Vanadium tetrasulfide cross-linking graphene-like carbon driving a sustainable electron supply chain from pollutants through the activation of dissolved oxygen and hydrogen peroxide†
Received
28th September 2020
, Accepted 23rd November 2020
First published on 17th December 2020
Abstract
Excessive energy consumption and low reaction efficiency caused by electron cycle rate limitations are bottlenecks in water treatment. Here, we introduce an innovative strategy to overcome this problem via constructing a novel three-dimensional (3D) hybrid of vanadium tetrasulfide cross-linking graphene-like carbon with π electrons (VSO–C(π)), which exhibits excellent performance during refractory pollutant removal based on sustainable electron cycling between hydrogen peroxide (H2O2), dissolved oxygen (DO), and pollutants at the solid–liquid micro-interface. VSO–C(π) is synthesized through an in situ hydrothermal synthesis procedure and characterized via a series of techniques. The cation-π structures are constructed through V–S–C(π) and V–O–C(π) bridges in VSO–C(π), triggering orientable electron transfer from C(π) to the metal V centers and forming a polarized distribution of surface electrons. In the VSO–C(π)/pollutants/DO/H2O2 system, the pollutants act as electron donors to C(π), with the subsequent degradation of pollutants, while DO and H2O2 act as electron acceptors and are activated by reactive oxygen species to further degrade the pollutants at the V centers. This sustainable electron cycling process is responsible for the excellent activity and superior adaptability to pH changes and different salt environments, while also greatly saving resources and reducing energy consumption.
Environmental significance
Advanced oxidation processes (AOPs) for water treatment typically require excessive oxidants and additional energy to maintain the electron cycle. Herein, we activate the energy of pollutants and dissolved oxygen (DO)/H2O2 for water treatment via constructing a novel three-dimensional (3D) hybrid consisting of nano-vanadium tetrasulfide cross-linking graphene-like carbon with π electrons (VSO–C(π)). The catalyst shows superior adaptability to pH changes and different salt environments, and the reaction rate was two orders of magnitude higher than that of a traditional Fenton nano-catalyst, greatly saving resources and energy.
|
Introduction
High consumption and low efficiency have been a serious problem in advanced oxidation processes (AOPs) for water treatment, which are mainly caused by the ineluctable electron cycle rate-limiting step during the redox process, which must consume too much oxidant and additional energy to maintain electron cycling.1 This problem particularly exists in the Fenton reaction, although it has been widely used in wastewater treatment.2 The classical Fenton process consists of ferrous ion (Fe2+)/hydrogen peroxide (H2O2), which reacts to generate hydroxyl radicals (˙OH) and superoxide radicals (O2˙−) under acidic conditions (pH 2–3.5) and attacks the recalcitrant organic contaminants.3–5 In this process, a serious bottleneck for application is the rate-limiting step, which involves the reduction of Fe3+ to Fe2+, deposits iron sludge, and excessively consumes iron salts and H2O2.5,6
In recent years, the development of heterogeneous Fenton/Fenton-like technologies has largely solved the problems of iron sludge production, improved the recycling performance, and broadened the pH response range of the Fenton process through various effective channels.7–17 Most of the developed technologies follow the primary reaction mechanism of the classical Fenton process, which means it is still difficult to break through the bottleneck. This process relies on H2O2 as the electron donor and electron acceptor and often requires external energy to drive this redox reaction, which results in the high consumption and low efficiency of H2O2. In fact, in the wastewater treatment process, most pollutants are rich in electrons, especially the aromatic and phenolic pollutants, which are promising electron donors. Meanwhile, wastewater is also rich in dissolved oxygen (DO), which is a natural oxidant and electron acceptor. Unfortunately, these potential electron donors (pollutants) and acceptors (DO) have not been effectively utilized, but instead have been identified as targets to be removed, which is a waste of resources and energy. The effective use of these substances is the key to solving the bottleneck problems.
In our previous research on Fenton/Fenton-like processes,18–24 we found that in addition to the efficient activation of H2O2 and peroxymonosulfate (PMS), the reduction of DO or the oxidative degradation of pollutants on the catalyst surface can be directly driven by constructing surface dual-reaction-centers (DRCs) with electron-poor/-rich microareas on the catalyst. Increasingly researchers have also found that DO can be directly reduced to O2˙− by obtaining electrons from the electron-rich centers on some polarized surfaces of the DRC catalysts before/during the Fenton reaction.25–27 These studies imply that the use of pollutants as electron donors and DO as electron acceptors can be realized by modulating the catalyst surface structure and polarizing the distribution of electrons, which is a promising method to achieve low consumption and high efficiency in AOPs. However, the largest challenges at present are the construction of the substrate structure with electron transmission and flexible control performance and the enhancement of the surface polarization centers to drive sustainable cycling of the directional electron transmission chain during the interfacial reaction.
Vanadium tetrasulfide (VS4) is a conductive linear-chain compound with neighboring V4+(S22−)2 chains bonded by van der Waals forces, which can facilitate fast charge-transfer kinetics and has been reported to be a favorable intercalation cathode material for high-performance batteries.28,29 This type of material with excellent electrochemical properties can likely control the surface electron polarization distribution. Herein, we present for the first time a novel three-dimensional (3D) hybrid water treatment material consisting of VS4 cross-linking graphene-like carbon with π electrons (VSO–C(π)) through an in situ hydrothermal synthesis procedure. The key structural characterization and electron distribution information are revealed and demonstrated by scanning electron microscopy (SEM), high-resolution transmission electron microscopy (HRTEM), X-ray powder diffraction (XRD), confocal laser Raman microspectroscopy (CLRM), X-ray photoelectron spectroscopy (XPS) and electron paramagnetic resonance (EPR) techniques. We find that VSO–C(π) can greatly drive the sustainable electron cycling of pollutants–O2/H2O2, which results in excellent pollutant removal performance. The use of pollutants as electron donors and DO as electron acceptors has been realized in VSO–C(π)/DO/H2O2 systems. The catalyst also showed superior adaptability to pH changes and different salt environments. The structure activity relationship of VSO–C(π) and interfacial reaction processes among DO, H2O2, pollutants and VSO–C(π) are investigated using Fourier transform infrared spectroscopy (FTIR), Raman and spin-trapping EPR techniques. A new interfacial reaction mechanism for sustainable electron cycling involving pollutants–DO/H2O2 for water treatment is proposed in this work.
