction between fullerene and lysozyme. Experimentally, the chemically functionalized fullerene showed more inhibition of enzyme activity. Correspondingly, the protein conformation changed more after incubation with functionalized fullerene with hydrophilic moieties (C60–COOH and C60–OH), indicated by the increased UV absorbance, the inhibition of intrinsic fluorescence and the loss of the α-helix structure. The contradictory results of computational and experimental approaches were attributed to the different dispersibilities, where functionalized fullerene with hydrophilic moieties dispersed well in aqueous systems to reach the lysozyme active pocket more easily. As a direct bio-effect, the dispersible fullerenes inhibited the antibacterial activity of lysozyme, which might affect the resistance to infection and the applications of lysozyme in biomedicine, food and bioengineering. Our findings suggested that surface modification should be carefully designed to obtain safer carbon NMs and enough consideration should be paid to the protein–nanomaterial interaction in environmental toxicity evaluations.
Environmental significance
Protein–nanomaterial interactions are one of the very first issues after nanomaterials enter biological systems, and determine their fate and toxicity. Fullerenes are novel nanomaterials for various applications, whose environmental release and toxicity have been widely reported. To elucidate the mechanism of surface chemistry regulated bio-effects, we compared the fullerene–protein interaction of four fullerene samples following the hydrophilicity sequence C60–OH > C60–COOH > C60–COOR > C60. Surface modification reduced the π–π interaction between aromatic residues and the fullerene cage, but hydrophilic functionalization helped the fullerenes disperse well to reach the lysozyme active pocket more easily, resulting in stronger fullerene–lysozyme binding. C60–OH showed the strongest fullerene–lysozyme interaction, and induced more structural changes and activity loss. These findings benefit the understanding of nanomaterial interactions with biological systems at the protein level.
|
Introduction
Carbon nanomaterials (NMs) are of particular interest and importance due to their unique structure and properties.1 Carbon NMs find applications in diverse areas, such as electronics, energy, environmental technology, personal care, biomedicine, and so on.2–5 The production of carbon NMs keeps increasing and commercial products of carbon NMs are available nowadays. With these great achievements, the biosafety of carbon NMs has become a crucial issue to address.6–8 Many studies have shown the potential toxicity of carbon NMs to cells, animals and plants.6–8 Due to their large surface area and high affinity to proteins, carbon NMs would adsorb proteins to form protein coronae after entering biological systems.9,10 The composition of protein coronae depends on the interactions between different proteins and carbon NMs, which changes the surface chemistry and biological behaviours of carbon NMs.9,10 To fully understand the bio-effects and bio-safety of carbon NMs, there is a growing need to push nanotoxicity studies to the molecular level and understand the biomolecule–nanomaterial interactions.11
Proteins are large biomolecules consisting of long chains of amino acid residues and play crucial roles in life. There are several key interactions between NMs and proteins, such as hydrophobic interactions, H-bonding, π–π interactions and van der Waals (vdw) interactions.10 Thus, when NMs enter biological systems, proteins would immediately bind to their surface as the very first issue regarding the bio-effects of NMs. In a protein enriched microenvironment, the surface properties of NMs might change, which consequently alters their behaviours and bio-effects. For example, Barbalinardo et al. found that silver nanoparticles (NPs) formed protein coronae in serum that enhanced the cellular uptake and cell death of fibroblasts.12 On the other hand, when proteins adsorb on NMs, the protein conformation and activity might change, too. The loss of normal conformation would lead to the disturbance of protein functions, which might further change the toxicity and applications of proteins. For instance, protein fibrillation is the main reason for Alzheimer's disease. Gold NPs could bind to amyloids through electrostatic interaction, depolymerize the fibrils, and reduce the toxicity of fibrils to neural cells.13 Therefore, the protein–NM interactions and their bio-effects on proteins are crucial for the bio-applications and biosafety of NMs.14,15
For carbon NMs in particular, many studies have revealed their toxicity and environmental risks.6–8 Changing the conformation and function of proteins is regarded as a potential toxicological mechanism of carbon NMs.9,10 Carbon NMs interact with proteins mainly through the π–π interaction. The π–π interaction is between the aromatic rings of the sp2 carbon domain on carbon NMs and the aromatic residues of proteins. Based on computational results, chemical functionalization would break the aromatic domains and decrease the π–π interaction; thus, a weaker protein–nanomaterial interaction is expected.16–18 For instance, Wu et al. docked hydroxylated fullerene to lysozyme and RNase A, where more hydroxyl groups led to weaker protein–nanomaterial interaction.16 However, recent studies on graphene showed contradictory results where oxidation of graphene largely enhanced the protein–nanomaterial interaction and the serious inhibition of lysozyme activity was observed with graphene oxide (GO).19 Similar observations were reported by other researchers, too.20,21 The contradictory results between computational and experimental results require further investigations. Moreover, graphene and other carbon NMs are complicated and heterogeneous in chemical structure, making such investigations very difficult.
Fullerenes have a precise cage structure and are easy to functionalize; thus, fullerene (C60) is an ideal model to investigate the protein–carbon nanomaterial interactions.22 Beyond that, fullerenes are the most typical carbon nanomaterials, and have attracted widespread attention in the scientific community due to their unique physical and chemical properties.23 Fullerenes are applied in catalysts, hydrogen storage materials, superconducting materials, biomedicine, cosmetics, and so on.23 Fullerenes have been found in environmental samples, such as meteorites, geological samples, soot from ordinary combustion processes, the atmosphere and polar ice cores.24,25 The environmental concentrations of fullerenes have been modelled and analysed.26 The environmental release of fullerenes was observed in Eastern China.27 Thus, fullerenes are highly relevant to the environment. In fact, the environmental safety and hazards of fullerenes have been widely considered using different models, such as white rot fungi,28Daphnia magna,29 and plants.30 The results of protein–fullerene interactions would strengthen these toxicity studies and benefit the understanding of their environmental effects.10
Previously, several groups reported that fullerene could fit into the active pocket of enzymes to inhibit their activity and induce conformational changes,31–33 but the influence of surface modification on the protein–fullerene interaction has not been evidenced by experimental measurements. Therefore, we bought pristine fullerene C60, prepared three functionalized fullerene samples, and compared the protein–fullerene interactions among the four fullerene samples (Fig. 1). The fullerene samples were characterized by multiple techniques. The fullerene samples were docked to lysozyme using AutoDock 4.2 software. The protein conformational changes were investigated using UV-vis spectra, intrinsic fluorescence (FL) spectra and circular dichroism (CD) spectra. The lysozyme activities and the antibacterial activities after the incubation with the fullerene samples were measured. The role of surface functionalization in the protein–nanomaterial interactions was revealed by comparing the computational and experimental data. Their implications for the toxicity and environmental effects of nanomaterials are discussed.
