DOI:
10.1039/D0EE01545H
(Review Article)
Energy Environ. Sci., 2021,
14, 815-843
Challenges in the use of hydrogen for maritime applications
Received
15th May 2020
, Accepted 8th December 2020
First published on 7th January 2021
Abstract
Maritime shipping is a key factor that enables the global economy, however the pressure it exerts on the environment is increasing rapidly. In order to reduce the emissions of harmful greenhouse gasses, the search is on for alternative fuels for the maritime shipping industry. In this work the usefulness of hydrogen and hydrogen carriers is being investigated as a fuel for sea going ships. Due to the low volumetric energy density of hydrogen under standard conditions, the need for efficient storage of this fuel is high. Key processes in the use of hydrogen are discussed, starting with the production of hydrogen from fossil and renewable sources. The focus of this review is different storage methods, and in this work we discuss the storage of hydrogen at high pressure, in liquefied form at cryogenic temperatures and bound to liquid or solid-state carriers. In this work a theoretical introduction to different hydrogen storage methods precedes an analysis of the energy-efficiency and practical storage density of the carriers. In the final section the major challenges and hurdles for the development of hydrogen storage for the maritime industry are discussed. The most likely challenges will be the development of a new bunkering infrastructure and suitable monitoring of the safety to ensure safe operation of these hydrogen carriers on board the ship.
Broader context
The shipping industry is crucial for today's global economy and is becoming an increasingly more polluting factor in the transport sector. New technologies must be adopted to reduce the pollution from the maritime industry. Ships for seagoing transport usually have a lifetime of around 20 years, so if a ship adopts a new technology to reduce its environmental impact, the decision has to be well considered to make sure that it will benefit the environment during the entire operation lifetime of the ship. This review aims to inform on the different aspects of hydrogen use as a maritime fuel and to give an objective outlook of the advantages and disadvantages and the major hurdles that come with the storage of hydrogen on board of seagoing ships.
|
1 Introduction
For millennia, humans have depended on the use of seas and waterways for the transportation of goods. Up until the late 18th century, the main power for ship propulsion was wind in the sails. This changed after the development of steamships powered by coal, and by half-way through the 20th century, diesel fueled ships had taken over. This development made ships go faster, but as trade grew, ships also became larger and heavier thereby dramatically increasing the cost on the environment. To date, if it were a country, the shipping industry would be the 6th largest emitter of CO2, with total emissions in the range of 900 million tons of CO2 per year.1,2 The European Commission projected an increase in global CO2 emissions from shipping of 80% between 1990 and 2020,3 and it is estimated that without action the global share of shipping's greenhouse gas (GHG) emissions may reach 17% by 2050.4 The International Maritime Organization (IMO) has set a 50% reduction target for emissions related to maritime transport by 2050 compared to 2008.5 The shipping industry is also responsible for a large amount of air pollutants: emissions of NOx and SOx represent roughly 15% and 13% of the global total, respectively.6 A proposed solution to the NOx and SOx emission in the shipping industry is the use of liquefied natural gas (LNG). In theory, this could even lead to a reduction of CO2 emissions by 23% compared to diesel, since LNG has a higher hydrogen to carbon ratio.7 However, methane slip both on the engine level and in the production chain of the liquefied natural gas in reality nullifies this effect and no real greenhouse gas reduction is achieved through the use of LNG.8 The IMO has placed more and more stringent restrictions on the emissions of NOx and SOx from ships, and emissions control areas (ECAs) have been designated (such as the North and Baltic sea) where there are more stringent restrictions on the emissions of air pollutants. The IMO has also implemented the energy efficiency design index (EEDI), a design tool aiming at reducing the emissions of newly built ships. Seagoing ships have an operating lifetime of over 20 years, and since the EEDI only applies to newly built ships, the global fleet is not expected to comply with this index before 2040. This makes the usefulness of the EEDI highly contested.8 The reductions that can be reached with the design index are not at all ambitious in order to reach the emissions to combat climate change.6 To reduce the emissions of the shipping industry, more focus should be placed on other methods than ship design. Other pathways are the use of operational measures such as mandatory speed limits, for example, lower sailing speeds and a better communication between ship and port. These measures can effectively reduce the CO2 emissions from shipping already by 1–60% depending on how strict the conditions are set.9 Next to ship design and the operational methods, the most disruptive way to reduce the CO2 emissions from shipping is switching away from fossil fuels, towards renewable fuels, with lower net CO2-emissions.
Hydrogen is gaining a lot of attention as a clean fuel, since it can be generated from renewable energy through electrolysis. The production of hydrogen through electrolysis is an established technology, but not the current industrial standard.10 Current methods for producing hydrogen rely on the use of fossil fuels as a starting material. It is however possible to use water and excess renewable (solar or wind) energy for the production of hydrogen.11 The potential for solar energy use is huge: on the earth's surface the incident solar energy is estimated to be 48
000 TW. The shipping industry uses less than 0.5 TW year.12 An additional benefit is that both water and renewable energy are available globally (although cost of electricity can vary widely between regions), whereas fossil fuels are unevenly distributed on the globe. To use hydrogen as an on-board fuel, a number of demonstration projects have already been initiated, both using hydrogen fuel cells and by using hydrogen adopted combustion engines. The most crucial bottleneck with hydrogen as a fuel is likely not the production or the end-point use but rather the storage, having even been called a show stopper in the past.13 By weight, hydrogen is an excellent energy carrier with a lower heating value (LHV) roughly 3 times that of diesel, 33 kW h kg−1 compared to 11.39 kW h kg−1. However, hydrogen is such a light gas that under atmospheric conditions the total energy content is only 3.06 W h L−1 whereas diesel contains 10.08 kW h L−1, roughly a difference of factor 3000. To deal with this low volumetric energy content at atmospheric conditions several technologies exist to concentrate hydrogen and make storage more efficient. These include: compression, liquefaction and storage in physical or chemical carriers.14 The storage of hydrogen in physical/chemical carriers is an innovative way of handling hydrogen storage. The principle is that hydrogen is not stored as a pure compound, but bound to a carrier either via adsorption (for physical storage)15–17 or by chemical bounds.18–22 In this review we will not focus on the use of physical (adsorption) based hydrogen storage, but we will specifically discuss chemical carrier molecules for hydrogen. Many carriers for hydrogen have been proposed and these can be gasses (like nitrogen or CO2),23–27 liquids (e.g. toluene) or solid materials (e.g. metals or borane compounds). Gaseous hydrogen compressed at 70 MPa, is a frequently used storage technique in the automotive industry28 and we will use this as a reference in this work. The hydrogen forms in liquid form that are considered are: liquid hydrogen, ammonia, synthetic fuels (from recycled CO2) and liquid organic hydrogen carriers (LOHC). As solid materials we consider some examples of metal hydrides (MgH2, NaAlH4, laves-type AB2 hydrides) and the borane compounds NaBH4 and NH3BH3.
Hydrogen storage for maritime applications is different and shows different challenges than hydrogen storage for stationary or automotive applications. However, the storage of hydrogen for maritime shipping is more challenging than these other cases. On the one hand, large quantities of hydrogen are stored on an isolated ship, whereas in large-scale stationary storage, there is the possibility of external energy input to either release the hydrogen from the carrier or keep it under ideal storage conditions. On the other hand, the storage on a ship is less restricted by weight and volume requirements compared to the automotive industries, and more extreme temperatures can also be used more safely for hydrogen storage on a large ship than in a much smaller passenger vehicle.
2 Production of hydrogen
Although the hydrogen atom is the most abundant atom in the universe, molecular hydrogen gas itself is not an earth abundant resource. Hydrogen gas has to be produced from other products.29 It is not an energy source like fossil fuels, but it functions more as an energy vector. Hydrogen is mostly produced as a chemical feedstock with an estimated global production of 70 million tons of pure hydrogen in 2019.30 There exist several methods for the production of hydrogen, but not all production methods for hydrogen can be considered green. Currently, about 96% of all hydrogen produced, is produced via reforming processes of fossil fuels, either natural gas, heavy oil and naphtha or from coal. These processes produce large quantities of CO2,31 and so alternative methods for hydrogen production are under investigation. These methods use renewable feedstocks like water, and biomass. Different hydrogen production methods have been reviewed in depth by Nikolaidis and Poullikkas32 and the section on hydrogen production of this review only aims to provide the reader a basic understanding of hydrogen production, by steam reforming of methane and by electrolysis. These methods were selected since they are proven technologies, already performed on a MW scale. An exception to this is membraneless electrolysis, which is a new technology but is strongly linked to the maritime industry since it was originally developed as a ballast water purification system.
2.1 Reforming of methane
Steam reforming of methane is the current state-of-the-art technology for hydrogen production. Currently, about 48% of the globally produced hydrogen is produced via steam reforming. Two process steps are required to convert methane to hydrogen and CO2. The first step in the process converts the methane to hydrogen and CO, according to eqn (1).33 | CH4 + H2O ⇌ CO + 3H2 ΔH = 206 kJ mol−1 | (1) |
In order to increase the hydrogen yield of the process, the water–gas-shift reaction occurs in the next step of this chemical process (eqn (2)).33 | CO + H2O ⇌ CO2 + H2 ΔH = −41 kJ mol−1 | (2) |
From one molecule of methane and 2 molecules of water, a total of 4 moles of hydrogen can be generated, while seemingly only emitting one mole of CO2. However, the overall reaction for hydrogen production is endothermic, and this heat is supplied by the combustion of fossil fuels, generating an additional emission of greenhouse gasses. A study showed that the median CO2 emission was 9 kg CO2 per kg hydrogen.34 Using the lower heating value of hydrogen, this resulted in a median emission of hydrogen of 270 g of CO2 per kW hH2 energy produced. Diesel fuels are known to emit ±3.15 g of CO2 per combusted gram of fuel.35 Using the same calculations as for hydrogen, 277 g of CO2 is emitted per kW h of diesel used. The advantage of lowering greenhouse gas emissions by using hydrogen from steam reforming is thus minimal, if no extra CO2 mitigation measures are taken to reduce the emissions of the steam reforming plant.36 CO2 emissions from steam reforming are not limited to the emissions from the chemical process; the heating of the product to high temperatures by combustion on natural gas, can contribute up to 41% of the total CO2 emissions in the process.37 The electrification of the reforming reactors is a possible new pathway that is under research to lower the emissions related to steam reforming. Instead of combusting natural gas for heat, the heat would be supplied by electricity; this not only has the potential to reduce CO2 emissions from the combustion, but it would also allow for higher methane conversion.36
Next to electrification chemical engineers are working on the development of dry reforming of methane; this process combines two greenhouse gasses, CO2 and methane, to generate hydrogen and carbon monoxide. This reaction follows eqn (3) at temperatures above 750 °C.38
| CH4 + CO2 ⇌ 2CO + 2H2 ΔH0298K = 260.5 kJ mol−1 | (3) |
This process provides a more sustainable alternative to steam reforming, since it turns a waste gas, carbon dioxide into syngas (carbon monoxide and hydrogen), which is a useful gas stream in the chemical industry.
39 This technology is often sighted in line with bio gas reforming, to enable a more sustainable production method of the syngas. Currently, dry reforming is not only used on large scales, but also this technology does provide a way of producing H
2 from natural gas or biogas.
40–42 Since this process still uses fossil fuels it is less likely to be as disruptive a technology as renewable energy production and this could ease the pathway to truly renewable hydrogen.