Experimental section
Chemicals and reagents
Hydrogen peroxide (H2O2, 30%, w/w), 5-tert-butoxycarbonyl-5-methyl-1-pyrroline-N-oxide (BMPO, 99%), bisphenol A (≥99%), 2-chlorophenol (≥99%), cetyl pyridinium bromide (98%), thioacetamide (99%), rhodamine B (96%), ethylene glycol (≥99%), absolute ethyl alcohol, anhydrous sodium sulfate (Na2SO4), sodium chloride (NaCl), and sodium dihydrogen phosphate (NaH2PO4) were obtained from Adamas Reagent Co. (Shanghai). Ammonium metavanadate (NH4VO3) was purchased from Aladdin Reagent Co. (Shanghai). All reagents in the experiment were of analytical grade.
Catalyst synthesis
VSO–C(π) was synthesized by the hydrothermal method as illustrated in Scheme 1. In a typical composition, cetyl pyridinium bromide (CPB, 0.5 g) was dissolved in 30 mL of deionized water; ammonium metavanadate (NH4VO3, 3 mmol, 0.35 g) was added into the solution, heated to 60 °C and stirred for 20 min. Then, 30 mL of ethylene glycol, in which thioacetamide (TAA, 15 mmol, 1.13 g) was dissolved, was introduced into the above solution. The mixed solution was stirred at 60 °C for 30 min, subsequently placed in a 100 mL high-pressure reactor and heated at 160 °C for 12 h. After cooling, the suspension was removed, centrifuged, and alternately washed with ethanol and deionized water 6 times. Finally, the obtained solid matter was dried at 60 °C for 10 h.
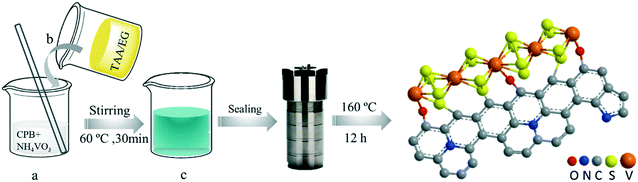 |
| Scheme 1 A schematic illustration of the synthesis process of VSO–C(π). | |
Characterization methods
The powder X-ray diffraction (XRD, PW3040/60) measurements were recorded at room temperature with 1° min−1 over a range (2θ) of 5–80°. Scanning electron microscopy (SEM) and transmission electron microscopy (TEM) images were obtained using a Mira3/MIRA3 (SEM) and a ALOS F200X TEM. XPS was performed on an AXIS Ultra DLD instrument with monochromatic Al Kα radiation and an energy resolution of 0.48 eV/(Ag 3d5/2), 0.68 eV/(C 1s). Fourier transform infrared spectroscopy (FTIR) of the KBr pelleted samples was recorded on a Nicolet iS10 FTIR spectrometer (Thermo Fisher Scientific Inc, USA). The solid samples were subjected to electron paramagnetic resonance (EPR) spectroscopy using an A300-10/12 electron paramagnetic resonance spectrometer (Bruker Biospin Ltd, Switzerland), and the types of free radicals in the different systems were identified. Raman spectra of the samples were collected on an inVia-Reflex confocal microscopic Raman spectrometer (Reinishaw, UK) with 40 mW 532 nm laser light irradiation. The CV curve was measured on an electrochemical workstation (Shanghai Chenhua Instrument Co., Ltd.) with three standard electrodes.
Procedures and analysis
The activity of the prepared catalyst was evaluated by removing some common refractory organic pollutants, including rhodamine B (RhB), bisphenol A (BPA), 2-chlorophenol (2-CP), acid orange 7 (AO7), methylene blue (MB) and ciprofloxacin (CIP). The molecular structures of these typical pollutants are shown in Fig. S1.† The optimal concentrations of the catalyst (0.6 g L−1) and H2O2 (10 mM) were determined based on the best activity for RhB degradation and were used in all experiments unless otherwise specified. In a typical catalytic experiment, 0.03 g of the catalyst powder and 50 μL of H2O2 were added to a beaker containing 50 mL of the pollutant solution (10 mg L−1). The entire solution was magnetically stirred in a water bath at 35 °C. At regular intervals, a sample was taken with a syringe and immediately filtered on a 0.45 μm membrane for further analysis. Dye contaminants were analyzed using an ultraviolet spectrophotometer (752 N), and other colorless contaminants were analyzed using HPLC (Agilent 1260). Detailed method settings are shown in the ESI.† When we tested the material properties after the reaction, the materials were collected by filtering the suspension after the normal reaction process with an organic filtration membrane, washed with deionized water and dried at 60 °C. This method was used to test the stability and recycle ability of the catalysts. Other characterization methods in this paper are shown in the ESI.†
Results and discussion
Catalyst characterization
The XRD pattern of VSO–C(π) is shown in Fig. 1a. Two diffraction peaks at 15.8° and 17.1° were clearly observed, which were attributed to the (011) and (020) diffractions of VS4 (PDF card no: 21-1434). The peaks at 36.3°, 40.7° and 44.2° corresponded to the (−402), (−422) and (042) crystal planes of VS4, respectively, which indicates that the main component of VSO–C(π) was VS4.30,31Fig. 1b presents the Raman spectrum of VSO–C(π). Two characteristic peaks at 1439.1 cm−1 and 1549.8 cm−1 belonged to the D and G bands of graphene-like carbon.32,33 The D band corresponded to defects or distortion of the crystal lattice carbon atoms. The G band corresponded to the active E2g vibration of sp2-hybridized carbon atoms,34 which confirms that a graphene-like structure was formed in VSO–C(π).
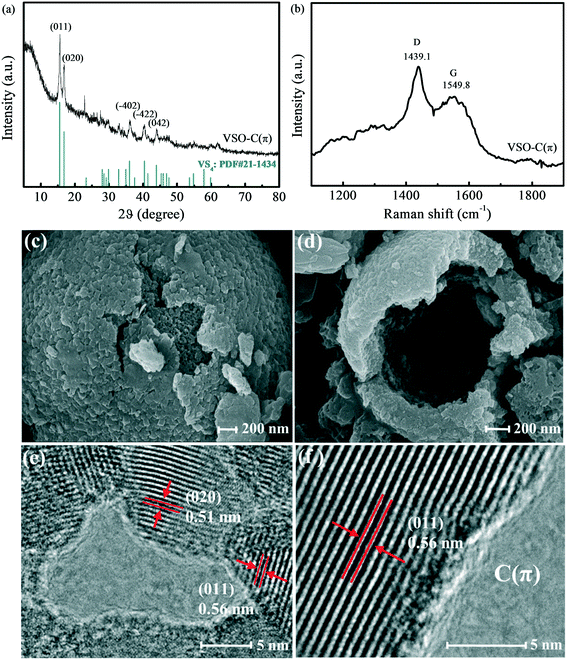 |
| Fig. 1 The (a) XRD pattern and (b) Raman spectrum of VSO–C(π). (c) and (d) SEM images of VSO–C(π). (e) and (f) HRTEM images of VSO–C(π). | |
Microscopy techniques were used to characterize the morphology of VSO–C(π). Fig. 1c and d show the SEM images, where VSO–C(π) was presented as a hollow sphere with a diameter of 1–3 μm. The surface of the sphere was loose and fragile, so there were many fragmentary lumps besides the small spheres in the images. HRTEM images are shown in Fig. 1e and f. Many lattice stripes in different directions were clearly observed in the high-resolution spectrum. The distinct lattice stripes with spacings of 0.56 nm and 0.51 nm were attributed to the (011) and (020) planes of VS4, which are consistent with the XRD patterns. The small piece without stripes in the middle was the carbon substrate, which formed in the synthesis, and the (011) and (020) surfaces of VS4 were closely embedded with the carbon substrate, which indicates that VS4 was cross-linking with graphene-like carbon.