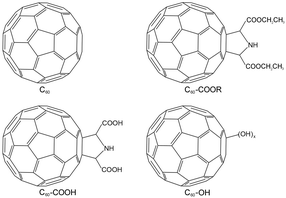 |
| Fig. 1 Structure of C60, C60–COOR, C60–COOH and C60–OH. | |
Results
Characterization of fullerenes
Fullerene and its derivatives were prepared and characterized following our previous reports.28,34 Under TEM, the small aggregates were presented (Fig. 2). The aggregations of fullerene C60 were more obvious, as indicated by the formation of larger particles. Small dots were stacked together in C60–COOR. For the dispersible C60–COOH and C60–OH, the aggregation was mild and only a few particles were found. It should be noted that during the TEM sampling, the drying of solvents would drive the aggregation of samples and change their morphology. The situation was different in DLS analyses (Fig. 3). Upon vigorous sonication, the average diameters were measured as 542 ± 391 nm for C60, 569 ± 481 nm for C60–COOR and 411 ± 325 nm for C60–COOH. The most distinct pattern was found for C60–OH, where single-dispersed C60–OH was identified as the peak at 0.67 ± 0.07 nm. The aggregates of C60–OH were reflected by the peaks at 607.9 ± 135.2 nm and 9.1 ± 1.5 nm. If a fullerene dispersion was stable against centrifugation of 3000 rpm for 5 min, we defined it as dispersible. C60 and C60–COOR could not disperse well in water and the dispersion was still muddy after sonication. C60–COOH and C60–OH formed a brown dispersion in water due to the hydrophilic groups on the fullerene cage. Obviously, C60–OH dispersed best in water with singly dispersed cages.
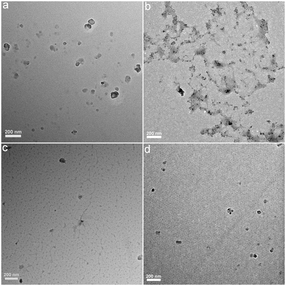 |
| Fig. 2 Representative TEM images of C60 (a), C60–COOR (b), C60–COOH (c) and C60–OH (d). | |
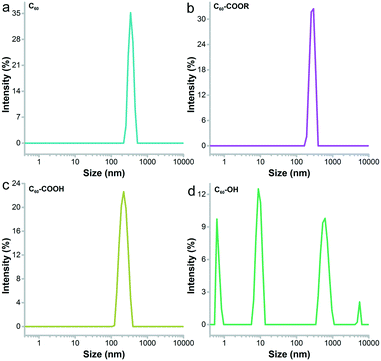 |
| Fig. 3 DLS spectra of C60 (a), C60–COOR (b), C60–COOH (c) and C60–OH (d). | |
The IR spectra indicated the fullerene cage at about 1640 cm−1 for sp2 carbon (Fig. S1†). The –OH/–COOH/–NH groups were found at 3450 cm−1 in all fullerene samples. The tiny peaks at 2925 cm−1 were assigned to –CH2–/–CH– for both C60–COOR and C60–COOH. The chemical states of carbon atoms were also analysed by XPS. It could be seen in Fig. 4 that even for pristine C60, there were oxygen atoms introduced on the fullerene cages, which were reflected by the sp3 carbon (285.2 eV) and C–O (286.2 eV) components. For C60–COOR, there were four carbon types, namely sp2 carbon (284.7 eV), sp3 carbon (285.5 eV), C–N (286.2 eV) and C
O (288.4 eV). For C60–COOH, there were also four carbon types, including sp2 carbon (284.8 eV), sp3 carbon (285.5 eV), C–N (286.6 eV) and C
O (288.5 eV). For C60–OH, there were sp2 carbon (284.8 eV), C–O–C (285.5 eV), C–O (286.5 eV) and C
O (288.9 eV). The characterization results suggested that fullerene was efficiently functionalized and suitable for the protein–fullerene interaction evaluations.
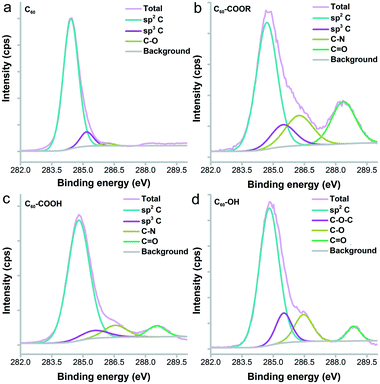 |
| Fig. 4 C1s XPS spectra of C60 (a), C60–COOR (b), C60–COOH (c) and C60–OH (d). | |
Docking of fullerenes to lysozyme
AutoDock is an automated docking tool for predicting the binding of small molecules to receptors. It has been used to study the interactions between proteins and nanoparticles.35,36 In particular, AutoDock has been successfully applied in docking the binding of fullerene to proteins.16,37 According to the docking results, all four fullerene samples were located in the active pocket of lysozyme (Fig. 5). In the docking experiments, single fullerene cages with nearly the same diameters were used for all four samples. A π–π interaction was found between the fullerene cage and the aromatic ring of the tryptophan (Trp) residue as the main driving force. H-Bonding was also a contributor to the total binding strength of the fullerene derivatives, but is much weaker than the π–π interaction. The high degree of functionalization destroyed the integrity of the fullerene cage and weakened the π–π interaction. Consequently, the binding capacity of the surface chemical modified fullerene nanomaterials should decrease, where the binding energy showed an increasing trend from −9.62 kcal mol−1 for C60 to −5.58 kcal mol−1 for C60–OH. Here, both C60–COOR (−12.93 kcal mol−1) and C60–COOH (−11.09 kcal mol−1) with one modified group kept the fullerene cage and the moieties squeezed into the pocket deeper; therefore, the binding strength increased slightly. Thus, the binding strength followed the sequence C60–COOR > C60–COOH > C60 ≫ C60–OH.