2.2 Water electrolysis
An alternative method of hydrogen production is the electrolysis of water. There exist several different kinds of water electrolysis reactions, but all start from the same common concept that when a high enough electrical current is passed through water, the water will split into hydrogen and oxygen gas (eqn (4)). | 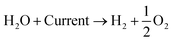 | (4) |
The different electrolysis methods that are discussed here are: alkaline, polymer electrolyte membrane, solid oxide and membraneless electrolysis. Electrolysis of water can be combined with renewable energy and is seen as a method to stabilize the intermittent character of renewable energy, by converting the excess energy to hydrogen.43 The cost for this renewable hydrogen is still higher than that of steam reforming of methane, expressed in US$2016 the price for hydrogen from natural gas could be as low as 0.91–1.69 $ kg−1, whereas the prices for renewable hydrogen could be as high as 3.56–9.08 $ kg−1 for wind energy and 3.34–17.30 $ kg−1 for solar energy.44 The availability of energy is a critical factor in the development of water splitting technology; the energy required to release one mole of hydrogen by water splitting is 7 times higher than the energy required to release a mole of hydrogen from methane.45
2.2.1 Alkaline electrolysis.
Alkaline electrolysis is the state-of-the-art electrolysis technology; it consists of two electrodes that are separated by a diaphragm46 that permeates ions in water but not the evolved gasses.47,48 A schematic view is seen in Fig. 1A. When current is applied to the electrodes the protons in water are reduced to hydrogen gas on the cathode, and oxygen is evolved from the oxidation of hydroxide ions at the anode. The reactions that occur at the electrodes are as given by eqn (5) and (6):49 | Cathode: 4H2O + 4e− → 2H2 + 4OH− | (5) |
| Anode: 4OH− → O2 + 2H2O + 4e− | (6) |
To separate the electrodes a diaphragm is used; traditionally asbestos was used and this limited the operating temperature of alkaline electrolysers to 80 °C. Due to the health risks of asbestos this is now replaced by alternative materials. Alternative materials are also under study to increase the working temperature of these electrolysers up to 150 °C; at higher temperatures less energy is required for water electrolysis reaction.50
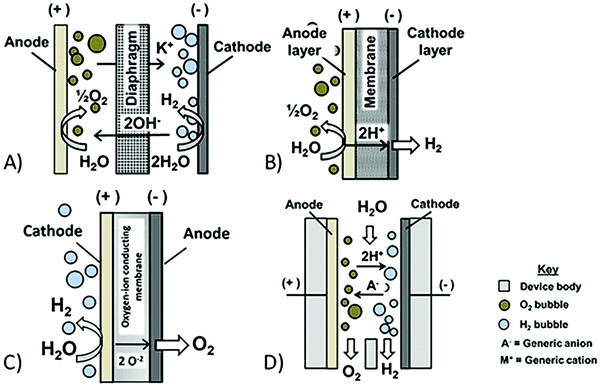 |
| Fig. 1 Schematic side-view of discussed electrolysis technology, based on ref. 58. (A) Conventional alkaline electrolysis, (B) conventional PEM, (C) solid oxide electrolysis, and (D) membraneless electrolysis. | |
Traditionally alkaline electrolysers are operated at near atmospheric pressures (0.1–0.6 MPa); however, systems at 70 MPa also exist, and these systems have the advantage that less energy is required for post-processing of the hydrogen for high pressure applications, but at the cost of reduced hydrogen purity.51 Still there is a high cost related to electrolysis as the most efficient electrodes are made of platinum,45 and purified water is required in the electrolysis process.52
2.2.2 Polymer electrolyte membrane electrolysis.
A second type of electrolysis is polymer electrolyte membrane (PEM) electrolysis, Fig. 1B. Here, the aqueous electrolyte is replaced by a proton conducting polymer membrane, that does not permeate gasses. The reactions at the electrodes are different from those in alkaline electrolysers, since only protons and electrons can be transported between the electrodes. The reactions at the anode and cathode are given by eqn (7) and (8):43 | 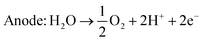 | (7) |
| Cathode: 2H+ + 2e− → H2 | (8) |
Water is split into oxygen and protons and the protons then migrate through the membrane to the cathode where a reduction reaction to hydrogen gas takes place. The materials used for the cathode, anode and membrane greatly influence the efficiency and the associated cost of a PEM electrolyser. As a cathode material, platinum deposited on carbon is frequently used; for the anode, iridium or iridium–ruthenium based catalysts are selected. For the membrane Nafion™ or other fluorinated polysulfonic acid membranes are the most likely choice.53 In practice the anode, cathode and membrane are assembled to make a compact membrane electrode assembly (MEA). By placing all components close to each other the distance the electrons and protons have to migrate is lowered and this improves the overall efficiency of the system. However, the overall efficiency of PEM electrolysers is still lower than in alkaline electrolysers, but due to the low permeability of the membrane for the gasses, PEM have reported hydrogen purities of 99.999 vol% without post-processing.43,51
2.2.3 Solid oxide electrolysis.
Of all types of electrolysers discussed in the literature, the system that requires the least electrical energy requirement is the solid oxide electrolyser. These systems operate at temperatures above 800 °C and due to the high thermal energy supplied to the system, less electrical energy is required. This is especially advantageous to the overall electrical efficiency if these high temperatures can – at least partially – be supplied by high temperature waste heat stream from e.g. industrial plants or from a geothermal source. Solid oxide electrolysers have an oxygen-ion conductive membrane and the electrochemical reactions at the electrodes are then given in eqn (9) and (10):54 | Cathode: 2H2O + 4e− → 2H2 + 2O2− | (9) |
| Anode: 2O2− → O2 + 4e− | (10) |
Just like with other electrolysers the membrane in a solid oxide electrolyser plays a crucial role. In this type of electrolyser, the most used membrane is yttria stabilized zirconia, a material that allows oxygen ions to pass through while remaining stable and electronically insulating even at higher temperatures. Solid oxide electrolyzers can be designed without the need for expensive noble metal electrodes. As the hydrogen electrode, the typical electrode material used is nickel cermet (a composite ceramic and metallic material), these materials show a high electrical conductivity and a high activity towards the electrochemical reactions. For the oxygen anode, most often perovskite materials are used, commonly LaMnO3.55 A schematic of a solid oxide electrolyser is shown in Fig. 1. However, solid oxide electrolysers are still in an R&D phase and face critical issues concerning thermal stability. Continued heating and cooling of the system due to load changes result in microscopic cracks in the membrane and this is detrimental to the entire system. Since load changes cause issues with operational stability of the solid oxide electrolysers, these systems are described by some authors to be incompatible with grid stabilization of renewable energy,51 since grid stabilization requires the electrolyser system to be switched on and off ad hoc.
2.2.4 Membraneless electrolysis.
Having been originally designed as a ballast water purification system, membraneless electrolysis is an innovative new concept for hydrogen production, that has the potential to significantly reduce the cost of electrolysis equipment due to the simple configuration of the device. The simplest setup of a membraneless electrolyser is based on flow-by electrodes; in this system the electrolyte flows parallel to the electrodes and into two different outflow channels at the end of the device, as can be seen in Fig. 1D. These systems use the Segré–Silberg effect where the gas bubbles are pinned close to the electrolyte surface where they are evolved thanks to the fluid gradient of the electrolyte. This means that by flowing the electrolyte through the channel and into the separated outlets the hydrogen and oxygen gas streams are separated from each other by the flow of the aqueous stream. The reactions at the separated electrodes are given in eqn (11) and (12):56 | Cathode: 2H2O + 2e− → 2OH− + H2 | (11) |
| 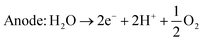 | (12) |
This system can, however, not produce hydrogen of the same purity as e.g. PEM electrolysers but the reported purity for the hydrogen stream is still as good as 99%.57 Since no membranes are involved in this system there are significant advantages over other types of electrolysers: the first advantage is the simple configuration of the device. Due to the simplicity of the membraneless system, it is also quite resilient to impurities and a wide range of operating conditions.58 Membraneless electrolysers are also known to run on tap water and even brine media.57 Another advantage is that membraneless electrolysers can work over a broad range of the pH spectrum (0.35–13.7)59 and in a broad spectrum of electrolyte solutions.57,59 All these advantages have to be weighed against the fact that membraneless electrolysers have a lower voltage efficiency compared to other systems; this is due the larger distance between the electrodes and thus the higher internal electrical resistance of the system compared to other electrolyser systems.58 This means that higher currents have to be used with this system to generate the same amount of hydrogen compared to other fuel cell systems. Table 1 summarizes the specifications of the different types of electrolyzers.
Table 1 Operational details of discussed electrolysis technologies
Membraneless electrolysers operating with electrolytes containing salts are also able to produce acids and bases for the chemical industry, and these value added chemicals have the potential to lower the cost of hydrogen production by membraneless electrolysis.56
3 Hydrogen storage methods
In this section the main different hydrogen storage technologies with potential applicability in the maritime industry are reviewed. Again, this section aims to provide insights into the basics of each technology, and its benefits and drawbacks. Readers already familiar with hydrogen storage technologies, can proceed directly to Section 4 in which these technologies are compared based on their energy efficiency, followed by Section 5 that discusses their actual applicability in the maritime industry.
3.1 Compressed hydrogen
The currently most developed and widely used method to store hydrogen is by compression. Five different types of storage tanks have been developed and are used to store hydrogen at increased pressures (from 10–20 to 70 MPa). These tank types are generally denoted by Roman numerals, I, II, III, IV and V based on the complexity of the storage tank. Type I pressure vessels are metallic, type II vessels are made of a combination of a polymer liner wrapped around a metallic tank, but with the polymer liner only wrapped around the cylindrical part of the vessel. Type III tanks build on the type II vessels with the addition of a metallic liner fully wrapped with the composite fibre for support. Type IV pressure vessels consist of a polymeric liner that is fully wrapped with composite fibres.61,62 They do not require the use of a metal vessel body, and this benefits their gravimetric efficiency, but decreases the system's thermal resistance.63 For the type V tanks a patent has been submitted in 2014, and these are all composite tanks without any liner and claim to work at pressures above 700 bar.64 However, there are only limited applications of these type V tanks.65
Most common types of pressure vessels for hydrogen storage (or gas storage in general) are type I metallic pressure vessels; they can store gas up to pressures of 20–30 MPa. Type II tanks are lighter than type I tanks but typically the pressures they can withstand only go up to 20 MPa. The gravimetric storage capacity of a type II tank is higher than for type I, 2.1 wt% vs. 1.7 wt%. Type III, IV and V are adapted for higher pressures up to 70 MPa for type III and IV and even higher for type V. The type IV storage vessels have reported gravimetric capacities of 5.7 wt% and are used to store hydrogen in fuel cell-powered cars. This high gravimetric storage capacity in gas cylinders is reported for the gas tanks of the Toyota Mirai.66 This higher storage capacity for hydrogen is translated into an almost ten times increase in cost per kilogram of hydrogen stored when compared to cheaper low pressure vessels.67 Even at these high pressures only 0.81 kW h L−1 (ref. 67) of hydrogen energy can be stored; this is a hydrogen density of roughly 26 g L−1. The energy penalty for the compression of hydrogen to such a high pressure is about 10% of the energy content of hydrogen (LHV).68
3.2 Liquid hydrogen
Next to compressed hydrogen, hydrogen can also be stored by liquification. When cooled to −253 °C, hydrogen has a density of 70.8 kg m−3, an increase in density of roughly 775 times compared to the density of gaseous hydrogen under atmospheric conditions. The energy density of liquid hydrogen is around 8.5 MJ L−1, compared to the value for diesel fuels of 36.3 MJ L−1, so more volume is required for liquid hydrogen even without considering the thickness of the insulation material for the liquid hydrogen tanks.
The shipping industry is gaining experience with cryogenic fuels as liquefied natural gas (LNG) has gained attention as a fuel with a lower carbon content. More specifications on LNG in the maritime industry can be found in later sections of this review. The challenges with liquid hydrogen in the shipping industry are larger than with LNG (the liquid is roughly 90 °C colder, the density and specific heat capacity are lower, 9.44 J g−1 K−1).69 But from the processes of developing LNG as a fuel, a lot has to be learned and adopted for the use of liquid hydrogen. Handling liquid hydrogen is also developed in other industries as well, liquid hydrogen is well known from its use as a fuel in aerospace programs, such as NASA's Space Shuttle programs.
The first successful attempt at liquifying hydrogen was in 1898 and carried out by Sir James Dewar.70 Since these first experiments the production process for liquid hydrogen has evolved and several cooling pathways have been proven successful at generating liquid hydrogen. These include the Linde–Hampson,71 the Claude,72 the pre-cooled Claude cycle and helium refrigerated systems.73 Of these systems, helium cooled liquid hydrogen production is most efficient, whereas the pre-cooled Claude system is the system of choice in industrial plants.74
The pre-cooled Claude cycle is based on a multi-step cooling cycle via heat exchangers with liquid nitrogen followed by an expansion step in a Joule–Thomson expansion valve to liquify the hydrogen. There are multiple cooling steps in a hydrogen liquefaction plant, combined with an ortho–para conversion step of the hydrogen atom spins. The first set of cooling steps use liquified nitrogen (−196 °C), to cool the hydrogen, which has been pressurised. Subsequent cooling steps use already liquified hydrogen as a coolant. In a final step the cooled hydrogen is depressurised which leads to the liquefaction.