To further confirm the chemical composition and valence state of the obtained product, an XPS technique was performed. The survey spectrum in Fig. S2† shows that VSO–C(π) contained C, O, S, V and a small amount of N elements. High-resolution spectroscopy was also performed to clearly analyze the valence distribution of the elements. In the vanadium spectrum (Fig. 2a), there were four peaks in the spectrum. Two peaks with binding energies of 514.05 and 521.73 were V4+ of 2p3/2 and 2p1/2.35,36 The other two peaks at 517.24 eV and 524.41 eV were from V5+ of 2p3/2 and 2p1/2,37,38 which indicates that VSO–C(π) contained both V4+ and V5+. The S 2p (Fig. 2b) peaks fitting analysis shows the presence of sulfide (S22−),31,39–41 and the peaks at 162.93 and 164.10 eV were assigned to S 2p3/2 and S 2p1/2 of S22−, which verifies the formation of V4+(S22−)2. In O 1s (Fig. 2c), V–O was found at 530.25, explaining that the existence of V5+ is due to the formation of the V–O bond.42,43 The peak at 531.43 eV was attributed to the C–O species, which confirms the formation of C–O–V bond bridges.44 The C 1s XPS spectrum of VSO–C(π) in Fig. 2d displays two fitted peaks. The peak at 284.74 eV corresponded to graphitic carbon (sp2 C–C bonds).22 The peak at 285.73 eV was largely attributed to the C–S and C–O bonds.21 The C–S bond was formed by the potential chemical interaction between VS4 and carbon during the hydrothermal process, which further clarifies that the cross-linking between VS4 and graphene-like carbon was achieved by C–S–V bond bridges. The N 1s spectra (Fig. 2e) fitted into two peaks at 400.24 and 401.81, which were ascribed to pyrrolic-N and graphitic-N, respectively, and it was mainly in the form of graphitic-N. Based on the above characterization results, we confirmed that the C(π)–O–V bond and C(π)–S–V bond were formed in VSO–C(π).
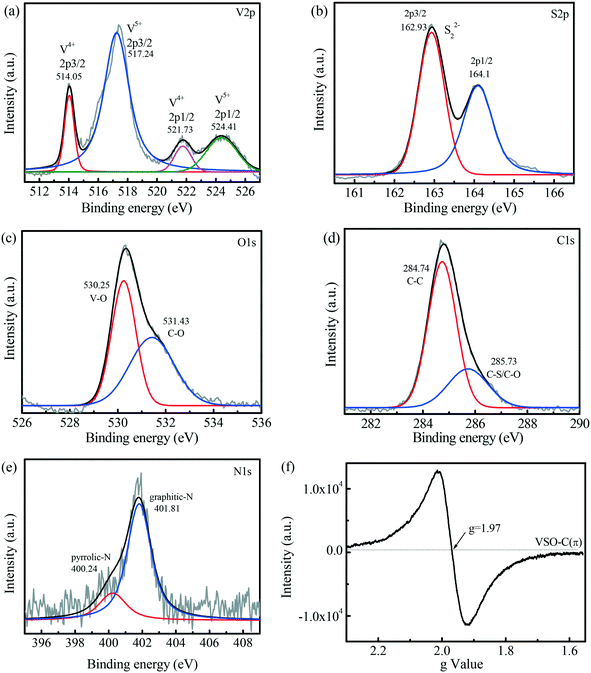 |
| Fig. 2 VSO–C(π) XPS high-resolution scans: (a) V 2p, (b) S 2p, (c) O 1s, (d) C 1s, and (e) N 1s. (f) The solid EPR spectrum of VSO–C(π). | |
Electron paramagnetic resonance (EPR) spectroscopy was used to analyze the electron information in the catalyst. As depicted in Fig. 2f, VSO–C(π) exhibited an obviously symmetric EPR signal at g = 1.97, which was very close to the ge value of the free electrons (∼2.0023).27 The previous characterization revealed the microstructure of VSO–C(π), which had a graphene-like skeleton structure and cross-linking V and C(π) rings through V–S–C(π) and V–O–C(π) bridges, forming a cation-π structure. Since O (3.544) and S (2.58) had a greater electronegativity than C (2.55), the electrons were activated and transferred from C(π) to the metal V center (π → M), which formed the polarized dual reaction microcenters (reinforced electron-rich V microcenters and electron-poor C(π) microcenters), so many free electrons were detected by EPR technology.
H2O2 enhanced the performance of VSO–C(π)
The catalytic activity of VSO–C(π) was evaluated by degrading RhB at natural pH. In the suspension with VSO–C(π)/DO/RhB without H2O2 (Fig. 3a), the performance of VSO–C(π) was very significant, the removal rate of RhB was 90% within 15 min and continued to increase over time to 96.3% at 120 min. This catalyst was far better than the conventional Fe-based Fenton catalyst, whose removal rate was only approximately 10% in 120 min. To reveal whether RhB was simply adsorbed on the surface of VSO–C(π), the VSO–C(π) reacted with RhB after 120 min was measured by UV diffuse reflectance spectroscopy (Fig. S3†). However, no absorption peak of RhB was detected in VSO–C(π), which proves that RhB was eventually degraded rather than only absorbed. Subsequently, HPLC-MS technology (Fig. S4†) was used to analyze the substances in the VSO–C(π)/DO/RhB system after reaction for 5 min. The intermediate products of RhB with different charge mass ratio were detected, strongly demonstrating that degradation was occurring all the time. To further explore the influencing factors of degradation, RhB was degraded by VSO–C(π) in two systems filled with O2 and N2, respectively (Fig. S5†). In the N2 system, the removal rate of RhB at 90 min was only 62.8%, while in the O2 system, it was 97.4%. Obviously, the removal of RhB in the N2 system was inhibited, but it was faster in the O2-filled system than in the normal air system, which indicates that the dissolved oxygen (DO) in water facilitated the reaction. The repeated activities of VSO–C(π) were tested by filtration, washing and drying after the reaction. However, as shown in Fig. 3b, with the increase in the number of repetitions, the degradation rate gradually decreased. Since the formation of the polarized dual reaction microcenters was demonstrated above, RhB in the suspension system was adsorbed in the electron-poor C(π) microcenter of VSO–C(π), where electrons were captured by the π–π interaction and transferred to the electron-rich V microcenters, which results in the degradation of the RhB. Theoretically, the electron-rich V microcenters are further strengthened by the electrons transferred from the pollutant and are more likely to activate DO to generate superoxide radicals. However, due to the low concentration of DO in the system, the pollutants donated electrons much faster than DO obtained electrons. When the number of cycles increased, electrons in VSO–C(π) gradually approached saturation, so the removal rate of pollutants gradually decreased. To drive the sustainable cycling of the directional electron transmission chain during the interfacial reaction, H2O2, which has redox properties, was selected to act as the electron acceptor with DO.