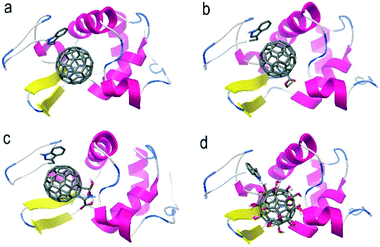 |
| Fig. 5 Binding models of fullerenes with lysozyme. (a) C60; (b) C60–COOR; (c) C60–COOH; (d) C60–OH. | |
Conformational changes
The main changes of UV-vis absorbance came from the range of 240–340 nm, which indicated the conformational changes of proteins.38 As shown in Fig. 6, the absorbance of lysozyme increased obviously after the incubation with C60–OH following a concentration-dependent way. No peak shift was observed for lysozyme incubated with C60–OH. The increased intensity at 282 nm indicated that C60–OH interacted with the aromatic amino acids (Trp and tyrosine, Tyr).38 In contrast, C60 led to the concentration-dependent decrease of absorbance, which might be due to the co-precipitation of lysozyme and C60 as indicated by the brown aggregates in the residue. For C60–COOR, decreases were observed at 2 and 5 μg mL−1 and a small increase was found at 40 μg mL−1. For C60–COOH, a decrease was found at 2 μg mL−1 and recovery was observed at 5 μg mL−1. A large increase was found at 10 μg mL−1 in the C60–COOH exposed group.
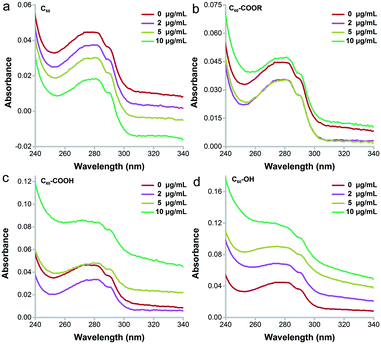 |
| Fig. 6 UV-vis absorption spectra of C60 (a), C60–COOR (b), C60–COOH (c) and C60–OH (d). | |
Then, far-UV CD spectroscopy was adopted to characterize the changes of secondary structure (Fig. 7). C60–OH induced serious unfolding of lysozyme, which led to a concentration-dependent loss of the α-helix structure. Along with the increase of C60–OH concentration, the far-UV CD spectra showed an increasing trend at 208 nm, suggesting the decrease of the α-helix content. C60–COOH and C60–COOR only led to an increase of intensity at 208 nm at 40 μg mL−1, and C60–COOH induced a higher increase. The situation was quite different for C60, where the far-UV CD spectra had no changes. Therefore, the α-helix loss followed the sequence C60 < C60–COOR < C60–COOH ≪ C60–OH.
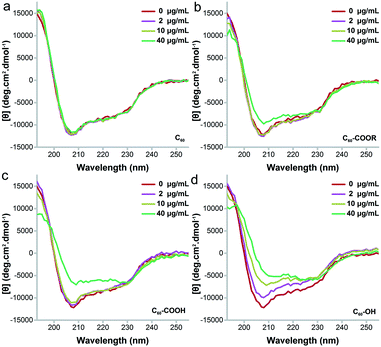 |
| Fig. 7 Far-UV CD spectra of C60 (a), C60–COOR (b), C60–COOH (c) and C60–OH (d). | |
Intrinsic fluorescence is a widely applied approach to investigate the surrounding environment of aromatic amino acid residues.39Fig. 8 presents the decreasing trend of intrinsic fluorescence for lysozyme incubated with fullerene and its derivatives. The decrease was much more serious after incubation with C60–OH and C60–COOH and showed a concentration-dependent pattern. Even at 2 μg mL−1, meaningful fluorescence decreases were observed in the C60–OH and C60–COOH groups. At 10 μg mL−1, the decrease of fluorescence for the C60–OH and C60–COOH groups was 100%, the decrease of fluorescence for the C60–COOR group was 57%, and the value was less than 10% for the C60 group. This indicated that C60–OH and C60–COOH induced more conformational changes to lysozyme. The excitation wavelength was 280 nm, which excited both Trp and Tyr residues of lysozyme. Tyr residues could not be excited at 295 nm and the excitation at 295 nm showed the nearly identical quenching trend of fluorescence to that at 280 nm for C60–OH and C60–COOH (data not shown). This suggested that C60–OH and C60–COOH only influenced the Trp residues, while C60–COOR might have influenced both Tyr and Trp. C60 hardly influenced the amino acid residues and the intrinsic fluorescence was not changed. Based on the ΔF values at 280 nm excitation, we calculated the KD values of the fullerene–protein complexes. The KD of lysozyme–C60–OH (KD = 5.522) was similar that of lysozyme–C60–COOH (KD = 5.604) and much smaller than that of lysozyme–C60–COOR (KD = 13.193), indicating that the binding strength between lysozyme and C60–OH was the strongest. Thus, the influence of fullerene samples on the intrinsic fluorescence was in the order C60 ≪ C60–COOR < C60–COOH ≈ C60–OH.
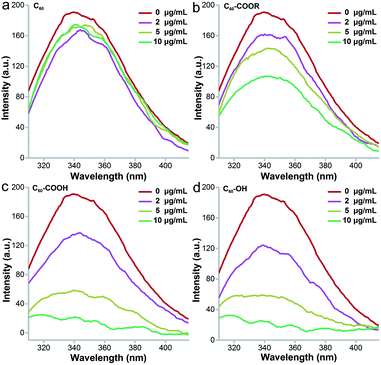 |
| Fig. 8 Intrinsic FL spectra of C60 (a), C60–COOR (b), C60–COOH (c) and C60–OH (d). | |
Antibacterial activity
The most obvious change of lysozyme after incubating with fullerene and its derivatives was the enzyme activity alteration. As shown in Fig. 9a, C60–OH and C60–COOH showed a strong inhibitory effect on lysozyme activity. On the other hand, C60–COOR had very little influence on the enzyme activity and C60 showed almost no effect. At 2 μg mL−1, C60–OH, C60–COOH and C60 inhibited the enzyme activity slightly (11.7%, 2.2% and 3.1%), while C60–COOR showed very small stimulation (5.2%). With the increase of exposure concentration, C60–OH and C60–COOH inhibited lysozyme activity more, but C60–COOR and C60 did not alter the enzyme activity. At 10 μg mL−1, the remnant lysozyme activity was 63.8% for C60–OH, 82.9% for C60–COOH, 95.7% for C60–COOR and 91.5% for C60. At 40 μg mL−1, the lysozyme activity was totally inhibited by C60–OH, and the remnant values were 19.1% for C60–COOH, 67% for C60–COOR and 91.5% for C60. Therefore, the enzyme activity loss followed the sequence C60 < C60–COOR < C60–COOH < C60–OH.