Often the nitrogen used for liquefaction is vented to the atmosphere as is the case with the well described Ingolstadt Liquide Hydrogen plant from Linde in Germany. A total flow of 1750 kg h−1 of liquid nitrogen is required to operate the liquefaction plant. At an estimated value of 0.4 kW h kg−1 required for liquid nitrogen production, the total production of is estimated at 0.9 kW h L−1 or 12.85 kW h kg−1 for liquid hydrogen production.75 A quick calculation shows that approximately 30% of the energy required for the liquefaction of hydrogen, is used to produce liquid nitrogen. Because of the high energy consumption of the liquid nitrogen in the process a European Research Project called IDEALHY focussed on reducing the total energy cost for the liquid hydrogen production. Liquefaction of hydrogen consumes high amounts of energy; values in the range of 12.5–15 kW h kgH2−1 (37–45% of the LHV of hydrogen) are common for industrial plants.70 IDEALHY achieved a theoretical energy consumption below 6.5 kW h kg−1 of liquid hydrogen, by using mixed liquid helium/neon refrigerants instead of nitrogen and using a closed loop for the coolant rather than an open one.76
After the cooling process, the hydrogen must be stored at temperatures below its boiling point at −253 °C. This is equivalent to storing ice-cream in an oven. To deal with this issue, tanks made of super isolating materials are used and the design of the tank is spherical since this lowers the surface to volume ratio of the tank. Even so, NASA's largest liquid hydrogen tank with a capacity of 3800 m3 (25 m diameter) is still reported to lose over 600 m3 per year due to evaporation losses out of the tank.77 Engineers at NASA have been working on a cooling system for a smaller liquid hydrogen tank (125 m3) that was fitted with a liquid helium heat exchanger. They showed that to achieve zero-boil off losses in the liquid hydrogen tank, the heat exchanger had to be switched on and off at time intervals of roughly equal length.78
The automotive industry has also experimented with liquid hydrogen; two types of hydrogen powered cars with liquid hydrogen storage have made it to the market, one being the GM HydroGen3 which is equipped with a 4.6 kg cryogenic hydrogen tank,79 and the other being the BMW hydrogen 7, which has a dual fuel (gasoline–hydrogen) engine and is equipped with a 170 L hydrogen tank which is reported to store around 8 kg of liquid hydrogen.80 Liquid hydrogen did not prove to be the best storage method in the automotive industry, one reason being the large energy requirements for liquefaction, the other being the boil-off. Even with super insulated tanks, the hydrogen could not be stored for over 5 days and liquid hydrogen is not considered the best method for automotive hydrogen storage.28,81,82 For hydrogen powered ships, the boil-off is less of a concern than it is for stationary applications. Much like LNG carriers, hydrogen powered ships that would use cryogenic hydrogen for propulsion, could also be designed to sail on the boil-off gas.83
As of now, no large-scale shipping of liquid hydrogen exists in the maritime industry: liquid hydrogen is not a common commodity that is being shipped globally. There have been previous attempts at studying the transfer of renewable energy across the oceans in the form of liquid hydrogen but they never saw realisation.84 Recently, a first pilot liquid hydrogen tanker ship has been launched by Kawasaki Heavy Industries.85
3.3 Ammonia, N2 based storage
Ammonia is globally one of the most industrially produced chemicals, with yearly production of over 150 million tons and an even further increased production is expected in the coming years. Ammonia is a crucial chemical in fertiliser production and results from combining hydrogen with nitrogen from air in an industrial process called the Haber–Bosch process. This is one of the oldest processes in the chemical industry and the first large scale plant for this process was constructed in Oppau, Germany in 1913 and was able to produce 30 tons of ammonia per day.86 The Haber–Bosch reaction is a catalytic exothermic chemical reaction that takes place at increased temperatures (300–500 °C) and pressure (15–20 MPa) and proceeds through the following global reaction equation:87 | N2 + 3H2 ⇌ 2NH3, ΔH = −91.8 kJ mol−1 | (13) |
From the above reaction it is clear that the source of hydrogen is of little concern for the Haber–Bosch reaction itself. Economic factors however play a vital role and therefore, almost all of today's hydrogen that is produced for ammonia comes from steam reforming of methane, with its associated greenhouse gas emissions, even though ammonia itself is a carbon free chemical. An optimized, modern ammonia plant with hydrogen from methane emits 1.5–1.6 ton CO2eq per ton ammonia.88 In terms of energy this equals a CO2 emission of 290 g kW h−1. Formerly industrial ammonia plants existed that used hydrogen from electrolysis coupled with ammonia production, before the low price of natural gas made them uncompetitive with SMR coupled ammonia plants.89 So, while technically feasible, renewable ammonia is not produced in today's large-scale ammonia plants.
Ammonia can serve both as a hydrogen carrier molecule, and as a fuel in itself. Ammonia has been used in Belgian busses during WW II, due to fuel shortages. However, ammonia has severe drawbacks when used in combustion engines; it has a narrow flammability range (15–28 vol%), a high auto-ignition temperature (651 °C) and a low laminar flame velocity (0.015 m s−1).90 These factors complicate engine operations with ammonia. Ammonia is a known poison for low temperature fuel cells and seriously deteriorates the lifetime of these systems. The reverse reaction of eqn (13) to release the hydrogen out of ammonia could be used to generate hydrogen, in this way nitrogen gas from the air is used as a hydrogen carrier. However, this reaction is highly endothermic and it requires high temperatures (>450 °C)91–93 and extensive purification of the hydrogen gas to remove all ammonia. High temperature fuel cells, or dedicated ammonia fuel cells could also be used to have ammonia as a fuel.
3.4 CO2 based hydrogen storage (methanol, S-LNG, FTS-diesel, formic acid)
Today's shipping industry infrastructure is completely based on liquid carbon-based fuels, mostly diesels (heavy fuel oil, HFO and marine gas oil, MGO) and to a minor (but growing) extent on Liquefied Natural Gas (LNG). The problem with these fuels is that (i) they are a major source of greenhouse gas emissions and (ii) being not renewable, they only represent a transition solution. A possible pathway is to consider making synthetic carbon fuels with CO2 as a feedstock. This is not at all a trivial challenge; due to the higher relative amount of oxygen in CO2 compared to the hydrocarbon fuel, the mass of CO2 increases up to three times. Based on mass an infrastructure for CO2 capture must be roughly threefold greater than the current fuel processing infrastructure. In this work four synthetic fuels are considered, because of their current use in the maritime industry, synthetic diesel, synthetic (liquefied) natural gas (S-LNG) and methanol and formic acid (FA) because of the promise it shows as a hydrogen storage medium. The reaction pathways to these fuels are considered: Fischer–Tropsch synthesis for synthetic diesel fuels, the Sabatier reaction for synthetic natural gas and CO2 hydrogenation to methanol or to formic acid.
3.4.1 Synthetic diesel production.
As early as 1926, Franz Fischer and Hans Tropsch reported their first results on the production of liquid fuels from a gaseous mixture of hydrogen and CO. This synthesis pathway, called the Fischer–Tropsch Synthesis (FTS), can produce a wide range of hydrocarbons. The product of FTS is an Anderson–Flory–Schulz distribution of hydrocarbon molecules ranging from methane to waxes, with a carbon skeleton of over 40 carbon atoms.94 These molecules can be allylic, olefinic or aromatic in nature. As side reactions the formation of alcohols or solid carbon and CO2 (Boudouard reaction) can take place.95 The distribution of the products depends on the process conditions and catalysts used. Typical conditions for the FTS for optimal diesel fuel production, are pressures in the range of 2.5–3 MPa and temperatures of 225 °C. For fuel production, alkanes ranging from eight to around 20 carbon atoms are the preferred product to produce diesel-like fuels.96
FTS has always been a very promising technology to upgrade carbon sources to liquid carbon fuels. This synthesis method is gaining attention as a possible tool to reduce global CO2 emissions, since the FTS route can be modified to use CO2 instead of CO, using an adequate catalyst. Traditional FTS-catalysts are based on cobalt, a metal that is known for its high activity and good distribution of end products. However, cobalt has only a low activity towards the Reverse Water Gas Shift Reaction (RWGS). This reaction converts CO2 to CO and is proposed to be the first step in CO2 based FTS. Cobalt is thus a good catalyst for the second reaction, but not much for the first reaction step in the CO2 FTS process. To promote this first reaction, catalysts based on iron are often used. The proposed reaction steps for CO2 based hydrogenation in FTS are then:97,98
| CO2 + H2 ⇌ CO + H2O, ΔH = 40.6 kJ mol−1 | (14) |
| (2n + 1)H2 + nCO → CnH2n+2 + nH2O, ΔH = −152 kJ mol−1 | (15) |
As can be seen from the reaction equations, only one in three produced hydrogen molecules is actually stored in the new liquid fuel. Since hydrogen production is an important energy cost in the overall process (for alkaline electrolysis 55 kW h per kilogram of hydrogen is required), the loss of this valuable resource comes with a great cost to the efficiency of the production of synthetic liquid hydrocarbon fuels and should be avoided. Since the FTS can only produce mixtures of hydrocarbons, the efficiency of this process is only 50% towards the production of diesel-like chemicals.
99 This further increases the losses of the produced hydrogen.
3.4.2 Synthetic SNG production.
The reaction to produce methane, the main component of natural gas out of CO2 has been described over a century ago. Paul Sabatier was the first to describe the synthesis of methane from hydrogen and CO2 at high temperatures in the presence of metallic nickel. This reaction is described as follows: | CO2 + 4H2 → CH4 + 2H2O, ΔH = −165 kJ mol−1 | (16) |
In current day industry, this reaction is very important for the purification of hydrogen rich gas streams that are contaminated with CO or CO2, which can poison catalysts in e.g. ammonia production.100 Typical process conditions for this process are temperatures of around 400 °C and pressures up to 10 MPa. This reaction is highly exothermic and thus generates a lot of waste heat, with the associated challenges in reactor design for efficient heat removal.
The real potential of using the Sabatier reaction for energy storage and global carbon recycling were only being considered more recently in the concept of power-to-gas or power-to-methane processes, and the increasing interest in this topic can be found in multiple reviews.101–105 The overall process design for CO2 methanation has also been well studied, with many different reactor designs described.106 Different metals have also been tested for the catalytic conversion of CO2 to methane. Nickel is the preferred metal, due to the low cost and near 100% selectivity to methane.107–109
Power-to-methane processes have already resulted in several pilot plants to demonstrate the technology. One such plant is the Power-To-Gas plant in Werlte, Germany. This plant combines an alkaline electrolyser with CO2 capture from a nearby bio-gas production site. The plant can be started up within five minutes, demonstrating the potential of this technology for electrical grid stabilization. This power to methane plant had a total energy capacity of 6 MW. The total methanation reactor unit itself has an efficiency of 78%, resulting in an extra energy cost for hydrogen storage of about 9 kW h per kilogram of hydrogen stored.106 From the global reaction it shows that although the reaction is highly selective towards methane, water is formed as a by-product and half of all produced hydrogen is lost.
3.4.3 Green methanol production.
The production of methanol from CO2 represents another frequently discussed pathway for sustainable fuel production. Nobel Prize laureate George Olah wrote an influential article on the usefulness and possibilities of a methanol economy,110 and this has earned him the honour of having Iceland's first CO2 to methanol plant named after him. This plant is currently able to produce around 300 tons of methanol per day using renewable energy and recycled carbon dioxide. The process conditions in this process must be tuned so that the dominant reaction would be eqn (17) and not the reverse water gas shift reaction, eqn (14). Typical process conditions for this reaction are temperatures of 230°C and pressures up to 5 MPa. Within the reactor at these conditions an equilibrium yield is reached around 30%, and the reaction gasses have to be recycled to reach higher overall plant yields.111 | CO2 + 3H2 → CH3OH + H2O, ΔH = 50 kJ mol−1 | (17) |
Like the process to make synthetic hydrocarbon fuels, the FTS and Sabatier reaction, the synthesis of methanol can be carried out with relatively cheap transition metal catalysts. In the case for methanol, a catalyst using Cu/ZnO/Al2O3 is often used.112 Compared to the other CO2 based hydrogen storage molecules, the loss in hydrogen is lower but still amounts to 1 in 3 hydrogen molecules converted to water.