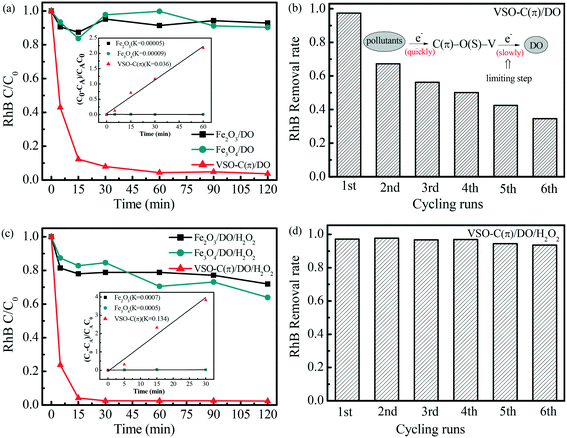 |
| Fig. 3 (a) The removal of RhB in different suspensions without H2O2. (b) The recycling performance for the removal of RhB in VSO–C(π) suspension. (c) The degradation of RhB in different suspensions with H2O2. (d) The recycling performance for the degradation of RhB in VSO–C(π) suspension with H2O2. (The insets in (a) and (c) show the pseudo-second-order kinetics fitting of the degradation curves). Reaction conditions: natural initial pH, initial pollutant concentration: 10 mg L−1, initial H2O2 concentration: 10 mM, initial catalyst concentration: 0.6 g L−1, no new catalyst was added in the cycling experiments. | |
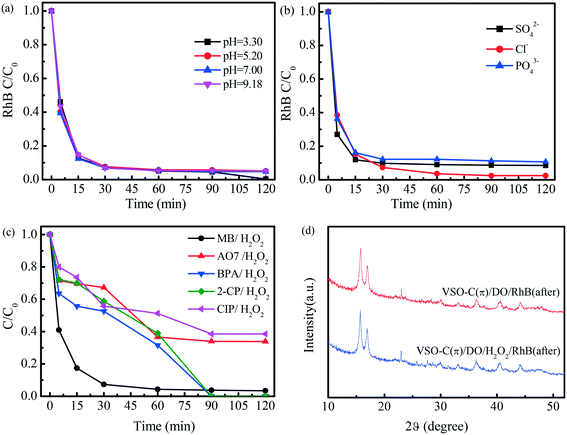 |
| Fig. 4 (a) The effect of initial pH value on RhB degradation in a VSO–C(π) suspension with H2O2. (b) RhB degradation using VSO–C(π) with H2O2 in the presence of different anions. (c) The degradation curves of different pollutants in VSO–C(π) suspensions with H2O2. (d) The XRD patterns of VSO–C(π) obtained after degrading RhB in systems with/without H2O2. Reaction conditions: natural initial pH (except for Fig. 4a), anion concentration: 0.1 M (in Fig. 4b), initial pollutant concentration: 10 mg L−1, initial H2O2 concentration: 10 mM, and catalyst concentration: 0.6 g L−1. | |
In the presence of H2O2 (Fig. 3c), the degradation of RhB by VSO–C(π) was improved: the degradation rate was 97.6% in 15 min and the reaction rate was 3.7 times that of the system without H2O2 and more than 100 times that of the conventional Fe-based Fenton catalyst. Astonishingly, the recycling performance for the degradation of RhB in the VSO–C(π) suspension with H2O2 maintained a high degradation efficiency, and the degradation rate remained above 90% after 6 repetitions (Fig. 3d), which shows that H2O2 played an important role in driving the circulation of the catalytic reaction. In this system, H2O2 and DO together acted as electron acceptors, accelerated the electron transfer of the metal V microcenters and maintained a high degradation rate in the cyclic reaction.
Fig. 4a shows the activity curves with different initial pH values in the VSO–C(π)/DO/H2O2 systems. Over a wide pH range (3.30–9.18), the degradation of RhB by VSO–C(π) was almost unchanged. Solution pH values were generally considered to be sensitive to the reactivity of metal-containing catalysts because the dissociation of H2O produces surface –OH groups in the solution,23 while the formation of the electron-poor/-rich reaction microcenter in VSO–C(π) avoided this effect and demonstrated a widely applicable pH range. In Fig. 4b, 1 M NaSO4, NaCl and NaH2PO4 were added to the VSO–C(π)/DO/H2O2 system to explore the RhB degradation effect in different saline solutions. The results show that VSO–C(π) had a high degradation effect on pollutants, among which Cl− had the least influence on the reaction: the degradation rate was 98% at 120 min, and the degradation rates of the other two were 90%, which reveals the strong adaptability of VSO–C(π). The degradation effects of different pollutants, including dyes MB and AO7, endocrine disruptor BPA, pesticide 2-CP and drug CIP, were also investigated in the VSO–C(π)/DO/H2O2 system. As shown in Fig. 4c, VSO–C(π) had a fast degradation rate of MB, which was 97% in 30 min. MB was very difficult to degrade in the conventional Fenton reaction, which suggests that it was rapidly degraded by the electron-poor C(π) microcenter instead of ˙OH produced by H2O2. BPA and 2-CP were completely degraded in 90 min. The degradation of CIP, which is a refractory antibiotic, and AO7, which is the most-difficult-to-degrade azo dye in printing and dyeing wastewater, reached 60% in 120 min. Fig. 4d shows the XRD pattern of VSO–C(π) after degrading RhB in systems with/without H2O2. The peak positions of the samples did not change compared with fresh VSO–C(π), which suggests that the lattice structures of the materials were not damaged after the reaction. The above results show that VSO–C(π) had broad universality, strong adaptability and good stability.