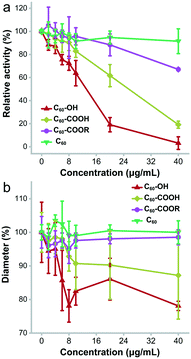 |
| Fig. 9 Antibacterial activity loss of lysozyme upon the incubation with the fullerene samples. (a) Enzymatic activities; (b) diameters of ZOI. | |
The direct consequence of lysozyme activity loss was the inhibition of antibacterial activity (Fig. 9b). According to the Kirby–Bauer test, C60 did not affect the antibacterial activity of lysozyme, and at 40 μg mL−1 the diameters of the zone of inhibition (ZOI) were the same as those of the control group. C60–COOR did not exhibit significant inhibition of the antibacterial activity either. At 40 μg mL−1, the diameters of ZOI were 98.5% of the control. In contrast, C60–COOH had meaningful inhibition of antibacterial activity. At 40 μg mL−1, the diameters of ZOI were 87.2% of the control. The strongest inhibition was observed in the C60–OH group. At 40 μg mL−1, the diameters of ZOI were 78.1% of the control. It was worthy to note that all fullerene samples had no antibacterial activity (Fig. S2†). This was consistent with the report of Aoshima et al. C60(OH)44 was reported to inhibit the growth of Staphylococcus aureus with a minimum inhibitory concentration (MIC) of 2000 μg mL−1, while pristine C60 had no inhibitory effect.40 Separately, due to the different substrates and more complicated systems, the inhibitory effects in Kirby–Bauer tests were slightly weaker than those in Shugar's assays. Therefore, the antibacterial activity inhibition of lysozyme followed the sequence C60 ≈ C60–COOR < C60–COOH < C60–OH.
Discussion
As aforementioned, surface functionalization obviously regulated the protein–fullerene interactions. The well dispersible fullerenes induced more conformational changes to lysozyme and showed much higher inhibition of the antibacterial activity.
The small size of the fullerene cage allowed the binding of fullerene samples into the active pocket of lysozyme. The binding models in the present study were well consistent with our previous studies where fullerene and its derivatives were docked into the active pocket of lysozyme.16,37 Other docking studies also observed the location of C60 in the active pocket of lysozyme.41,42 More importantly, the 1
:
1 C60@lysozyme adduct was experimentally observed by nuclear magnetic resonance (NMR) to highlight the residues interacting with C60 with a chemical shift in the active pocket of lysozyme.43 Similar results were reported for other proteins. Gieldon et al. found that C60 tended to interact with the active sites of proteins.44 Li et al. docked fullerene derivatives to the interfacial cavity of HSA subdomains.45
The major interaction between fullerene samples and lysozyme was the π–π interaction, while other interactions also contributed to the total binding strength. Wu et al. showed that π–π interaction was the major contributor to the binding strength between fullerene and proteins. H-Bonds were observed in the C60–OH–protein interaction, too.16 Gieldon et al. found that C60–protein interactions included π–π, cation–π and anion–π interactions, where hydrophilic residues were preferred, such as GLU, LYS, ARG, ASN and ASP.44 Huy et al. reported that vdw interaction dominated the amyloid β–fullerene interaction.46 Similarly, Li et al. found that vdw interaction was the most important contributor to fullerene–HSA interaction.45 Ma et al. docked Gd@C82(OH)22 to cytochrome P450 and found the contribution of electrostatic interactions to be dominant.47 Based on our calculations, a high degree of chemical functionalization seriously decreased the π–π interaction between C60–OH and lysozyme, while slightly increased the H-bond interaction, resulting in weaker total binding strength.
However, the experimental results collectively showed that hydrophilic functionalization increased the lysozyme–fullerene interaction, leading to more conformation changes and the loss of antibacterial activity. The conformational changes of proteins upon the incubation with fullerene were widely reported in the literature. Previously, we showed that C60–OH induced the nearly complete loss of the secondary structure of lysozyme.37 Bednarikova et al. found that fullerenol C60(OH)16 bound to amyloid β, changed the conformation, and inhibited the fibrillization.48 Zanzoni et al. reported the binding of C60(OH)24 to ubiquitin and observed the conformational changes in a fullerenol
:
protein ratio-dependent way.49 Yang et al. showed that fullerenol could quench the intrinsic fluorescence and increase the UV absorbance of NSA and γ-globulins.50 Li et al. observed the slight distortion of the HSA structure by C60.51 The enzyme activity loss upon the incubation with functionalized fullerene was consistent with the literature studies, too. Previously, we observed the activity inhibition of lysozyme by C60–OH in a concentration dependent way.37 Kobzar et al. showed the effective inhibition of phosphatases by four fullerene derivatives.52 Therefore, these results collectively indicated that the strong interaction between chemically functionalized fullerene and lysozyme would change the protein conformation and inhibit the enzymatic activity.
The contradictory results from computational and experimental results might be explained from the perspective of the dispersion state of fullerene samples and the complexity of real environments. In the computational evaluations, a single fullerene cage was used for docking to lysozyme for all fullerene samples. Single fullerene was easier for docking and also for dispersibility variation. However, C60 was insoluble in water and precipitated as big aggregates. It was the same for C60–COOR. Thus, it was unlikely to have single-dispersed C60 and C60–COOR in aqueous systems. This was confirmed by the DLS measurements (Fig. 3a and b) that only large aggregates of a small portion were suspended upon sonication. The aggregates were too large for the active pocket of lysozyme, so C60 and C60–COOR could not reach the lysozyme active centre and had a low impact on lysozyme activity (Fig. 9). There have been reports showing that fullerene preferred to form 1
:
1 adducts with lysozyme and BSA.41,53 Upon hydroxylation, the water dispersibility of the fullerene increased and smaller aggregates were detected in DLS. Even single-dispersed C60–OH was found as indicated by the small peak at 1 nm (Fig. 3d). After the binding of C60–OH to lysozyme, more C60–OH would disperse singly due to the shift of dissolution balance. Therefore, C60–OH could move into the active pocket of lysozyme to induce the conformational changes and activity loss, although the binding strength between C60–OH and lysozyme was the lowest among the four complexes. For C60–COOH, the situation was more complicated. C60–COOH was water dispersible, but C60–COOH formed colloids due to its amphiphilic nature, where the fullerene cage was hydrophobic and the –COOH groups were hydrophilic. The disassociation of single C60–COOH was much harder than that of C60–OH. Thus, the influence of C60–COOH was stronger than that of C60 and C60–COOR, but lower than that of C60–OH. These data suggested the importance of the dispersion state in evaluating the biological and environmental effects of NMs. As supplementary evidence of our speculation, Song et al. prepared water soluble C60 by sonicating a C60 toluene solution and observed serious conformational changes of HSA upon the exposure to C60.54 They supposed that the edge of C60 aggregates stuck into the cavity of HSA. In a more recent study, Fu et al. further confirmed that smaller C60 aggregates had stronger binding strength to HSA and BSA.55 The intrinsic fluorescence quenching and absorbance of proteins showed size-dependent trends. For the bigger aggregates of fullerenes, their large size might allow more protein molecules to bind on the aggregate surface to form coronae in realistic exposures to biomolecules.9,10,56 The aforementioned data implied that the direct binding to active sites had more influences on proteins than the formation of more complex coronae. In the future, the protein corona formation, components and impact on fullerenes should be further investigated.9,10
Our results were important for the design of safe NMs and the understanding of the bioactivity and toxicity of NMs. First, the NM surface could be designed and functionalized to regulate the protein–NM interaction. Through this, safer NMs of excellent bioactivities could be obtained. For example, Lu et al. developed a hydroxylation method to regulate the protein interaction manner of graphene.57 Through the serum protein binding, the blood circulation and cytotoxicity of graphene could be controlled. Second, the different effects of functional groups on the protein–NM interaction provide new insights into the nanotoxicity and environmental effects. For instance, we found that C60–COOH strongly inhibited the decomposition activity of white rot fungi, where C60–COOH bound intensely to laccase.28 Third, the results highlighted the importance of the dispersion state of NMs in the protein–NM interaction and toxicity. In our evaluations of carbon NMs on plants, we evidenced that well-dispersed carbon NMs were more toxic than non-dispersible ones.58 Silver nanoparticles and TiO2 nanoparticles also demonstrated the aggregation regulated toxicity in different evaluations.59,60 The aggregation changed the protein binding and corona formation, which consequently influenced the uptake and toxicity of these NMs. Overall, in future studies, the surface chemistry regulated protein–NM interactions should be carefully considered in the design, application and safety evaluation of NMs.