3.4.4 Formic acid for hydrogen storage.
Similar to the production of methanol, formic acid can also be produced from the hydrogenation of CO2. However, unlike the previously mentioned fuel production processes with CO2 feedstock, the reaction to FA does not have water as a waste product resulting in a theoretically 100% atom efficient process.113 | CO2 + H2 → HCOOH, ΔH = −32.2 kJ mol−1 | (18) |
FA is a widely produced chemical with global production capacities close to 1000 metric ton annually (2016).114 On a mass basis FA stores 4.3% of hydrogen; due to the high density of 1.22 kg m−3 the volumetric density totals 53 g L−1. The main production method for FA, however, is not eqn (18) but a process developed by BASF, but uses methanol as a starting product.115 In recent years, the production of formic acid by hydrogenation of CO2 is gaining more attention in academic research, Enthaler et al. reviewed different catalysts, homogeneous, heterogeneous and enzymatic catalysts for the production and dehydrogenation of FA.113 Sordakis et al., later wrote an analysis of the use of homogeneous catalysts for a sustainable method of hydrogen storage in formic acid. Most research in this field makes use of alkaline additives to the reaction system, which, when present in the formic acid end-product, lowers the hydrogen storage capacity of the FA and will require downstream purification of the hydrogen gas stream after dehydrogenation.116
Unlike the other CO2 based fuels, FA cannot be used as is in engine systems. Some researchers have focused on the use of Direct Formic Acid Fuel Cells117 but most common is the dehydrogenation of FA to hydrogen and CO2. This reaction however is not trivial, and catalysts have to be tuned to selectively perform the dehydrogenation over the dehydration reaction.116
| HCOOH → H2 + CO2, ΔH = 31.2 kJ mol−1 | (19) |
| HCOOH → H2O + CO, ΔH = 28.7 kJ mol−1 | (20) |
Like the production of FA from CO
2 the decomposition of FA has gained a lot of attention in recent years and there have been reports on the scale-up of the power systems fuelled by FA. Impressive work has been delivered by students of TU Eindhoven by building a 25 kW range extender for a hydrogen powered bus. Critical here was the selection of the catalyst, where long-term stability proved crucial over catalyst-efficiency. By scaling-up the technology they also discovered that CO concentration of the decomposition product increased, so an additional gas purification system was required. Additional problems with formic acid that came from the scale up were FA purity. The presence of solvents that remained in the reactor diluted the catalyst and in the long term caused the FA-decomposition reactor to overflow.
118
3.4.5 CO2 capture and storage.
An important note on the use of CO2-based fuels, is the availability of CO2. Although, emissions of CO2 are increasing globally, it is always a waste product and there is no large availability of CO2 at an industrial level that is required, certainly not at the scale for the current maritime fuel infrastructure.119 Due to the addition of oxygen upon combustion the mass of CO2 is roughly three times higher than the mass of the original fuel. Thus, the required CO2-recycling infrastructure would have to be three times as large on a mass basis compared to the current fuel infrastructure. There exist three strategies of CO2 recycling: (i) CO2 could be stored on board, but this requires purification systems of the exhaust gas to an unseen level120 (ii) atmospheric capture of the emitted CO2, which would mean that the system is entirely carbon neutral. However, atmospheric carbon capture is not expected to be developed at a large enough scale to effectively reduce CO2-emissions as required by IMO standards. Based on energy efficiency direct air capture for fuel production is also not considered an efficient process.121 (iii) CO2-Recycling from industrial sources, large scale industrial facilities could be retrofitted with CO2 capture technology which could then be recycled for new purposes. The usefulness of recycling of CO2-capture for the production of fuels, is however contested to have a long-lasting impact on the environment.122
3.5 Aromatic liquid organic hydrogen carriers
A final method that will be discussed, showing great promise for the maritime industry is the use of the so-called Liquid Organic Hydrogen Carriers (LOHCs). Originally, LOHCs were a class of liquid molecules, consisting of homocyclic or heterocyclic aromatic rings. These compounds have a moderate hydrogen storage density between 5 and 7.2 wt%, translating to the storage of between 50 and 60 g of hydrogen per litre. LOHCs can now more broadly be defined as “Liquids or low-melting solids that can be reversibly hydrogenated and dehydrogenated at elevated temperatures in the presence of a catalyst.”19 To avoid confusion, in this text we use the term aromatic LOHCs to refer to the class of molecules that store hydrogen in aromatic C–C double bonds. The volumetric storage density of aromatic LOHCs is only slightly lower than the density of liquid hydrogen (70 g L−1), but with the considerable advantage that the hydrogen is stored at room temperature.
Even though different aromatic LOHCs have been proposed in the literature, the overall process and process conditions are similar. Hydrogen storage in aromatic LOHCs is based on the reversible hydrogenation and dehydrogenation of carbon–carbon double bonds. Aromatic LOHCs are thus also hydrocarbon molecules, just like diesel, LNG and methanol, but since they are not combusted, they do not emit any CO2 as long as the stability of the carrier can be guaranteed.
The cycle of hydrogen storage proceeds through two reaction steps, the exothermic hydrogenation reaction, and the endothermic dehydrogenation to release hydrogen (Fig. 2).123
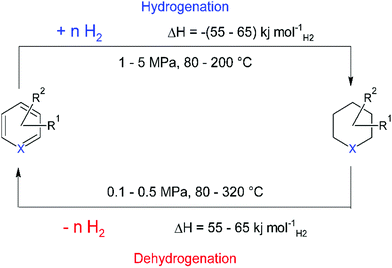 |
| Fig. 2 General cycle of aromatic LOHCs. | |
In both catalytic steps of the cycle, suitable heat management is crucial for both the extent to which hydrogen can be stored, and for the commercial deployment of this technology. For example, in the charging process of dibenzyltoluene (DBT), one of the most studied LOHCs, 65 kJ molH2−1 of heat is produced and must be removed to avoid thermodynamic equilibrium. The reverse is true for the dehydrogenation process; this process step requires around 27% of the energy of hydrogen (LHV) at medium temperature (200–300 °C) to release hydrogen from the carrier molecule. Key for the deployment of this safe and ambient conditions storage technology is the development of aromatic LOHCs with lower energy requirements. However, herein lies a great engineering challenge also; since only heat and no electricity has to be supplied to the system, there is the possibility of using waste heat streams to reduce the required energy and make the overall process a lot more efficient. Recent work has shown that combining high temperature SOFC with aromatic LOHC technology for hydrogen release leads to efficiencies of the overall system that are comparable to gaseous hydrogen storage.124
The properties for the three most discussed aromatic LOHC molecules are listed in Table 2. The physical, safety and practical aspects of using toluene, NEC and DBT as a hydrogen carrier have been reviewed by Preuster et al. and benchmarked by reaction enthalpy, boiling point, flash point, melting point, toxicity and gravimetric hydrogen content.123 For the shipping industry we limit the discussion of different aromatic LOHCs to the following three (dehydrogenated carrier names): toluene, N-ethyl carbazole (NEC), and dibenzyltoluene (DBT).
Table 2 Properties of selected aromatic LOHC molecules
Name |
MCH |
TOL |
H12-NEC |
NEC |
H18-DBT |
DBT |
Structure |
|
|
|
Properties |
Density (kg m−3) |
770 |
867 |
937 |
1158 |
909 |
1041 |
Gravimetric hydrogen capacity (wt%) |
7.2 |
5.7 |
6.2 |
Volumetric hydrogen capacity (g L−1) |
55.44 |
53.4 |
56.358 |
(De)hydrogenation enthalpy (kJ molH2−1) |
68.3 |
50.6 |
62 |
Melting point (°C) |
−127 |
−95 |
84 |
69 °C |
−58 |
−39 |
Boiling point (°C) |
101 |
111 |
281 |
378 °C |
371 |
390 |
Flash point (°C) |
−4 |
4 |
|
186 |
|
212 |
3.5.1 Toluene.
Toluene (C7H8) is one of the simplest molecules that is considered a viable option for aromatic LOHC technology (benzene (C6H6) has an even simpler structure than toluene, but due to the high carcinogenicity of this molecule it is not often considered a reliable option). When fully hydrogenated, toluene stores three moles of hydrogen, resulting in the storage of 7.2 wt% of hydrogen. Of all LOHC molecules considered in this review, toluene has the highest gravimetric capacity for hydrogen storage. However, since it is a small homocyclic molecule, it has a very high aromaticity, and this increases the energy needed for the hydrogenation and dehydrogenation processes. The temperature range of toluene and methyl-cyclohexane shows that both molecules are liquid at room temperature, but under the dehydrogenation reaction conditions (>250 °C), all reactants are in the gas phase, so additional processing of the gas flow is required, with an additional energy cost and processing difficulty.
Yearly a few million tons of toluene are produced by several production pathways. By far the most widely used process is the catalytic reforming of naphtha (low boiling point crude oil fraction) streams, 87% of all globally produced toluene is produced this way. Other methods of production are toluene separation from pyrolysis of gasoline during the cracking process to produce ethylene and propylene; this accounts for 9% toluene production. 2% of toluene produced is from by-products of styrene production and the remaining 1–2% is produced from coal tars.125 Current cost estimates to produce toluene are around $700–800 USD ton−1.
In order to have a functioning hydrogen storage system, two catalytic processes have to be reviewed, the hydrogenation and dehydrogenation step. The hydrogenation process of toluene is well studied in diesel fuel processing: the total aromatic fraction is decreased in hydrotreatment processing. A high aromatic fraction in diesel lowers the fuel quality and causes more air pollutants upon combustion.126 In the early 1990s limits on the fraction of aromatic compounds in fuels have been set and this sparked research for the hydrotreatment of fuels. The major difference between hydrotreatment of diesel fuels and hydrogenation of toluene is the purity of the aromatic stream. In hydrotreatment the reaction mixture is a blend of both alkylic and aromatic hydrocarbons with a significant concentration of nitrogen and sulphur, whereas the aromatic LOHC in theory consists of a pure compound at the start of the reaction. This translates into a difference in the operating parameters for the catalysts: catalysts for hydrotreatment are required to be highly stable to sulphur poisoning and are less optimized to work in the specific reaction conditions for aromatic hydrogenation. Hydrotreatment catalysts based on group VIII metals did show great promise for more specific hydrogenation of aromatic compounds, however, the tolerance of these catalysts to the sulphur containing diesel streams proved too low to be commercially viable.127 The group VIII metals have shown great promise in the field of toluene hydrogenation, and also for the reverse process of the dehydrogenation to release the hydrogen from the carrier.19 For fuel applications, especially in the maritime sector, efficient release of hydrogen from the carrier is required so a lot of research is being put into the development of new catalysts for this reaction.128–133
Toluene and methylcyclohexane however, have quite a low flashpoint, and this implies fire hazards when using this molecule in bulk as a hydrogen carrier medium. Furthermore, even though it is still present in present day fuels, the aromatic contents have been restricted in fuels due to the proven carcinogenic nature of small aromatic molecules. The Japanese company Chiyoda corporation has envisioned the first use of this chemical carrier as a hydrogen storage medium for large scale transport by ship between Japan and Brunei; this is one of the first large-scale maritime transport projects of hydrogen in an aromatic LOHC.
3.5.2
N-Ethyl carbazole (NEC).