The interfacial reaction mechanism of pollutants/DO/H2O2 at different sites of VSO–C(π)
Fig. 5a shows the FTIR spectra of the fresh VSO–C(π) and the sample after degrading RhB for 30 min in systems with/without H2O2. The spectra of fresh VSO–C(π) displayed a wide absorption band at 3442 cm−1, which was ascribed to the stretching vibrations of OH [v(OH)] adsorbed on the surface of VSO–C(π). Generally, [v(OH)] can be greatly affected by the complexation of organic substances on the electron-rich species (such as metal species and O site) of the catalyst, with often originated from the deprotonation of the phenolic OH group via σ bonding to the lone pairs of the oxygen atom, leading to the red-shift of the wavenumber.45,46 However, the [v(OH)] band was shifted to 3454 cm−1 after degrading RhB without H2O2 and continuously blue shifted to 3481 cm−1 after the reaction with H2O2, which indicates that the electron-rich pollutants did not contact with metal V sites during the entire process, but were mainly adsorbed in the electron-poor C(π) microcenter of VSO–C(π). As clearly demonstrated in the Raman spectra (Fig. 5b), after degrading RhB, the D and G bands of the graphene-like structures in VSO–C(π) were significantly weakened compared with the fresh VSO–C(π) in Fig. 1b, which indicates that the pollutants were indeed adsorbed on the surface of graphene-like carbon in VSO–C(π), covered the characteristic peaks of the graphene-like structures, and eventually lost electrons to the electron-poor C(π) microcenter of VSO–C(π) through the π–π interaction.
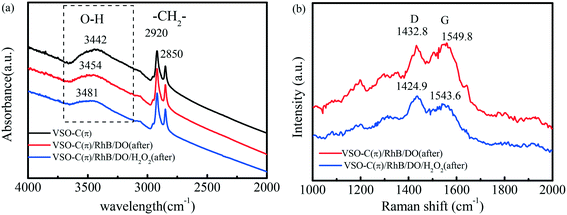 |
| Fig. 5 (a) FTIR spectra and (b) Raman spectra of VSO–C(π) obtained under different conditions, including a fresh VSO–C(π) sample and samples after degrading RhB for 30 min in systems with/without H2O2. | |
The interaction processes among VSO–C(π), H2O2 and pollutants were observed by in situ Raman spectroscopy (Fig. S6†). The characteristic peak at 870.89 cm−1 belonged to the expansion of the O–O bond of H2O2. After VSO–C(π) was added, the adsorption peak of H2O2 shifted to 867.53 cm−1, since H2O2 was adsorbed to the metal V center and formed a peroxide complex with the metal.47 Thus, H2O2 was adsorbed onto metal V microcenters, captured electrons in the electron-rich V microcenters and reduced to ˙OH throughout the reaction, which resulted in the high degradation efficiency of pollutants.
Fig. 6a shows the EPR spectroscopy of the sample after the reaction. The EPR signal intensity of the sample that degraded RhB without H2O2 was approximately 30 times higher than that of the fresh VSO–C(π) (Fig. 2f). This result verifies that the electron-rich contaminant directly interacted with the graphene-like structure of VSO–C(π) though π–π stacking, acted as an electron donor, provided many free electrons to VSO–C(π), and subsequently transferred from the π-electron reservoir of the graphene-like structure to the V center via C(π)–O–V and C(π)–S–V bond bridges. However, DO only slowly received electrons in the V microcenters so the electrons were stored in VSO–C(π); thus, the EPR signal was significantly enhanced. In the presence of H2O2, H2O2 and DO acted as electron acceptors in the system, captured electrons in the metal V center, accelerated the electron transfer, decreased the EPR signal compared with the system without H2O2, and ultimately maintained the continuous and efficient degradation of the cyclic reaction. This result reveals the difference in cycle performance for the degradation of RhB in the VSO–C(π) suspension with/without H2O2.
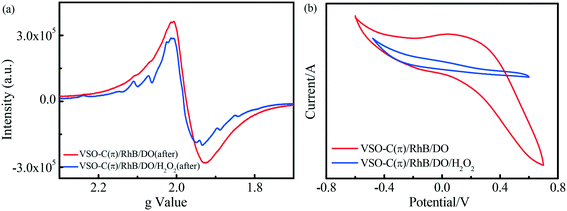 |
| Fig. 6 (a) Solid EPR spectra of VSO–C(π) obtained after degrading RhB for 30 min in systems with/without H2O2. (b) CV curves for VSO–C(π) in electrolyte solution with/without H2O2 and pollutants. | |
Fig. 6b shows the CV data of the electrodes prepared by VSO–C(π) in the electrolyte solutions with H2O2 and pollutants. In general, a narrower CV ring implies a faster electron transfer rate. The CV ring significantly shrank with the addition of H2O2, which suggests that H2O2 accelerated the electron transfer rate of the system.
The production of reactive oxygen species (ROS) in the VSO–C(π) suspension was detected by the EPR spin-trapping technique. Using 5-tertbutoxycarbonyl-5-methyl-1-pyrroline-N-oxide (BMPO) as a trapping agent, the ˙OH species were detected in water media, and the HO2˙/O2˙− species were detected in methanolic media. As shown in Fig. 7a, six continuous peaks corresponding to BMPO–HO2˙/O2˙− were observed in the VSO–C(π) suspension without H2O2 and pollutants, which strongly confirms that DO in the suspension was efficiently reduced in the electron-rich V microcenters of VSO–C(π). Meanwhile, there were four weak peaks with the strength ratio of 1
:
2
:
2
:
1 attributed to ˙OH in the pure catalyst-water suspension (Fig. 7b), which shows that a small amount of ˙OH was produced by H2O losing electrons at the C(π) microcenter.48 After RhB was added into the suspension, the HO2˙/O2˙− signal and ˙OH signal did not significantly decrease, which indicates that the degradation of the pollutant relies mainly on the action of the dual reaction center, with free radical attack being secondary.