Conclusions
In summary, we adopted fullerene as a representative model to investigate the influences of surface modification on protein–carbon nanomaterial interactions by both computational and experimental approaches. Molecular docking results suggested that surface modification on the carbon cage decreased the protein–fullerene interactions due to the breakage of aromatic domains of the fullerene cage. In contrast, the protein conformational investigations indicated that functionalization with hydrophilic groups increased the contact between fullerene and lysozyme, which consequently led to more conformational changes and serious antibacterial activity inhibition. Our results collectively suggested that surface modification of carbon NMs with hydrophilic moieties increased their water dispersibility, making fullerene more accessible to the protein molecules. The dispersion state was the dominant influencing factor in the protein–nanomaterial interactions rather than the decreased π–π interaction. Our results provided good explanations of the contradiction between the computational and experimental results of the protein–nanomaterial interactions. It is believed that our results would benefit the understanding of biological/environmental effects of NMs at the protein level and the design of safer carbon NMs by suitable surface functionalization.
Experimental
Preparation of fullerene samples
The preparation of fullerene samples was performed following our previous protocols.26,27 Briefly, C60 was obtained from Nanjing XFNANO Materials Tech Co., Ltd. C60 was dissolved in toluene. Me2HNCH2COOEt·HCl (279 mg, 2.0 mmol) and NaOH (228 mg, 5.7 mmol) were added to 15 mL of methanol. The mixture solution was added to the C60 toluene solution (1.2 mg mL−1, 60 mL) in a flask. The mixture was stirred for 1 h under the irradiation of a xenon lamp (300 W). The solution colour changed from purple to brown. The solution was added to water, filtered and freeze-dried to give C60–COOR. Sodium hydride (1 g) was added to the concentrated solution of C60–COOR. The mixture was stirred continuously at 70 °C for 6 h and then added to methanol (10 mL) slowly. The solvent was evaporated and water (50 mL) was added to dissolve C60–COOH. The suspension was centrifuged at 3000 rpm for 5 min to remove the insoluble precipitates. A Sephadex G-25 gel filled column was applied to separate C60–COOH from the small molecules, e.g. NaOH. The first fraction was collected and lyophilized to obtain C60–COOH. Separately, 80 mg of C60 was dissolved in benzene (60 mL). KOH aqueous solution (10 mL, 1.0 g mL−1) and 0.2 mL of tetra-n-butylammonium hydroxide were added to the solution and stirred for several minutes. After the solution colour faded, benzene was removed by vacuum distillation. The mixture was stirred for another 60 h. After the addition of 20 mL of water, the mixture was stirred for 12 h and diluted with water again. After the filtration to remove the insoluble precipitates, C60–OH was precipitated with methanol. The precipitate was solubilized in water and separated using the Sephadex G-25 gel filled column. The first fraction was collected and lyophilized to obtain C60–OH.
The obtained fullerene samples were characterized using a TEM (Tecnai G2 20, FEI, USA), SEM (JSM-7500, JEOL, Tokyo, Japan), XPS (ESCALAB 250XI, Thermo-Fisher, USA), IR (Nicolet Avatar 370, Thermo, Madison, WI, USA), and UV-vis spectrometer (UV1800, Mapada, Shanghai, China) and a nanosizer (Nanobrook-Omni, Bruker, Holtsville, USA).
AutoDocking
Fullerene C60 was built from the molecular template in the CS Chem3D Ultra system and the surface modifications were constructed using CS Chem3D Ultra following our previous protocol.28 Lysozyme (PDB code: 1HEL) was obtained from the protein databank at the official website http://www.rcsb.org/. The AutoDock 4.2 software was downloaded from the official website http://AutoDock.scripps.edu/ for docking experiments. The fullerene samples and lysozyme were prepared using AutoDock Tools 1.5.1, including extracting the other molecules and water from the protein PDB file, adding all hydrogen atoms, merging non-polar hydrogen, and setting all the bonds in functional groups rotatable and the fullerene cage rigid. The ligand and receptor charges were assigned automatically. To build the binding model and obtain the binding energy, three rounds of docking were performed. Firstly, “blind docking” was performed with a large grid volume covering the entire surface of lysozyme to find the preferable binding regions (10 sites). Then, the second round docking with a smaller fine box only covering the binding site was performed to evaluate the possible binding sites from “blind docking”. Finally, flexible docking was carried out to build the optimal binding models. The binding energy and the association constant were calculated from the third round docking.