NEC was the first molecule that drew large attention to heterocyclic aromatic compounds for hydrogen storage. The nitrogen atom in the cycle reduces the amount of hydrogen that can be stored but has the advantage that the enthalpy for dehydrogenation is lower compared to non-substituted aromatic cycles, resulting in a lower dehydrogenation temperature. From Table 2, it can be seen that the melting point of dehydrogenated NEC is higher than ambient temperatures and therefore it is a solid under normal conditions. Having a solid fuel on board of a ship is not very favourable, since the maritime industry is based on liquid fuels and this cancels one of the biggest advantages of LOHCs (it being a stable liquid that is easy to pump even at room temperature) compared to other hydrogen carriers. Solutions have been offered to deal with the high melting point of NEC, e.g. stopping the dehydrogenation at 90% has shown that the final products result in a liquid end product.134 In this way however, the total storage efficiency of NEC diminishes by 10% as well. The thermal stability of NEC is another issue that has to be dealt with; at temperatures above 270 °C the bond between nitrogen and the ethyl chain breaks.128 This has a twofold negative consequence: (i) the melting point of unsubstituted carbazole is much higher, around 274 °C and (ii) the free electron pair on the nitrogen atom is exposed, and this free electron pair has a high affinity for the noble metal catalyst, therefore causing deactivation. Another disadvantage of NEC is the low availability of the molecule; the annual production of this chemical is lower than 10
000 tons, and all of it is obtained from coal tar by distillation.18
3.5.3 Dibenzyltoluene (DBT).
In 2014 Brückner et al. published for the first time the use of dibenzyltoluene as a hydrogen carrier molecule.128 This molecule combines a large availability, being liquid in a broad temperature window and a low toxicity. The gravimetric capacity for hydrogen storage is slightly less compared to methylcyclohexane, but due to the higher density of H18–DBT the volumetric storage capacities are similar. DBT is made by aromatic substitution of two molecules of toluene on a central toluene molecule. Since it is a form of processed toluene, naturally the cost of toluene will be lower than that of DBT. Current estimates are that DBT is sold for a price of $3000 per ton. DBT is produced at a capacity of several thousand tons per year, and it is mainly used as a heat transfer oil, known as Marlotherm® SH.
Merging three toluene molecules into one new DBT molecule comes with advantages over base toluene. Firstly, the boiling point of the DBT becomes higher than the reaction temperature for dehydrogenation (Table 2). This means that within the dehydrogenation reactor only hydrogen is generated in the gas phase and this eliminates the need for further downstream separation of the hydrogen from the LOHC. Although, gaseous by-products from the decomposition of the carrier molecule can be present as well. The flashpoints of DBT and H18–DBT are also higher than that of toluene and methylcyclohexane, making these molecules less likely to be a fire hazard. DBT has a further advantage when looking at the toxicity profile, and is considered less toxic than NEC and toluene.123 Spills of DBT out of a ship in the ocean should still be avoided as the chemical is still a category 4 (lowest) danger for chronic aquatic toxicity in the MSDS.135 Due to its listed advantages, DBT will be used as the reference aromatic LOHC substance and compared with other hydrogen carriers for their use for maritime applications in the remaining text.
3.6 Solid state hydrogen storage
Currently, there have been several methods researched that store hydrogen in solid materials. These materials can be metallic structures that work like hydrogen sponges,136–138 or they can be powders that release hydrogen upon contact with water or increased temperatures.139–142 There has been a lot of research put into different solid materials for hydrogen storage, and it is not in the scope of this review to comment on all these methods, but we will select those that show promise for hydrogen storage in a maritime environment.
3.6.1 Metal hydrides.
In chemistry, most metals have a certain affinity to react with hydrogen, to form metal hydrides. Due to the often-large difference in atomic mass between the metal and the hydrogen atoms however, these complexes often have very low gravimetric storage capacities. The most sensible thing to do in this regard, is to use the lightest metals as storage materials, but these hydrides are often most stable and thus require the most stringent conditions to release the hydrogen again. The lightest metal, lithium, can form the LiH that contains 12.5 wt% of hydrogen, however the dehydrogenation temperature of pure LiH is 944 °C.143 In the selection of metal hydrides there is a trade-off between dehydrogenation temperature and mass fraction of hydrogen to be stored. An example that showcases this trade-off is MgH2. This monometallic hydride is made from a metal with a medium atomic weight, magnesium (MW 24.3) and has an increased, but moderate dehydrogenation temperature. MgH2 stores 7.7 wt% of hydrogen and roughly 110 gH2 L−1 and releases it at a temperature of 300 °C.144,145 Mg does have the advantage of being the fifth most abundant metal in the Earth's crust, which lowers the price for Mg based storage. One of the largest disadvantages of MgH2 is the slow kinetics of the hydrogenation reaction: the formation of the hydride is a surface reaction, so a MgH2 layer is formed first on the Mg metal fraction in the storage vessel. This layer, however, is a significant barrier for further diffusion of hydrogen into the metal, and further hydrogenation slows. An offered solution is to then use smaller particles of magnesium so hydrogen does not have to penetrate so deep in the metal, but research has shown that the cyclability of these small particles is low due to agglomeration.
In current research there is a lot of focus on the use of the so-called intermetallic compounds that are described by the general formula ABxHy, with A being either a rare earth or alkali (earth) metal and B a transition metal. An example of a metal hydride that has been used in a shipping application is the use of a TiMn2-like compound. This is a so called laves phase alloy.146 A publication by Bevan in 2010 described a canal boat operational in the UK, that was able to store 4 kg of H2; the exact composition of the storage material was described as Ti0.93Zr0.05(Mn0.73V0.22Fe0.04)2. The practical hydrogen storage capacity of this material was determined to be around 1.4 wt%. Hydrogen could be pressurized in this material at 3 MPa and released by lowering the pressure and adding cooling water at 9 °C. High cost of this system was however also mentioned as a downside of this promising storage system.147 This type of storage system, of a metal hydride sealed in a pressure vessel is also known as a hybrid hydrogen storage vessel.148
Another type of metal hydride that is under research is the class alanates; these are complex hydrides with the [AlH4]− anion, of which NaAlH4 is an interesting compound since it contains aluminium and sodium the no. 1 and no. 4 most abundant metals in the Earth's Crust respectively. The complex metal hydrides do not release all the hydrogen in one step, but it occurs in a cascade reaction when exposed to elevated temperatures.
| 3NaAlH4 → Na3AlH6 + 2Al + H2 | (21) |
| 2Na3AlH6 → 6NaH + 2Al + 3H2 | (22) |
The reaction no. 21 takes place in a temperature range between 185 and 230 °C, reaction 22 at 260 °C. As can be seen from the reaction equation, one hydrogen atom per alanate stays bound to sodium, forming NaH; this compound does not decompose at temperatures below 425 °C. For practical applications, NaAlH
4 thus only has 5.6 wt% of the theoretical 7.4 wt% available for storage. NaAlH
4 can store around 75 g
H2 L
−1 of which roughly 63 g L
−1 are accessible.
149 NaAlH
4 on its own is deemed an irreversible hydrogen storage carrier, however the addition of other metals as a catalyst (titanium) has shown that the alanate species does regain its hydrogen storage capacity from direct hydrogenation at increased pressure.
3.6.2 Boron-based hydrogen storage.
A second class of solid hydrogen storage materials is those based on boron. This element is the 5th lightest in the period system and classified as a metalloid, meaning that it has both metallic and non-metallic properties. In the literature the two most prevalent forms of boron-based hydrogen storage materials are NaBH4 and NH3BH3. Both these materials have very high hydrogen capacities >10 wt%; this is due to the lightness of boron while still being able to store many hydrogen atoms.
NaBH4, with a hydrogen capacity of 10.8 wt% and roughly 125 gH2 L−1, has a high decomposition temperature (530 °C)150 but it has the advantage that it decomposes in water releasing hydrogen according to the reaction:151
| NaBH4 + H2O → 4H2 + NaBO2 | (23) |
As can be seen from the reaction in
eqn (23), there are four moles of hydrogen released while only two moles of hydrogen are stored in the boron storage material, the additional two moles of hydrogen originate from the water in the hydrolysis reaction. With proper water purification this could be highly beneficial on a ship, as without the weight of water the hydrogen storage capacity of NaBH
4 would total 21 wt%. This water however is not added in stoichiometric quantities, but it must be added in excess, due to the low solubility of NaBH
4 and the even lower solubility of NaBO
2 (0.282 kg L
−1). Furthermore, the formation of NaBO
2 increases the pH of the reaction medium, which inhibits the further hydrolysis of the NaBH
4. To deal with this self-inhibition of the reaction, catalysts have been developed, usually based on cobalt.
152–154 On the side of hydrogen release NaBH
4 has many advantages for hydrogen release, but the reverse reaction is very difficult to perform efficiently. The boron–oxygen bonds are very stable, and thus difficult to rehydrogenate directly. A method that is used to rehydrogenate the boron–oxygen bonds is to mix it with MgH
2 in a ball mill; this however shifts the problem of rehydrogenating the boron to the hydrogenation of magnesium.
The other boron compound that is often seen as a promising hydrogen storage material is NH3BH3. This compound has the highest gravimetric capacity of hydrogen stored, 19.4 wt% and volumetrically close to 180 gH2 L−1.149 This is the highest storage capacity of all non-CO2-based hydrogen storage media. To release the hydrogen out of the NH3BH3 there exist two different strategies. One could opt to release the hydrogen by supplying heat. Like the alanates this is a step wise process and the moles of hydrogen released depend on the temperature.
The reaction in
eqn (24) takes place starting at 100 °C when the NH
3BH
3 starts to liquefy, and the reaction in
eqn (25) requires temperatures of 155 °C and higher. Releasing the hydrogen from the final mole of hydrogen which would form boron-nitride requires temperatures above 500 °C.
155
Like the NaBO4, described above the dehydrogenation of NH3BH3 can also be achieved by a hydrolysis reaction in an acid solution at room temperature. The proposed reaction equation for this process is:156
| NH3BH3 + H+ + 3H2O → 3H2 + B(OH)3 + NH4+ | (26) |
From the reaction equation it can be seen that all hydrogen atoms stored within the NH
3BH
3 compound can be released. Next to acids, the dehydrogenation can also be achieved by metal catalysts.
156
It is important to note that the final products of the dehydrogenation reactions, both B(OH)3 and NHBH (which is a polycyclic mixture of its oligomers), are very stable products. The main limitation that is sighted for the use of NH3BH3 as an energy storage medium is the recycling of the product. The boron–oxygen bonds of the hydrolysis reaction are limited by the same regeneration reaction as for NaBH4, described above. The stability of the boron–nitrogen bonds that result from thermolysis of NH3BH3 are also not suitable for direct hydrogenation strategies and several strategies for the hydrogenation of these bonds have been described, but only at very low TRL levels.157–159
4 Energy comparison for hydrogen storage methods
In the next section the different hydrogen carriers are compared based on the production and storage energy required for the entire process. The conversion efficiency of the fuel to useful work is neglected; we only look at the energy required to supply either an FC or an engine with the equivalent energy of 1 kg of hydrogen.
4.1 Energy requirements for storage
First the base case energy requirements for different energy carriers is discussed with the following assumptions:
• Hydrogen is produced via alkaline electrolysis with an efficiency of 55 kW h per kg hydrogen. This assumption was made to showcase the energy requirements for hydrogen produced with the current most industrially relevant zero-CO2 emission pathway.
• Storage losses e.g. evaporation of cryogenic liquids are neglected since ships can sail on these boil-off gasses.
• CO2 sources are fossil fuel power plants, where carbon dioxide is captured with efficiencies according to Lucquiaud et al.160 This method was chosen since it is most likely to be the first method to produce relevant quantities of CO2.
• Hydrogen release processes do not use any external heat sources and require energy stored in the hydrogen carrier itself, i.e. the worst-case scenario for these fuels. This extra energy is calculated as additionally produced and stored hydrogen.
• For simplicity, the only side products that are assumed are in the FTS, here a 50 wt% conversion efficiency of diesel fuels from CO2 in the FTS-production process, is assumed. FTS-diesel is assumed to consist only of C12H26, the weight averaged most common molecule in diesel.161
• Chemical processing energy of the metal-based hydrogen carriers is the only required energy to pressurize hydrogen according to Andersson and Grönkvist (2019).162
• Boron based materials have been neglected since no relevant energy efficiency of the overall storage cycle could be found.