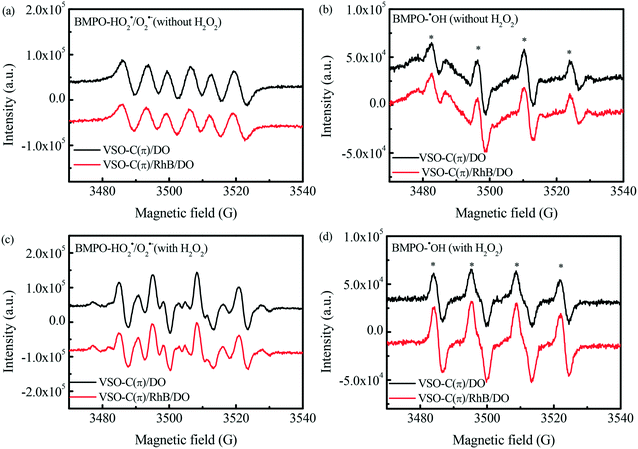 |
| Fig. 7 BMPO spin-trapping EPR spectra for (a) HO2˙/O2˙− and (b) ˙OH in VSO–C(π) aqueous suspensions with/without pollutants; and (c) HO2˙/O2˙− and (d) ˙OH in VSO–C(π) aqueous suspensions in the presence of H2O2 with/without pollutants. | |
On this basis, the interfacial electric transfer process of the VSO–C(π)/DO/H2O2 system was further studied. In the presence of H2O2 (Fig. 7c), the HO2˙/O2˙− signal was significantly enhanced in the VSO–C(π)/DO/H2O2 system due to the oxidation of H2O2 as a nucleophilic reagent in the C(π) microcenter and the reduction of DO in the electron-rich V microcenters. Similarly, the signal strength of ˙OH increased compared to that in the system without H2O2 (Fig. 7d). This phenomenon indicates that H2O2 was oxidized in the C(π) microcenter and effectively reduced to the ˙OH radical in the electron-rich V microcenters of VSO–C(π). After pollutants were added into the suspension, the HO2˙/O2˙− signals slightly weakened, which suggests that the electron-rich pollutants were more likely to replace H2O2 to be adsorbed onto the C(π) microcenter. They played the role of electron donors, prevented H2O2 from the oxidative decomposition to HO2˙/O2˙− and improved the utilization of H2O2. However, the signal intensity of ˙OH radicals did not decrease but significantly increased after RhB was added, which confirms that the electron donor role of pollutants in the C(π) microcenter promoted the reduction of more H2O2 in the V microcenter, and the degradation of pollutants was not mainly dependent on ˙OH.19,23
Based on the above results, a schematic diagram of the interface reaction mechanism in the VSO–C(π)/pollutants/DO/H2O2 system is presented in Fig. 8. The pollutants were oxidized as electron donors at the electron-poor C(π) microcenters, while DO and H2O2 acted as electron acceptors and reduced at the electron-rich V microcenters. This process eventually resulted in two degradation pathways of the pollutants: (i) oxidized as an electron donor and (ii) attacked by free radicals produced from DO and H2O2. In this system, both pollutants and DO were effectively utilized.
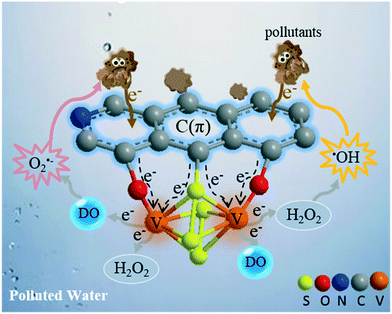 |
| Fig. 8 A schematic diagram showing the interfacial reaction mechanism in the VSO–C(π)/pollutants/DO/H2O2 systems. | |
Conclusions
In summary, VSO–C(π) was successfully designed and prepared via a hydrothermal process. In the VSO–C(π)/pollutants/DO/H2O2 system, RhB exhibited excellent degradation activity with a degradation rate of 97.6% within 15 min, and the degradation rate remained above 90% after six cycles. The catalyst also showed superior adaptability to pH changes and different salt environments. According to the characterization results, dual reaction microcenters formed in VSO–C(π) due to the cross-linking of VS4 with graphene-like C(π) to form C(π)–S–V and C(π)–O–V bridges, which activated the π electrons, transferred them from C(π) to the metal V microcenters, and enhanced the polarization distribution of the electrons. At the electron-poor C(π) microcenters, pollutants were oxidized as electron donors, while at the electron-rich V microcenters, DO and H2O2 were reduced as electron acceptors, which greatly promoted the sustainable electronic cycle of pollutants–DO/H2O2 and resulted in excellent pollutant removal performance. In this work, pollutants as electron donors and DO as an electron acceptor were effectively utilized, providing a strategy for realizing low energy consumption and high-efficiency AOPs.
Conflicts of interest
There are no conflicts to declare.
Acknowledgements
This work was financially supported by the National Natural Science Foundation of China (52070046, 51808140, 51838005, and 51538013), the introduced innovative R&D team project under the “The Pearl River Talent Recruitment Program” of Guangdong Province (2019ZT08L387), the Natural Science Foundation of Guangdong Province (2018A030313487), the Funding Program of Postgraduate Creative Ability Training in Guangzhou University (2019GDJC-M10), the National College Students' Innovation and Entrepreneurship Training Program of Guangzhou University (202011078019 and 201911078037) and the Guangdong Province Universities and Colleges Pearl River Scholar Funded Scheme (Young Scholar), China.
Notes and references
- S. Zhan, H. Zhang, X. Mi, Y. Zhao, C. Hu and L. Lyu, Efficient Fenton-like process for pollutant removal in electron-rich/poor reaction sites induced by surface oxygen vacancy over cobalt-zinc oxides, Environ. Sci. Technol., 2020, 54, 8333–8343 CrossRef CAS PubMed.
- L. Lyu and C. Hu, Heterogeneous Fenton catalytic water treatment technology and mechanism, Progress in Chemistry, 2017, 29, 981–999 CAS.
- K. R. Crincoli, P. K. Jones and S. G. Huling, Fenton-driven oxidation of contaminant-spent granular activated carbon (GAC): GAC selection and implications, Sci. Total Environ., 2020, 734, 139435 CrossRef CAS PubMed.
- Y. Liu, N. Tan, J. Guo and J. Wang, Catalytic activation of O2 by Al0-CNTs-Cu2O composite for Fenton-like degradation of sulfamerazine antibiotic at wide pH range, J. Hazard. Mater., 2020, 396, 122751 CrossRef CAS PubMed.
- C. Lu, K. Deng, C. Hu and L. Lyu, Dual-reaction-center catalytic process continues Fenton's story, Front. Environ. Sci. Eng., 2020, 14, 5 CrossRef.
- L. Lyu, L. Zhang, Q. Wang, Y. Nie and C. Hu, Enhanced Fenton catalytic efficiency of gamma-Cu-Al2O3 by sigma-Cu2+-Ligand complexes from aromatic pollutant degradation, Environ. Sci. Technol., 2015, 49, 8639–8647 CrossRef PubMed.