Protein conformation investigations
A lysozyme solution (50 μg mL−1) was prepared by dissolving lysozyme powder (lysozyme from hen egg white, Sigma Co., St Louis, USA) in 40 mM phosphate buffer (pH 6.2). For the conformational change investigation, lysozyme was incubated with the fullerene samples at different concentrations for 12 h before measurements. The UV-vis absorption spectra were scanned from 200 to 500 nm on a UV-vis spectrometer (UV1800, Mapada, Shanghai, China). The absorbance of the fullerene samples at corresponding concentrations was subtracted to avoid the interference of fullerene samples. The highest concentration of 40 μg mL−1 was not used because the absorbance of the fullerene samples was too high and would interfere with the measurements. The far-UV CD spectra were recorded on a CD spectrometer (MOS-500, Bio-Logic Science Instruments Co., Seyssinet-Pariset, France) from 250 to 200 nm. For intrinsic fluorescence, the samples were excited at 280 and 295 nm independently, and the emissions were recorded at the range of 310–470 nm (Cary Eclipse PCB150, Varian Medical Systems Co., Palo Alto, USA). The ΔF and KD values were calculated following the literature protocol. The highest concentration of 40 μg mL−1 was not used, because the absorbance of the fullerene samples was too high and would quench the fluorescence.
Enzymatic and antibacterial activity measurements
For enzymatic activity measurements, lysozyme was incubated with different concentrations of the fullerene samples for 12 h at room temperature. The enzyme activity of lysozyme was determined following Shugar's method. Briefly, 1.0 mL of Micrococcus lysodeikticus (Sigma Co., St Louis, USA) was added into a quartz cuvette at 0.25 mg mL−1 and the absorbance was recorded at 450 nm. Then, the lysozyme solution in the presence/absence of fullerene samples (200 μL) was added to the cuvette and the dynamic absorbance was recorded at 450 nm for 20 s. The initial slope (k) of the dynamic absorbance curve was used for the relative activity calculations.
For antibacterial activity measurements, lysozyme was dissolved in the solutions of fullerene samples. The mixture was added to circular filter paper (diameter of 0.6 cm) and incubated for 12 h at room temperature. The filter paper was placed on tryptic soy agar (TSA) culture medium, which was inoculated with S. aureus (50 μL, 6 × 104 cells per mL).46 The plates were incubated at 37 °C for another 12 h. The diameters of the ZOI were recorded to reflect the antibacterial activities.
Conflicts of interest
There are no conflicts to declare.
Acknowledgements
We thank Prof. Aoneng Cao at Shanghai University for the CD measurements. This work was supported by the National Natural Science Foundation of China (No. 21777132 and 11675189), the Beijing Natural Science Foundation (2202065), the National Key Research and Development Program of China (2016YFA0201603), the National Program for Support of Top-notch Young Professionals, and the Fundamental Research Funds for the Central Universities, Southwest Minzu University (No. 2019NYB17).
Notes and references
- Y. Ding, M. Q. Zeng and L. Fu, Low-temperature synthesis of sp2 carbon nanomaterials, Sci. Bull., 2019, 64, 1817–1829 CrossRef CAS.
- S. Gupta and N.-H. Tai, Carbon materials and their composites for electromagnetic interference shielding effectiveness in X-band, Carbon, 2019, 152, 159–187 CrossRef CAS.
- R. Karthick and F. Chen, Free-standing graphene paper for energy application: Progress and future scenarios, Carbon, 2019, 150, 292–310 CrossRef CAS.
- L. Q. Zhao, S.-T. Yang, Y. L. H. M. Ailimire and D. Y. Wu, Advances in the applications of graphene adsorbents: From water treatment to soil remediation, Rev. Inorg. Chem., 2019, 39, 47–76 CAS.
- G. E. LeCroy, S.-T. Yang, F. Yang, Y. Liu, K. A. S. Fernando, C. E. Bunker, Y. Hu, P. G. Luo and Y.-P. Sun, Functionalized carbon nanoparticles: Syntheses and applications in optical bioimaging and energy conversion, Coord. Chem. Rev., 2016, 320–321, 66–81 CrossRef CAS.
- Z. Peng, X. J. Liu, W. Zhang, Z. T. Zeng, Z. F. Liu, C. Zhang, Y. Liu, B. B. Shao, Q. H. Liang, W. W. Tang and X. Z. Yuan, Advances in the application, toxicity and degradation of carbon nanomaterials in environment: A review, Environ. Int., 2020, 134, 105298 CrossRef CAS.
- P. Laux, C. Riebeling, A. M. Booth, J. D. Brain, J. Brunner, C. Cerrillo, O. Creutzenberg, I. Estrela-Lopis, T. Gebel, G. Johanson, H. Jungnickel, H. Kock, J. Tentschert, A. Tlili, A. Schäffer, A. J. A. M. Sips, R. A. Yokel and A. Luch, Challenges in characterizing the environmental fate and effects of carbon nanotubes and inorganic nanomaterials in aquatic systems, Environ. Sci.: Nano, 2018, 5, 48–63 RSC.
- M. Chen, Y. Sun, J. Liang, G. M. Zeng, Z. W. Li, L. Tang, Y. Zhu, D. N. Jiang and B. Song, Understanding the influence of carbon nanomaterials on microbial communities, Environ. Int., 2019, 126, 690–698 CrossRef CAS.
- Y. L. Wang, R. Cai and C. Y. Chen, The nano–bio interactions of nanomedicines: Understanding the biochemical driving forces and redox reactions, Acc. Chem. Res., 2019, 52, 1507–1518 CrossRef CAS.
- S.-T. Yang, Y. Liu, Y. Wang and A. N. Cao, Biosafety and bioapplication of nanomaterials by designing protein–nanoparticle interactions, Small, 2013, 9, 1635–1653 CrossRef CAS.
- S. Zhu, L. L. Li, Z. J. Gu, C. Y. Chen and Y. L. Zhao, 15 years of small: Research trends in nanosafety, Small, 2020, 2000980 CrossRef CAS.
- M. Barbalinardo, F. Caicci, M. Cavallini and D. Gentili, Protein corona mediated uptake and cytotoxicity of silver nanoparticles in mouse embryonic fibroblast, Small, 2018, 14, 1801219 CrossRef.
- M. Barbalinardo, A. Antosova, M. Gambucci, Z. Bednarikova, C. Albonetti, F. Valle, P. Sassi, L. Latterini, Z. Gazova and E. Bystrenova, Effect of metallic nanoparticles on amyloid fibrils and their influence to neural cell toxicity, Nano Res., 2020, 13, 1081–1089 CrossRef CAS.
- A. J. Chetwynd and I. Lynch, The rise of the nanomaterial metabolite corona, and emergence of the complete corona, Environ. Sci.: Nano, 2020, 7, 1041–1060 RSC.
- H. Meng, W. Leong, K. W. Leong, C. Y. Chen and Y. L. Zhao, Walking the line: The fate of nanomaterials at biological barriers, Biomaterials, 2018, 174, 41–53 CrossRef CAS.