The results of the calculations with these assumptions are seen in Fig. 3. First and foremost, the production of hydrogen via electrolysis is the most energy intensive process in every storage method. This means that any loss of hydrogen in the overall production process is detrimental to the overall energy efficiency of the storage process. This is the reason why the energy requirements for FTS diesel are so high: firstly, due to the reverse water gas shift reaction already one mole of hydrogen is lost per mole of CO2 captured. Secondly in the reaction for long alkane chain diesel, roughly half of the hydrogen is lost to the formation of water in the reaction. Thirdly, since the FTS always generates a distribution of hydrocarbons and never pure long chain hydrocarbons, there is loss inside product formation as well in this case. Here we assumed 50% efficiency of the FTS towards carbon molecules found in diesel.
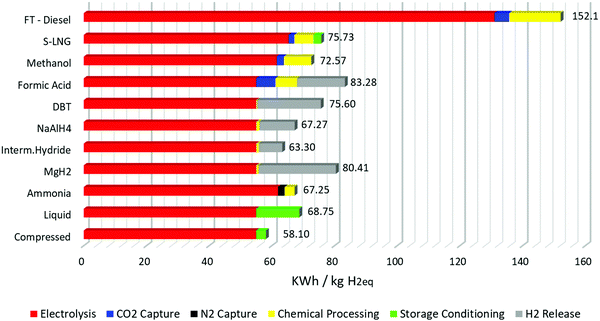 |
| Fig. 3 Energy comparison for the storage requirements for the selected hydrogen storage carriers. The total sum of the values of these carriers is the total energy required (in kW h) to produce and store 1 kg of hydrogen (=33 kW h, LHV) with all the assumed product and energy losses. | |
The overall energy storage cycle for S-LNG, methanol and DBT is roughly half that of the storage requirements for FTS. The similarities in energy requirements for methanol and S-LNG are noteworthy; from the analysis it is shown that even with the much higher loss in hydrogen in the Sabatier process compared to methanol synthesis, the total energy efficiency overall is still very similar. This is because the stored energy per kilogram of S-LNG is much higher than that of methanol, compensating to a large extent for the hydrogen losses in the storage processes. For hydrogen storage in DBT the overall efficiency is of the same order as that of S-LNG and methanol. This result is obtained by assuming that all the energy needed to release the hydrogen is stored in the DBT itself, i.e. without any external waste heat recovery. From Fig. 3 it can also be derived that if the energy for the dehydrogenation is provided by another heat source, the overall efficiency of this storage process would greatly increase to the point where it rivals compressed hydrogen storage. This has been proven experimentally by linking a high temperature fuel cell with hydrogen storage in DBT.124 The same can be said for FA, although heat integration with FA should be achieved easier than with DBT, since the decomposition of FA is possible at lower temperatures. With suitable heat integration the energy efficiency of FA would be the lowest of the CO2-based storage methods. Without it the energy efficiency is drastically lower. Also, important to note in FA production is the higher energy need of CO2-capture in the overall process, since only one hydrogen molecule is stored in FA, compared to 2 molecules in methanol or S-LNG.
In the metal hydrides there is a large difference in the overall energy efficiency of the storage process and it follows the trend of the ease of dehydrogenation. Of the three discussed examples, MgH2 has the highest temperature requirement for the dehydrogenation (>300 °C) and requires the highest energy overall. The intermetallic hydrides release the temperature at much lower energies and even show the second lowest dehydrogenation energy overall. The value reported here is also the average of reported dehydrogenation energy for intermetallic hydrides, so energy efficiency for this storage material could be even higher.162 It has to be noted that for the three cases the only energy required for the storage process was the compression of the gas to suitable pressure; no cooling or other processing was included.
Ammonia and liquid hydrogen also have similar energy efficiencies when comparing the storage requirements, which are about 10% lower than those of the other high-density liquid storage methods, S-LNG, methanol and DBT. Of these two, ammonia production has the lowest energy requirements, and since this process is fully developed already at an industrial scale, the production of green ammonia can serve as a good benchmark figure for the comparison of other hydrogen storage methods. In the energy analysis of Fig. 3 an energy cost of 13.75 kW h per kilogram of hydrogen is assumed for the liquefaction. This is an average value for the liquefaction energy of hydrogen in today's industry.74 However, there is a lot of work going on to lower this energy need below 7 kW h kg−1 of hydrogen, as demonstrated by the European Union's IDEALHY project.163 The findings for this project proved, however, that in order to reduce this process to 6 kW h kg−1 the coolant had to change from liquid nitrogen, a widely available resource, to a mixture of liquid helium and neon,76 which are considered rare.164 Liquid helium was required because of its boiling point, which is much lower than that of liquid nitrogen (−269 °C vs. −196 °C).
Compressed hydrogen storage even at 700 bars currently remains the most energy effective way to store hydrogen. But handling gasses comes with its own pitfalls that are especially relevant in the maritime industry as will be discussed in Section 5.
4.2 Space and weight requirement for fuel storage
While the energy balances provide a good overview of the total system efficiency for the use of hydrogen fuel, this is not the only thing that will determine whether a certain energy storage system will break through in the industry. A very important aspect is the hydrogen-based fuel's energy density, as this will determine storage space, weight requirements and fuelling (or bunkering) time.
In Fig. 4 different energy densities are shown both out and in dedicated fuel tanks required for proper storage. From this figure it becomes evident that hydrocarbon fuels are still superior to non-hydrocarbon fuels in terms of energy storage densities, even with the weight and volume restrictions of the tanks. Calculating the energy densities of the selected hydrogen storage methods within the tank has interesting implications. Firstly, the use of dedicated LNG tanks shifts the volumetric storage capacity of LNG down to be on par with that of methanol and ammonia. However, per kilogram, LNG remains superior over the latter two storage methods. A large shift in system efficiency by adding the weight of the tank is seen for compressed and liquid hydrogen. This extra weight is necessary to keep the fuel under the right conditions for processes, i.e. 70 MPa or −253 °C respectively. For the storage system at 70 MPa, it has to be highlighted that this is the most advanced compressed hydrogen system available, and that this system comes at a high cost of 600–800 $ per kilogram hydrogen stored.67,165 Based on energy density, DBT as a hydrogen storage system is still almost twice as efficient per litre of fuel stored when compared to the most advanced compressed hydrogen systems. The energy density is also close to that of liquid hydrogen, but with the added benefit of having stable storage at ambient temperatures. In order to properly function, DBT would require an extra buffer tank on the ship, since the loaded and unloaded forms of the LOHC cannot be stored in the same tank. This buffer tank would be empty when the ship sets sail and is filled with unloaded LOHC during the voyage; with this buffer system there is no need for mixing the charged and uncharged DBT. In the calculation for Fig. 4, an 11th empty tank is included for 10 filled tanks (see Fig. 5). This figure represents a schematic division to clarify the strategy for DBT storage on a ship; in a real case, the tanks will have to be designed and distributed to avoid any effects from sloshing. Of all the hydrogen carriers discussed in this analysis formic acid shows the lowest gravimetric and volumetric storage density of all. However, within tanks formic acid is on par with DBT for volumetric hydrogen storage capacity, showing a greater storage capacity per litre than compressed hydrogen. This shows the importance of the weight of the tank rather than the energy storage capacity of a pure compound. Fig. 4 also shows a comparison between the solid-state hydrogen carriers and the liquid phase carriers. Based on the intrinsic weight and volume storage efficiency all outperform DBT and formic acid, but the additional weight required for storage systems of the solid-state carriers in a working system lower the storage efficiency of the carriers. However, these systems are often developed on a smaller scale and further research is needed for the development of larger solid-state hydrogen carriers for a better comparison.166,167 The NaAlH4 and AB2 intermetallic hydride are contained here in vessels designed to withstand the pressure required for hydrogenation, the hybrid hydrogen storage vessels. For NaBH4 the practical hydrogen capacity is limited by the spent fuel rather than by the fresh fuel. This is caused by the fact that the spent fuel is an aqueous solution so the weight and volume of the water must be added, since ships will have to keep the spent fuel on board. To reduce the weight and volume as much as possible, it was assumed that a volume exchange tank is used, as described by Kim.168 No reliable sources were found on the practical storage density of MgH2 and NH3BH3 and these had to be omitted in this analysis.
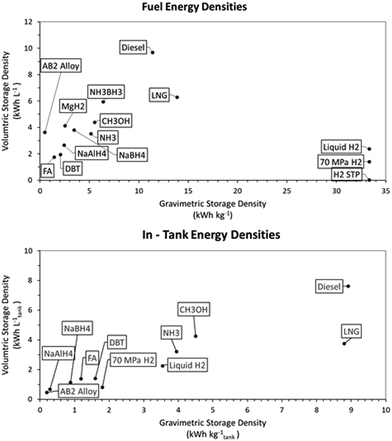 |
| Fig. 4 Energy densities of the selected hydrogen storage methods: (A) the net energy density and (B) the gross energy density with the fuel stored in suitable tanks. | |
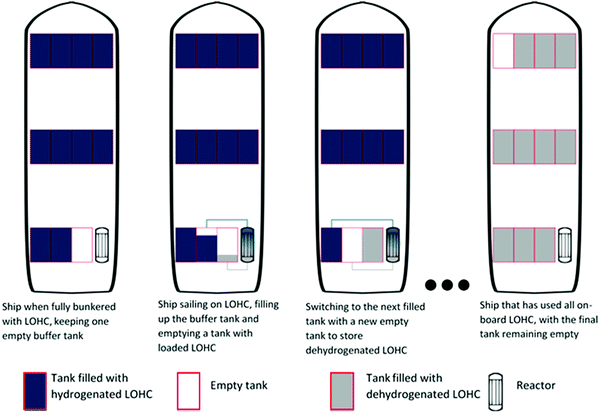 |
| Fig. 5 Use of a buffer tank for LOHC fuel systems on board of a ship. | |
Unfortunately, no matter which hydrogen storage method is selected, the energy densities both by weight and by volume of diesel fuels (renewable or not) cannot be reached with other alternative fuels. This means that switching the fuel type will always increase required weight and volume on board of a ship. Luckily, there are two factors in maritime shipping that counteract this negative effect of increased storage volume. Firstly, ships usually have more fuel on board than is required for the chartered voyage. This means that if the bunker frequency of a ship is increased, the decreased energy content stored within the tank is less of an issue. Secondly, for large seagoing vessels in the range of 100
000 tons deadweight, the fuel tank only takes up about 1–2% of the total ship weight. So even if an increased fuel capacity is needed when sailing on hydrogen for longer voyages, the total extra weight that the fuel tank will take up should not be too extensive.
5 Challenges for hydrogen storage in the maritime industry
5.1 Current state of hydrogen technologies in the maritime industry
Compressed hydrogen is currently being used to showcase the potential of hydrogen in the maritime shipping industry. The Belgian ship owner company CMB has developed the Hydroville, the first ever seaworthy passenger vessel that sails on dual-fuel hydrogen combustion engines.169 The ship owner more recently started a joined venture called BeHydro with an engine manufacturing company, ABC engines.170 This demonstrates more than anything the interest of ship owners in H2-fuelled engines, as well as their potential. Ships using fuel cells and hydrogen have also been demonstrated but to a smaller extent, examples are the Hydra-ship and the Duffy–Herreshoff water taxi and the Yacht XV 1.171 On the Scottish Orkney Islands an innovation project called HySeas III is using hydrogen fuel cell powered ships as ferries.172 A research project at the TU Berlin, investigated the use of hydrogen in a power system for a towboat, called the RiverCell-Elektra. Near Hamburg another ship, the ZemShip Alsterwasser, a small passenger vessel, was also used to showcase the potential of hydrogen as a shipping fuel. Other examples of passenger vessels that were fuelled by hydrogen in recent years include the Nemo H2 in Amsterdam, the Hornblower Hybrid and the Hydrogenesis in Bristol, the MF Vlågen173 and the SF-Breeze, a hydrogen powered high speed ferry operating near San Francisco.174,175
5.2 On-board end use
Hydrogen is often linked to fuel cells in a power system, but hydrogen can also be combusted in a diesel or a gas engine. In these combustion engines, hydrogen can be the sole fuel in these engines (mono fuel), or it can be used in a dual fuel system.176 Some storage methods also require dedicated reactors to release the hydrogen prior to use in the engine or fuel cell. This is discussed in Section 5.4. It is important to note that the end-product of the hydrogen is always water, regardless of whether it is combusted or used in a fuel cell. From an emission point of view, the largest difference between fuel cells and combustion engines is the emission of NOx.177 Another difference is that fuel cells have a higher energy efficiency than combustion engines, but they have a much higher cost and usually a lower lifetime.178,179 In the current day maritime industry, the fuel cell is a novelty used mostly in passenger vehicles while combustion engines have been used for decades in the shipping industry.