- Y. Wang, H. Zhao and G. Zhao, Iron-copper bimetallic nanoparticles embedded within ordered mesoporous carbon as effective and stable heterogeneous Fenton catalyst for the degradation of organic contaminants, Appl. Catal., B, 2015, 164, 396–406 CrossRef CAS.
- Y. Ding, W. Huang, Z. Ding, G. Nie and H. Tang, Dramatically enhanced Fenton oxidation of carbamazepine with easily recyclable microscaled CuFeO2 by hydroxylamine: Kinetic and mechanism study, Sep. Purif. Technol., 2016, 168, 223–231 CrossRef CAS.
- X. Yang, X. Xu, J. Xu and Y. Han, Iron oxychloride (FeOCl): An efficient Fenton-like catalyst for producing hydroxyl radicals in degradation of organic contaminants, J. Am. Chem. Soc., 2013, 135, 16058–16061 CrossRef CAS PubMed.
- V. Cleveland, J.-P. Bingham and E. Kan, Heterogeneous Fenton degradation of bisphenol A by carbon nanotube-supported Fe3O4, Sep. Purif. Technol., 2014, 133, 388–395 CrossRef CAS.
- X. Hou, X. Huang, F. Jia, Z. Ai, J. Zhao and L. Zhang, Hydroxylamine promoted goethite surface Fenton degradation of organic pollutants, Environ. Sci. Technol., 2017, 51, 5118–5126 CrossRef CAS PubMed.
- J. Ma, L. Xu, C. Shen, C. Hu, W. Liu and Y. Wen, Fe-N-Graphene wrapped Al2O3/pentlandite from microalgae: High Fenton catalytic efficiency from enhanced Fe3+ reduction, Environ. Sci. Technol., 2018, 52, 3608–3614 CrossRef CAS PubMed.
- J. Tang and J. Wang, Metal organic framework with coordinatively unsaturated sites as efficient Fenton-like catalyst for enhanced degradation of sulfamethazine, Environ. Sci. Technol., 2018, 52, 5367–5377 CrossRef CAS PubMed.
- X. Zhang, J. Liang, Y. Sun, F. Zhang, C. Li, C. Hu and L. Lyu, Mesoporous reduction state cobalt species-doped silica nanospheres: An efficient Fenton-like catalyst for dual-pathway degradation of organic pollutants, J. Colloid Interface Sci., 2020, 576, 59–67 CrossRef CAS PubMed.
- L. Lyu, L. Zhang and C. Hu, Enhanced Fenton-like degradation of pharmaceuticals over framework copper species in copper-doped mesoporous silica microspheres, Chem. Eng. J., 2015, 274, 298–306 CrossRef.
- L. Lyu, L. Zhang, C. Hu and M. Yang, Enhanced Fenton-catalytic efficiency by highly accessible active sites on dandelion-like copper-aluminum-silica nanospheres for water purification, J. Mater. Chem. A, 2016, 4, 8610–8619 RSC.
- Y. Zhang, Z. Chen, L. Zhou, P. Wu, Y. Zhao, Y. Lai and F. Wang, Heterogeneous Fenton degradation of bisphenol A using Fe3O4@beta-CD/rGO composite: Synergistic effect, principle and way of degradation, Environ. Pollut., 2019, 244, 93–101 CrossRef CAS PubMed.
- L. Lyu, L. Zhang, G. He, H. He and C. Hu, Selective H2O2 conversion to hydroxyl radicals in the electron-rich area of hydroxylated C-g-C3N4/CuCo-Al2O3, J. Mater. Chem. A, 2017, 5, 7153–7164 RSC.
- L. Lyu, D. Yan, G. Yu, W. Cao and C. Hu, Efficient destruction of pollutants in water by a dual-reaction center Fenton-like process over carbon nitride compounds-complexed Cu(II)-CuAlO2, Environ. Sci. Technol., 2018, 52, 4294–4304 CrossRef CAS PubMed.
- L. Lyu, M. Han, W. Cao, Y. Gao, Q. Zeng, G. Yu, X. Huang and C. Hu, Efficient Fenton-like process for organic pollutant degradation on Cu-doped mesoporous polyimide nanocomposites, Environ. Sci.: Nano, 2019, 6, 798–808 RSC.
- M. Han, L. Lyu, Y. Huang, J. Liang, M. Xue, T. Wu, J. Li, M. Chen and C. Hu, In situ generation and efficient activation of H2O2 for pollutant degradation over CoMoS2 nanosphere-embedded rGO nanosheets and its interfacial reaction mechanism, J. Colloid Interface Sci., 2019, 543, 214–224 CrossRef CAS PubMed.
- W. Cao, M. Han, L. Lyu, C. Hu and F. Xiao, Efficient Fenton-like process induced by fortified electron-rich O microcenter on the reduction state Cu-doped CNO polymer, ACS Appl. Mater. Interfaces, 2019, 11, 16496–16505 CrossRef CAS PubMed.
- L. Lyu, W. Cao, G. Yu, D. Yan, K. Deng, C. Lu and C. Hu, Enhanced polarization of electron-poor/rich micro-centers over nZVCu-Cu (II)-rGO for pollutant removal with H2O2, J. Hazard. Mater., 2020, 383, 121182 CrossRef PubMed.
- H. Zhang, C. Li, L. Lyu and C. Hu, Surface oxygen vacancy inducing peroxymonosulfate activation through electron donation of pollutants over cobalt-zinc ferrite for water purification, Appl. Catal., B, 2020, 270, 118874 CrossRef CAS.
- Y. Zhuang, Q. Liu, Y. Kong, C. Shen, H. Hao, D. Dionysiou and B. Shi, Enhanced antibiotic removal through a dual-reaction-center Fenton-like process in 3D graphene based hydrogels, Environ. Sci.: Nano, 2019, 6, 388–398 RSC.
- Z. Xie, J. Zhou, J. Wang, C. P. Francois-Xavier and T. Wintgens, Novel Fenton-like catalyst gamma-Cu-Al2O3-Bi12O15Cl6 with electron-poor Cu centre and electron-rich Bi centre for enhancement of phenolic compounds degradation and H2O2 utilization: The synergistic effects of sigma-Cu-ligand, dual-reaction centres and oxygen vacancies, Appl. Catal., B, 2019, 253, 28–40 CrossRef CAS.
- S. Xu, H. Zhu, W. Cao, Z. Wen, J. Wang, C. Francois-Xavier and T. Wintgens, Cu-Al2O3-g-C3N4 and Cu-Al2O3-C-dots with dual-reaction centres for simultaneous enhancement of Fenton-like catalytic activity and selective H2O2 conversion to hydroxyl radicals, Appl. Catal., B, 2018, 234, 223–233 CrossRef CAS.