- X. Wu, S.-T. Yang, H. Wang, L. Wang, W. Hu, A. Cao and Y. F. Liu, Influences of the size and hydroxyl number of fullerenes/fullerenols on their interactions with proteins, J. Nanosci. Nanotechnol., 2010, 10, 6298–6304 CAS.
- P. Roy, S. Bag, D. Chakraborty and S. Dasgupta, Exploring the inhibitory and antioxidant effects of fullerene and fullerenol on ribonuclease, ACS Omega, 2018, 3, 12270–12283 CAS.
- H. Yilmaz, L. Ahmed, B. Rasulev and J. Leszczynski, Application of ligand- and receptor-based approaches for prediction of the HIV-RT inhibitory activity of fullerene derivatives, J. Nanopart. Res., 2016, 18, 2–12 Search PubMed.
- Y. T. Bai, Z. Ming, Y. Y. Cao, S. C. Feng, H. Yang, L. Y. Chen and S.-T. Yang, Influence of graphene oxide and reduced graphene oxide on the activity and conformation of lysozyme, Colloids Surf., B, 2017, 154, 96–103 CrossRef CAS.
- X. T. Liu, C. X. Yan and K. L. Chen, Adsorption of human serum albumin on graphene oxide: Implications for protein corona formation and conformation, Environ. Sci. Technol., 2019, 53, 8631–8639 CrossRef CAS.
- D. H. Zhao, L. B. Li and J. Zhou, Simulation insight into the cytochrome c adsorption on graphene and graphene oxide surfaces, Appl. Surf. Sci., 2018, 428, 825–834 CrossRef CAS.
- Z. Z. Wang, X. L. Chang, Z. H. Lu, M. Gu, Y. L. Zhao and X. F. Gao, A precision structural model for fullerenols, Chem. Sci., 2014, 5, 2940–2948 RSC.
- S. S. Babu, H. Mohwald and T. Nakanishi, Recent progress in morphology control of supramolecular fullerene assemblies and its applications, Chem. Soc. Rev., 2010, 39, 4021–4035 RSC.
- L. Becker, J. L. Bada, R. E. Winans and T. E. Bunch, Fullerenes in Allende meteorite, Nature, 1994, 372, 507 CrossRef CAS.
- J. Sanchís, N. Berrojalbiz, G. Caballero, J. Dachs, M. Farré and D. Barceló, Occurrence of aerosol-bound fullerenes in the Mediterranean Sea atmosphere, Environ. Sci. Technol., 2012, 46(3), 1335–1343 CrossRef.
- J. Sanchís, R. Milačič, T. Zuliani, J. Vidmar, E. Abad, M. Farré and D. Barceló, Occurrence of C60 and related fullerenes in the Sava River under different hydrologic conditions, Sci. Total Environ., 2018, 643, 1108–1116 CrossRef.
- J. Wang, T. B. Onasch, X. Ge, S. Collier, Q. Zhang, Y. Sun, H. Yu, M. Chen, A. S. H. Prévo and D. R. Worsnop, Observation of fullerene soot in Eastern China, Environ. Sci. Technol. Lett., 2016, 3, 121–126 CrossRef CAS.
- Z. Ming, S. C. Feng, Y. L. H. M. Ailimire, Q. Ma, S. N. Yang and S.-T. Yang, Toxicity of pristine and chemically functionalized fullerenes to white rot fungus Phanerochaete chrysosporium, Nanomaterials, 2018, 8, 2–15 Search PubMed.
- M. M. Du, H. Zhang, J. X. Li, C. Z. Yan, X. Zhang and X.-L. Chang, Bioaccumulation, depuration, and transfer to offspring of 13C-labeled fullerenols by Daphnia magna, Environ. Sci. Technol., 2016, 50, 10421–10427 CrossRef CAS.
- C. L. Wang, H. Zhang, L. Ruan, L. Chen, H. Li, X.-L. Chang, X. Zhang and S.-T. Yang, Bioaccumulation of 13C-fullerenol nanomaterials in wheat, Environ. Sci.: Nano, 2016, 3, 799–805 RSC.
- M. I. Melnyk, I. V. Ivanova, D. O. Dryn, Y. I. Prylutskyy, V. V. Hurmach, M. Platonov, L. T. Al Kury, U. Ritter, A. I. Soloviev and A. V. Zholos, C60 fullerenes selectively inhibit BKCa but not Kv channels in pulmonary artery smooth muscle cells, Nanomedicine, 2019, 19, 1–11 CrossRef CAS.
- A. Dabrowska, T. Pieńko, P. Taciak, K. Wiktorska, Z. Chilmonczyk, A. P. Mazurek and A. Stasiulewicz, Fullerene derivatives of nucleoside HIV reverse transcriptase inhibitors-in silico activity prediction, Int. J. Mol. Sci., 2018, 19, 3231 CrossRef.
- O. Acton, T. Grant, G. Nicastro, N. J. Ball, D. C. Goldstone, L. E. Robertson, K. Sader, A. Nans, A. Ramos, J. P. Stoye, I. A. Taylor and P. B. Rosenthal, Structural basis for fullerene geometry in a human endogenous retrovirus capsid, Nat. Commun., 2019, 10, 1–13 CrossRef.
- C. L. Wang, Y. T. Bai, H. L. Li, R. Liao, J. X. Li, H. Zhang, X. Zhang, S. J. Zhang, S.-T. Yang and X.-L. Chang, Surface modification-mediated biodistribution of 13C-fullerene C60 in vivo, Part. Fibre Toxicol., 2016, 13, 2–14 Search PubMed.
- Y. Zhang, Y. Wan, Y. Chen, N. T. Blum, J. Lin and P. Huang, Ultrasound-enhanced chemo-photodynamic combination therapy by using albumin “Nanoglue”-based nanotheranostics, ACS Nano, 2020, 14, 5560–5569 CrossRef CAS.
- Y. Budama-Kilinc, R. Cakir-Koc, S. Kecel-Gunduz, Y. Kokcu, B. Bicak, H. Mutlu and A. E. Ozel, Novel NAC-loaded poly(lactide-coglycolide acid) nanoparticles for cataract treatment: preparation, characterization, evaluation of structure, cytotoxicity, and molecular docking studies, PeerJ, 2018, 6, e4270 CrossRef.
- S.-T. Yang, H. F. Wang, L. Guo, Y. Gao, Y. F. Liu and A. N. Cao, Interaction of fullerenol with lysozyme investigated by experimental and computational approaches, Nanotechnology, 2008, 19, 395101 CrossRef.
- B. Aneja, M. Kumari, A. Azam, A. Kumar, M. Abid and R. Pate, Effect of triazole-tryptophan hybrid on the conformation stability of bovine serum albumin, Luminescence, 2018, 33, 464–474 CrossRef CAS.