5.2.1 Fuel cells.
Hydrogen is the fuel that is mostly associated with fuel cells (FCs). The exact workings of fuel cell systems are outside the scope of this review, but in general fuel cells operate in a reverse direction as an electrolyzer system. This means that the fuel is split into different components at the electrodes of the fuel cell and electricity is generated.180 The use of fuel cells for main ship propulsion thus also requires the electrification of a ship whereas now mechanical power delivered by an engine makes the rotor move. Several types of fuel cells exist: the proton exchange membrane fuel cell (PEMFC), the molten carbonate fuel cell and the solid oxide fuel cell. Of these systems the PEMFC system is one of the most used fuel cells, but it is limited by the need for very pure hydrogen and can be poisoned by ppm levels of CO or ammonia.181,182 This requires extensive purification of the hydrogen from storage methods like ammonia, methanol, formic acid, ammonia borane and DBT. At higher temperatures the fuel cell becomes more resistant to poisoning and this is why systems like the molten carbonate FC and the solid oxide FC are under research.183 There has also been progress made to make the PEMFC more resistant to higher temperatures by making use of more resistant membranes.184 The molten carbonate and solid oxide fuel cell operate at such high temperatures that hydrocarbons and ammonia are decomposed within the fuel cell and these chemicals can be used directly in these types of fuel cells.185 Due to the high temperatures in the fuel cells, the formation of NOx can still be an issue.186 For ammonia, formic acid and methanol fuel cells are being researched that only operate with these specific fuels.187–189
5.2.2 Combustion engines.
Next to fuel cells, hydrogen can also be combusted in engines. The main problem with hydrogen is the high auto-ignition temperature which complicates timed and efficient combustion in an engine cylinder compared to hydrocarbon fuels.190,191 Hydrogen in a mono-fuel operation is possible is spark-ignition engines; it can be used in compression engines only in dual-fuel mode with a hydrocarbon pilot fuel.176 Of the hydrogen storage methods discussed in this work, the FTS-fuels, S-LNG and methanol can be used directly in combustion engines (as is done on ship's today). Ammonia can only operate in a dual-fuel model with a pilot fuel in engine systems. This is due to the high auto-ignition temperature and narrow flammability range. Work done at the university of Pisa has shown that ammonia can be partially split into nitrogen and hydrogen by an integrated reactor and this hydrogen can then serve as a pilot fuel for ammonia combustion.90,192 Formic acid is often seen in the exhaust gas of methanol engines but is too oxidized to function as an effective fuel in combustion engines. Recent work by Sarathy et al. showed one of the first studies on the burning charateristics of formic acid in air and mixed with hydrogen and CO2. They showed that for effective combustion of formic acid, hydrogen in pure form had to be present in the combustion mixture.193 For LOHCs, combustion engines provide the advantage of higher tolerance to fuel impurities but the reduced power efficiency compared to fuel cells means that more hydrogen has to be released from the carrier, which will have to be weighed against the energy needed for purification. In Table 3 the properties of the discussed fuels can be found and compared to one another. Table 4 gives an overview of which types of storage methods are compatible with which power systems and if there is a conversion step required.
Table 3 Properties of selected fuels
Fuel |
Density (kg m−3) |
Lower heating value (MJ kg−1) |
Auto-ignition temperature (°C) |
Flammability in air (vol%) |
Lam. flame velocity (m s−1) |
Ref. 194.
Ref. 195.
Ref. 194.
Ref. 196.
Ref. 90.
Ref. 197.
Ref. 198.
Ref. 114.
Ref. 199.
|
Diesel |
837a |
43.25 |
316 |
1–6 |
0.867b |
LNG |
443.5c |
50 |
540 |
5.3–15 |
0.38d |
Hydrogen |
0.082e |
120 |
571 |
4.7–75 |
3.51 |
Methanol |
790f |
20.09 |
465 |
6.7–36 |
0.54g |
Ammonia |
0.730c |
18.8 |
651 |
15–28 |
0.015 |
Formic acid |
1220h |
5.54h |
520 |
18–15i |
|
Table 4 Compatibility of hydrogen storage methods with power systems
Fuel |
High temp. FC |
LT PEMFC |
Specific low temp. FC |
Compression engine |
SI engine |
Diesel |
Conversion step |
No |
No |
Mono-fuel |
No |
LNG |
Direct use |
No |
No |
Dual-fuel |
Mono-fuel |
Hydrogen |
Direct use |
Yes |
— |
Dual fuel |
Mono-fuel |
Methanol |
Direct use |
Conversion + purification |
Yes |
Dual fuel |
Mono-fuel |
Formic acid |
Yes |
Conversion + purification |
Yes |
No |
No |
Ammonia |
Yes |
Conversion + purification |
Yes |
No |
Dual fuel |
LOHC |
Conversion |
Conversion + purification |
No |
Conversion dual fuel |
Conversion mono fuel |
5.3 Bunkering ease and on-board use
An important factor in selecting the shipping fuel of the future is the ease at which it can be brought on board and handled on the ship. In the next section we evaluate the selected storage forms based on their bunkering ease and on-board use. In the shipping industry, certain ships can be fully fuelled or bunkered with several thousand cubic meters of heavy diesel fuel in a matter of a few hours. Typically, this is carried out by having a so-called bunkering ship navigate itself right next to the ship, so the loading of the fuel can be done while cargo is being loaded or offloaded from the ship to the shore. For compressed and liquid hydrogen there are already several projects underway that investigate the possibilities of hydrogen bunker facilities.173
5.3.1 Compressed hydrogen.
With compressed hydrogen the difficulties in storage are two-fold. On the one hand, the storage in gas cylinders results in rather low weight and volume densities of the overall system. So, this will require large parts of the ship's deck and its hold to be used for the storage of the cylinders. Another issue is the slow fuelling/bunkering time that is inherent to transporting a low-density gas. For the automotive industry, a specific fuelling protocol has been developed for fuelling hydrogen gas at 70 MPa, the SAE J2601 Protocol. This ensures safe operation of hydrogen fuelling for cars; the hydrogen is cooled down to −40 °C at first so no heat management in the car is needed and the fuelling speed is limited in the range of ∼1 kg of hydrogen per minute.200 This protocol is well suited for operations in the automotive industry were 1 kg of hydrogen corresponds to a driving range of around 100 km.81 With the type IV polymer tanks, the temperature of the gas should not exceed 70 °C for safety reasons and therefore slow and chilled bunkering of the gas is required.201 For the Oakney Island ferries, a compressed hydrogen bunkering system has been described and assessed for safety. However, in this project it was opted to go for hydrogen storage at 35 MPa rather than 700 MPa. The total bunkering facility was sized at 2 MW or 800 kgH2 daily.202 A large seagoing ship would require a few 100 tons of hydrogen for relevant operation and the fuelling at this rate would take several weeks or a very high number of nozzles. Attaching and releasing this large number of nozzles to a ship would be a very challenging and time-consuming operation on its own.
Another concept of bunkering could be to make use of hydrogen tanks mounted in a standard 20- or 40-foot container, which can then be loaded on a ship. This is referred to as cassette-type fuel systems.173,203 This might be an option for smaller ships like ferries, but for large ships the time it would need to load and unload all these containers would drastically elongate the port calls.
5.3.2 Liquid hydrogen.
To date, the largest operations that have used liquid hydrogen as a fuel are in the field of aerospace programmes like that of the space shuttles. A lot of research on the use and storage of liquid hydrogen found today is still based on the aerospace industry.78,204–207 The largest problem with liquid hydrogen fuelling processes is the low temperature at which the fuelling process has to take place and the subsequent evaporation of liquid hydrogen. Specific insulation materials are required for the tank materials to keep the heat flux into the tank as low as possible.208 NASA has reported that for the space shuttle launch, 45% of purchased liquid hydrogen was lost in the process chain, before even reaching the fuel tank of the space shuttle. 12.6% of this was lost due to transport from the liquid hydrogen carrying trucks towards the large storage tank, and 20.6% of the liquid hydrogen was lost from the onsite storage tank to the space shuttle fuel tank.209 Since the liquefaction process for hydrogen is an energy intensive processes the loss of liquid hydrogen must be reduced as much as possible to keep the overall energy efficiency of the process as high as possible.
Before ports can supply ships with enough liquid hydrogen, an entirely new fuel infrastructure will have to be built, without a lot of experience from handling liquid hydrogen. Currently, it is not even transported as a commodity on the ocean, although Kawasaki is looking to change this with their current HySTRA project.85 The development of a liquid hydrogen port is still a significant challenge before liquid hydrogen can be used globally as a fuel.
Although on board and in port, lessons can be learned from the use of LNG as a fuel. The development of LNG fuelled ships can serve as a baseline for the use of hydrogen ships.175 Although the challenges with hydrogen ships will be even greater since the liquid is about 90 °C colder and the energy density a factor of 2 lower, so colder and more liquid will have to be stored compared to LNG. The fuel of a ship is usually divided into multiple smaller tanks, but for each of these tanks, the hydrogen will evaporate, creating partially filled hydrogen tanks. In each of these tanks, sloshing of the liquid hydrogen is possible, with stability issues of the ship as a consequence.210
5.3.3 Ammonia.
Compared to liquid hydrogen, ammonia has a few significant advantages for its use as a shipping fuel. For one, the energy density of liquid ammonia is higher than that of liquid hydrogen, under less stringent conditions. Moreover, the production and transport of ammonia has been developing for over a century now and the shipping of this compound is well developed. Safety protocols have also been written to lower the risks of accidents related to this still toxic chemical.
5.3.4 Synthetic diesels.
Since the synthetic diesel fuels obtained by FTS are in a large part a cleaner alternative to currently used diesel fuels, the challenge with these fuels is thus not so much in getting them on board of the ship but mostly on the production side.
5.3.5 Synthetic LNG.
LNG has been promoted as a lower carbon alternative fuel for the shipping industry for many years now and several ships have been developed that sail on LNG. Chemically and physically there is no difference between S-LNG and LNG, so the developments that have been made for LNG ships are preserved for S-LNG ships. A ship sailing on LNG is not the current standard, but progress has been made in this field in the last few decades. Shipping of LNG as a commodity is well established as the first tests with LNG shipping date back already to 1959,211 and LNG is currently shipped in vessels with a carrying capacity of more than 100 000 m3.212 For the past 40 years, LNG carriers have been equipped with engines that allow them to sail on boil-off gasses in a dual-fuel operation with diesel fuels. The first ship built to sail on LNG as a main propulsion fuel was launched in 2000 and by 2017 over 117 LNG powered vessels were in operation.8 This still only accounts for roughly 2% of total bunkers in the shipping industry.213
For on-board use of LNG, either fossil or synthetic, there is still the issue of methane slip from the engine. Methane has a greenhouse gas warming potential that has 25 times the 100-year global warming potential of CO2,214 so emissions of methane to the atmosphere are detrimental to the overall environmental benefit of using LNG. Methane-slip in LNG powered engines arises from the unburned fuel in the engine or from venting the engine pipes after shutting down the engine.215
5.3.6 Methanol.
Like ammonia, methanol is one of the most produced and shipped chemicals in the world. In 2015 more than 70 million tons of methanol were produced globally. As such, there is already a widely developed infrastructure available to handle and ship methanol which makes it fit for its further development as a fuel. Since methanol is liquid at ambient temperatures there are little changes required to the existing bunkering infrastructure.
Historically, methanol has already been used as a fuel additive to enhance engine performance. But more and more testing is being carried out on using pure methanol as a fuel. Typically, methanol is seen as a fuel that matches well with SI engines, due to the high auto-ignition temperature and the high heat of vaporisation. As discussed above, SI engines are not widely used for systems larger than cars. As such, development on this side is needed. Compression ignition engines that use methanol are being developed, but they require more changes to use methanol as efficiently as a fuel, and currently, CI engines only run on methanol diesel-dual fuel operation. The most famous case of methanol in the shipping industry is the Stena Germanica, a 1500 passenger ferry ship that has an especially adopted four stroke medium speed engine that uses methanol together with MGO as a pilot fuel.197
Storing methanol on board also offers an interesting advantage over other fuel types; due to its low toxicity to marine life (see Section 5.5) methanol could be stored inside the double hulls of ships. Since methanol poses no danger to marine life there is no environmental risk if methanol spills into the ocean and therefore it should be possible to store it in the available space of the hulls. Methanol, however, is considered a low flashpoint fuel according to the IMO so they will have to agree with future regulations in the International Code for Ships using gases and other low flashpoint fuels, the IGF code.