- L. Luo, J. Li, H. Yaghoobnejad Asl and A. Manthiram, In-Situ assembled VS4 as a polysulfide mediator for high-loading lithium-sulfur batteries, ACS Energy Lett., 2020, 5, 1177–1185 CrossRef CAS.
- Q. Zhu, Q. Xiao, B. Zhang, Z. Yan, X. Liu, S. Chen, Z. Ren and Y. Yu, VS4 with a chain crystal structure used as an intercalation cathode for aqueous Zn-ion batteries, J. Mater. Chem. A, 2020, 8, 10761–10766 RSC.
- C. S. Rout, B. H. Kim, X. Xu, J. Yang, H. Y. Jeong, D. Odkhuu, N. Park, J. Cho and H. S. Shin, Synthesis and characterization of patronite form of vanadium sulfide on graphitic layer, J. Am. Chem. Soc., 2013, 135, 8720–8725 CrossRef CAS PubMed.
- Y. Zhou, P. Liu, F. Jiang, J. Tian, H. Cui and J. Yang, Vanadium sulfide sub-microspheres: A new near-infrared-driven photocatalyst, J. Colloid Interface Sci., 2017, 498, 442–448 CrossRef CAS PubMed.
- D. Torres, J. L. Pinilla, R. Moliner and I. Suelves, On the oxidation degree of few-layer graphene oxide sheets obtained from chemically oxidized multiwall carbon nanotubes, Carbon, 2015, 81, 405–417 CrossRef CAS.
- G. Luo, X. Jiang, M. Li, Q. Shen, L. Zhang and H. Yu, Facile fabrication and enhanced photocatalytic performance of Ag/AgCl/rGO heterostructure photocatalyst, ACS Appl. Mater. Interfaces, 2013, 5, 2161–2168 CrossRef CAS PubMed.
- M. Liu, L. Gan, W. Xiong, Z. Xu, D. Zhu and L. Chen, Development of MnO2/porous carbon microspheres with a partially graphitic structure for high performance supercapacitor electrodes, J. Mater. Chem. A, 2014, 2, 2555–2562 RSC.
- N. A. Kalam, C. Sengottaiyan, R. Jayavel, K. Ariga, R. G. Shrestha, T. Subramani, S. Sankar and L. K. Shrestha, Anadium sulfide/reduced graphene oxide composite with enhanced supercapacitance performance, J. Taiwan Inst. Chem. Eng., 2018, 92, 72–79 CrossRef.
- Y. Qu, M. Shao, Y. Shao, M. Yang, J. Xu, C. T. Kwok, X. Shi, Z. Lu and H. Pan, Ultra-high electrocatalytic activity of VS2 nanoflowers for efficient hydrogen evolution reaction, J. Mater. Chem. A, 2017, 5, 15080–15086 RSC.
- M. Li, G. Sun, P. Yin, C. Ruan and K. Ai, Controlling the formation of rodlike V2O5 nanocrystals on reduced graphene oxide for high-performance supercapacitors, ACS Appl. Mater. Interfaces, 2013, 5, 11462–11470 CrossRef CAS PubMed.
- M. Guo, J. Balamurugan, N. H. Kim and J. H. Lee, High-energy solid-state asymmetric supercapacitor based on nickel vanadium oxide/NG and iron vanadium oxide/NG electrodes, Appl. Catal., B, 2018, 239, 290–299 CrossRef CAS.
- B. Zhang, S. Zou, R. Cai, M. Li and Z. He, Highly-efficient photocatalytic disinfection of Escherichia coli under visible light using carbon supported Vanadium Tetrasulfide nanocomposites, Appl. Catal., B, 2018, 224, 383–393 CrossRef CAS.
- W. Guo and D. Wu, Facile synthesis of VS4/graphene nanocomposites and their visible-light-driven photocatalytic water splitting activities, Int. J. Hydrogen Energy, 2014, 39, 16832–16840 CrossRef CAS.
- A. Santoni, F. Rondino, C. Malerba, M. Valentini and A. Mittiga, Electronic structure of Ar+ ion-sputtered thin-film MoS2: A XPS and IPES study, Appl. Surf. Sci., 2017, 392, 795–800 CrossRef CAS.
- M. Shao, Y. Shao, S. Ding, J. Wang, J. Xu, Y. Qu, X. Zhong, X. Chen, W. F. Ip, N. Wang, B. Xu, X. Shi, X. Wang and H. Pan, Vanadium disulfide decorated graphitic carbon nitride for super-efficient solar-driven hydrogen evolution, Appl. Catal., B, 2018, 237, 295–301 CrossRef CAS.
- F. Ureña-Begara, A. Crunteanu and J.-P. Raskin, Raman and XPS characterization of vanadium oxide thin films with temperature, Appl. Surf. Sci., 2017, 403, 717–727 CrossRef.
- Y. Gao, Y. Zhu, L. Lyu, Q. Zeng, X. Xing and C. Hu, Electronic structure modulation of graphitic carbon nitride by oxygen doping for enhanced catalytic degradation of organic pollutants through peroxymonosulfate activation, Environ. Sci. Technol., 2018, 52, 14371–14380 CrossRef CAS PubMed.
- L. Wang, D. Yan, L. Lyu, C. Hu, N. Jiang and L. Zhang, Notable light-free catalytic activity for pollutant destruction over flower-like BiOI microspheres by a dual-reaction-center Fenton-like process, J. Colloid Interface Sci., 2018, 527, 251–259 CrossRef CAS PubMed.
- W. Cao, L. Lyu, K. Deng, C. Lu and C. Hu, l-Ascorbic acid oxygen-induced micro-electronic fields over metal-free polyimide for peroxymonosulfate activation to realize efficient multi-pathway destruction of contaminants, J. Mater. Chem. A, 2020, 8, 810–819 RSC.
- L. Lyu, L. Zhang and C. Hu, Galvanic-like cells produced by negative charge nonuniformity of lattice oxygen on d-TiCuAl–SiO2 nanospheres for enhancement of Fenton-catalytic efficiency, Environ. Sci.: Nano, 2016, 3, 1483–1492 RSC.
- Y. Zhuang, X. Wang, Q. Liu and B. Shi, N-doped FeOOH/RGO hydrogels with a dual-reaction-center for enhanced catalytic removal of organic pollutants, Chem. Eng. J., 2020, 379, 122310 CrossRef CAS.
Footnotes |
† Electronic supplementary information (ESI) available. See DOI: 10.1039/d0en00982b |
‡ Deng and Gao contributed equally to this paper. |
|
This journal is © The Royal Society of Chemistry 2021 |
Click here to see how this site uses Cookies. View our privacy policy here.