- S. Gorinstein, I. Goshev, S. Moncheva, M. Zemser, M. Weisz, A. Caspi, I. Libman, H. T. Lerner, S. Trakhtenberg and O. Martín-Belloso, Intrinsic tryptophan fluorescence of human serum proteins and related conformational changes, J. Protein Chem., 2000, 19, 637–642 CrossRef CAS.
- H. Aoshima, K. Kokubo, S. Shirakawa, M. Ito, S. Yamana and T. Oshima, Antimicrobial activity of fullerenes and their hydroxylated derivatives, Biocontrol Sci., 2009, 14, 69–72 CrossRef CAS.
- M. Calvaresi, A. Bottoni and F. Zerbetto, Thermodynamics of binding between proteins and carbon nanoparticles: The case of C60@lysozyme, J. Phys. Chem. C, 2015, 119, 28077–28082 CrossRef CAS.
- S. Radic, P. Nedumpully-Govindan, R. Chen, E. Salonen, J. M. Brown, P. C. Ke and F. Ding, Effect of fullerenol surface chemistry on nanoparticle binding-induced protein misfolding, Nanoscale, 2014, 6, 8340–8349 RSC.
- M. Calvaresi, F. Arnesano, S. Bonacchi, A. Bottoni, V. Calò, S. Conte, G. Falini, S. Fermani, M. Losacco, M. Montalti, G. Natile, L. Prodi, F. Sparla and F. Zerbetto, C60@lysozyme: Direct observation by nuclear magnetic resonance of a 1:1 fullerene protein adduct, ACS Nano, 2014, 8, 1871–1877 CrossRef CAS.
- A. Gieldon, M. M. Witt, A. Gajewicz and T. Puzyn, Rapid insight into C60 influence on biological functions of proteins, Struct. Chem., 2017, 28, 1775–1788 CrossRef CAS.
- J. Y. Li, L. Z. Jiang and X. L. Zhu, Solvation of the fluorine containing anions and their lithium salts in propylene carbonate and dimethoxyethane, J. Mol. Model., 2015, 21, 2–9 CrossRef.
- P. D. Q. Huy and M. S. Li, Binding of fullerenes to amyloid beta fibrils: Size matters, Phys. Chem. Chem. Phys., 2014, 16, 20030–20040 RSC.
- D. F. Ma, X. Y. Meng, D. R. Bell, S. T. Liu and R. H. Zhou, Inhibition of CYP2C8 by metallofullerenol Gd@C82(OH)22 through blocking substrate channels and substrate recognition sites, Carbon, 2018, 127, 667–675 CrossRef CAS.
- Z. Bednarikova, P. D. Q. Huy, M.-M. Mocanu, D. Fedunova, M. S. Li and Z. Gazova, Fullerenol C60(OH)16 prevents amyloid fibrillization of Aβ40-in vitro and in silico approach, Phys. Chem. Chem. Phys., 2016, 18, 18855–18867 RSC.
- S. Zanzoni, A. Ceccon, M. Assfalg, R. K. Singh, D. Fushman and M. D'Onofrio, Polyhydroxylated [60] fullerene binds specifically to functional recognition sites on a monomeric and a dimeric ubiquitin, Nanoscale, 2015, 7, 7197–7205 RSC.
- L. Y. Yang, S. Y. Hua, Z. Q. Zhou, G. C. Wang, F. L. Jiang and Y. Liu, Characterization of fullerenol-protein interactions and an extended investigation on cytotoxicity, Colloids Surf., B, 2017, 157, 261–267 CrossRef CAS.
- S. Li, X. C. Zhao, Y. M. Mo, P. T. Cummings and W. T. Heller, Human serum albumin interactions with C60 fullerene studied by spectroscopy, small-angle neutron scattering, and molecular dynamics simulations, J. Nanopart. Res., 2013, 15, 1769 CrossRef.
- O. L. Kobzar, V. V. Trush, V. Y. Tanchuk, A. V. Zhilenkov, P. A. Troshin and A. I. Vovk, Fullerene derivatives as a new class of inhibitors of protein tyrosine phosphatases, Bioorg. Med. Chem. Lett., 2014, 24, 3175–3179 CrossRef CAS.
- B. Belgorodsky, L. Fadeev, J. Kolsenik and M. Gozin, Formation of a soluble stable complex between pristine C60-fullerene and a native blood protein, ChemBioChem, 2006, 7, 1783–1789 CrossRef CAS.
- M. Y. Song, S. F. Liu, J. F. Yin and H. L. Wang, Interaction of human serum album and C60 aggregates in solution, Int. J. Mol. Sci., 2011, 12, 4964–4974 CrossRef CAS.
- X. F. Fu, Y. L. Fang, H. L. Zhao and S. F. Liu, Size-dependent binding of pristine fullerene (nC60) nanoparticles to bovine/human serum albumin, J. Mol. Struct., 2018, 1166, 442–447 CrossRef CAS.
- W. Richtering, I. Alberg and R. Zentel, Nanoparticles in the biological context: Surface morphology and protein corona formation, Small, 2020, 2002162 CrossRef CAS.
- X. Lu, P. P. Xu, H. M. Ding, Y. S. Yu, D. Huo and Y. Q. Ma, Tailoring the component of protein corona via simple chemistry, Nat. Commun., 2019, 10, 4520 CrossRef.
- L. Y. Chen, C. L. Wang, S. N. Yang, X. Guan, Q. Q. Zhang, M. Y. Shi, S.-T. Yang, C. Chen and X.-L. Chang, Chemical reduction of graphene enhances in vivo translocation and photosynthetic inhibition in pea plants, Environ. Sci.: Nano, 2019, 6, 1077–1088 RSC.
- M. Smékalová, A. Panácek, D. Jancula, B. Marsálek, J. Kolarík, R. Pruceka, L. Kvítek and R. Zboril, Culture medium mediated aggregation and re-crystallization of silver nanoparticles reduce their toxicity, Appl. Mater. Today, 2018, 12, 198–206 CrossRef.
- J. Hu, J. Wang, S. Liu, Z. Zhang, H. Zhang, X. Cai, J. Pan and J. Liu, Effect of TiO2 nanoparticle aggregation on marine microalgae Isochrysis galbana, J. Environ. Sci., 2018, 66, 208–215 CrossRef.
Footnote |
† Electronic supplementary information (ESI) available: IR spectra of fullerene samples, and low antibacterial activity of fullerene samples. See DOI: 10.1039/d0en00645a |
|
This journal is © The Royal Society of Chemistry 2021 |