5.3.7 Formic acid.
Formic acid has not been tested as a hydrogen storage carrier on board of ships, but has made headway as a potential fuel for busses, notably in the project at the TU Delft. In their project they stated that pure formic acid was corrosive so minor changes to fuel infrastructure were needed compared to current-day diesel infrastructure.118 For the maritime industry to sail on formic acid, there will have to be a new bunkering infrastructure built that can withstand the corrosive nature of formic acid, but no big changes in day-to-day operations are expected.
5.3.8 Dibenzyltoluene.
The liquid properties of DBT are very similar to those of diesel fuels; this is again beneficial for the development of a bunkering infrastructure since most of it can be kept in place. The downside of using DBT is that it is not a fuel on its own but merely a carrier. This implies that the empty carrier will have to be offloaded and stored on the ship next to the empty carrier. Currently, the emptying of ship fuel tanks to facilities on shore is not a standard practice and so new protocols will have to be developed for this process.
The charged and uncharged DBT are miscible with each other, but for greater reactor efficiency the fraction of uncharged DBT with the DBT mixture has to be reduced. It is thus ill-advised to store the liquids in the same tanks at the same time. Physically there is only a small difference between the charged and the uncharged carrier (the former having the lowest density), and the same type of storage tanks can be used. A possible concept to store the DBT on board is having multiple fuel tanks on board, but with one spare tank that is not filled in port and that can serve as a buffer tank (see Fig. 5). When a system with n tanks is assumed, 1 is kept empty but will be filled with unloaded DBT after it passes from the reactor and must be kept on the ship. While the nth fuel tank is being filled the (n − 1)th is being emptied and this will serve as the new storage vessel when the (n − 2)th tank is being emptied etc. A system like this will require an intricate system of piping and pumping, like cleaning-in-place systems used in the pharmaceutical and food industries. A more significant challenge with the use of LOHCs will also be the development of a reactor type that can withstand the conditions at sea (see Section 5.4).
5.3.9 Solid-state hydrogen storage.
Before the invention of diesel-powered ships, coal was the fuel of choice in shipping, so although there is a precedent of solid-state energy carriers in the maritime industry, the handling of solid-state hydrogen carriers is significantly different from coal. In essence, there are two methods to fuel a ship using solid state hydrogen carriers. Charged carriers can be either brought on board pumped as a solution or as powders (but sealed away from air and moisture) or it could be possible to bring the hydrogen on board in pure form and to let it react with the hydrogen carrier in the storage tank.
The first method to bring the hydrogenated carriers on board, also requires an offloading of the spent fuel, like it is required for DBT. This method is required for all the storage media that cannot be hydrogenated directly but require additional chemical processes for the hydrogenation. This is the case for the boron-based storage methods and likely also for MgH2. This chemical is reported to be very slow to hydrogenate (even slower than pressurized gas phase bunkering). In the literature hydrogenation of magnesium is often carried out via a ball-milling process under an inert atmosphere which is not likely to be an onboard process. The rehydrogenation of boron-based storage requires such extensive chemical processing that it is more likely to take place in dedicated processing plants than be an onboard process. One such fuel, NaBH4, is under investigation by the University of Delft. Simulations of this material as a hydrogen storage material on a passenger ship in the port of Amsterdam have been performed. For NaBH4 on ships two strategies exist, the fuel is either brought on-board in powder form, which complicates the handling of the fuel, but has the advantage that this makes the fuel more dense and more stable (if sealed away from air) than the alternative of bringing an aqueous solution of NaBH4 on board. Bringing a solution on board lowers the fuel density but eases the handling, however this process required strong bases to keep the NaBH4 from decomposing spontaneously in the fuel storage tank.216 One of the major challenges with the use of NaBH4 is dealing with the spent fuel, NaBO2; this waste product is a hydrated salt that is not only prone to form crystals that can clog217 the reactor system but also takes up more space than the fresh fuel, further lowering the overall volume efficiency of the boron based storage.218 A method to deal with this volume increase upon dehydrogenation is using a single tank with a moveable barrier; this would require only one tank for fuel storage. This type of volume exchange fuel tank has been described by Kim.168
The other method of bunkering with solid-state hydrogen carriers is by hydrogenation of the carrier on board the ship. Direct hydrogenation of intermetallic compounds is possible and has been used on the Ross Barlow canal boat. On this ship it took 2.5 hours to fuel it with 4 kg of hydrogen. This process is inherently slow due to the exothermicity of an adsorption reaction. An adsorption, by nature, releases heat and when the metal becomes too hot, the adsorption slows, and the dehydrogenation starts. The need for proper cooling and slow hydrogenation is thus inherent in this bunkering method. The Alanates have also been shown to be hydrogenated directly, but only with the addition of other metals as a catalyst.219
Overall, the adoption of solid-state hydrogen storage is likely to be a major engineering hurdle; the bunkering times are either limited by low adsorption speeds or by the transfer rate of the solid hydrogen state carriers to and from the ship. Unlike like liquids, powders cannot be pumped as easily; furthermore, most of the hydrogen storage powders are not stable when exposed to oxygen or moisture and thus should be transferred under an inert atmosphere. Due to the wide variety of hydrogen storage materials for solid state hydrogen there are a lot of options on how to handle, store and transport each of the fuel types on board. Likely, this disruptive character of the bunkering and storage process is one of the major hurdles to be overcome should solid state hydrogen storage become a storage method for the maritime industry.
5.4 Reactor development
For those hydrogen storage media that require a chemical conversion step the development of a reactor system that can work on-board is crucial. Today, chemical reactors are mostly designed to operate in stationary applications and thus do not have to deal with the motions of a ship at sea, which is expected to be a significant challenge in the development of new reactor systems.
Several reactor types have been discussed in the literature to be used for several dehydrogenation reactions. The two most used types of reactors, the tubular reactor and the continuously stirred reactor have been discussed for dehydrogenation of several hydrogen carriers. Modisha et al. reviewed the reactors used for dehydrogenation of aromatic LOHCs.220
The CSTR reactor is a closed reactor vessel that mixes the reaction mixture while it flows through the reactor. Of the hydrogen carriers discussed here NaBH4, DBT and FA have been envisioned in a CSTR-like reactor.128,216,221,222 An issue with this reactor type is the constant accumulation of impurities, which has been reported as an issue in the scaled up version of the FA dehydrogenation reactor.118
The tubular reactor (a reactor in cylindrical shape usually filled with catalyst particles) has been used for the dehydrogenation of both ammonia and DBT. An issue in this reactor is usually the formation of hotspots where the dehydrogenation is more difficult to control. Due to the closed packing of the reactor the removal of hydrogen is often also an issue. Dehydrogenation processes are equilibrium limited and hydrogen should thus be removed as fast as possible to keep the reaction going. Tubular reactors are also not very efficient on a size basis; since most of the dehydrogenation takes place at the start of the reactor, a substantial part of the reactor has dehydrogenated carrier flowing over it, which does not contribute to the reaction.
To deal with the hydrogen removal from DBT, Hydrogenous Technologies has patented a tubular reactor that is placed horizontally and only partially filled with catalyst particles. DBT flows over these particles from one side to the other. This leaves empty space where the hydrogen can move to and be removed more easily than from a completely filled reactor bed.223,224 This reactor however is not suited for use on board as the movement of the ship would heavily interfere with the flow character of the reactor. Another type of reactor that is under research for fast removal of hydrogen is membrane reactors. In this reactor type, the reactor walls are catalyst coated membranes which are only permeable for hydrogen. This fast removal of hydrogen has been shown to cause an equilibrium shift in dehydrogenation of methyl cyclohexane by 70% even at reduced temperatures.225 Membrane reactors have been under research for decades, mostly in steam reforming of methane to produce hydrogen, but the limiting factor in the development is the membrane material. The hydrogen selective membranes are made from palladium, which renders them very costly. Next to this high cost the stability of these membranes is often low, which further hampers the development of membrane reactors.226
To function on board a reactor will have to be developed adopted to be independent of the movement of the ship, or be capable of operating with alternating flow movements due to ship movement, while still being able to remove the released hydrogen efficiently to force the reaction towards dehydrogenation. In this field reactors that operate using vortex fields might be of use. At high enough inlet velocities, in the flow field in these reactors the centrifugal forces become high enough that gravity becomes negatable. Furthermore, the high centrifugal forces cause separation of the fluids with different densities while at the same time there will be a low-pressure zone where the hydrogen can be removed.227 Currently these reactors have not been tested for any dehydrogenation reaction discussed above but conceptually they could provide a promising pathway for onboard dehydrogenation of hydrogen carriers.
5.5 Hazards of on-board use
First, it must be addressed that, generally, hydrogen has a bad name when it comes to safety. The fear for this molecule can be attributed to the Hindenburg incident in 1937; the destruction of this airship struck the public with a fear of the use of hydrogen. It is also recommended to inform the public more on the use of hydrogen as an alternative to fossil fuels.175 Hydrogen is a fuel, so it has to be combustible; the ignition energy required, 0.017 mJ, is low, which combined with a wide limit of flammability (4–75 vol% in air), results in an easily ignitable fuel. Hydrogen is the smallest molecule and it can easily leak through joints and cracks in any piping or storage system. This high diffusivity is also one of its major advantages. Hydrogen is such a light gas that it easily disperses if it is released in open air.228 Detectors for hydrogen also exist that can detect hydrogen at levels 100-fold lower than the explosion limit.229 Furthermore, hydrogen is an odourless, invisible gas, that is not toxic but does cause asphyxiation by replacing the oxygen in the air at high concentrations.
When in a compressed state, the hydrogen release must be controlled, so as to not cause any risks of explosions. Storage in the tank itself is safe; the designed tanks are able to withstand bullet impacts.230
When storing hydrogen as a cryogenic liquid, so either as liquid hydrogen at −253 °C or as S-LNG at −162 °C, suitable materials have to be used that can withstand the cold temperatures so that they do not become brittle. If the cryogenic liquid spills, this can cause damage to the hull of the ship. Larger spills of liquid hydrogen or LNG on the ground cause a fast cooling of the ground surface, due to evaporation of the liquid in the ambient. Spills on board ships can be especially dangerous as this can cause cold fracture of the steel out of which the ship is constructed, and this can lead to hull damage.231 After spills of cryogenic liquids, vapour clouds form that are still at very low temperatures. These vapour clouds can pose serious dangers to people working on board of the ship, due to the low temperatures. Clouds of spilled cryogenic liquids contain a lot of water vapour, making them heavier than air, this means that they do not disperse like gaseous fuels do. This later effect thus increases the dangers for asphyxiation and explosions.
Due to high similarities between currently used fuels and the Fischer–Tropsch diesels, no real additional risks are expected with the CO2 recycled diesels. Although considered a greener fuel when made by recycling CO2, FTS diesels are chemically the same carbon chain molecules that are used as fuel today. They contain less sulphuric compounds than standard crude oil diesels, but oil spills of FTS diesels will still have a huge impact on the environment. This is in contrast with methanol; methanol is highly soluble in water and very fast to bio-degrade. Methanol spills, from ships, therefore, do not have such a great environmental impact as compared to spills of diesel fuels. However, compared to diesel, the flashpoint of methanol is lower, 11 °C vs. 52–96 °C, as is the boiling point 65 °C vs. 150–350 °C. This means that methanol poses a higher fire risk than diesel. Methanol is also toxic upon ingestion and inhalation; the lethal dose for ingestion is by median 56.2 g per person and for inhalation a concentration of 4000–13
000 ppm (parts per million) is considered deadly.232 Methanol evaporates roughly five times as fast as water, so additional monitoring of methanol content in confined spaces of a ship's engine rooms and near the fuel tanks will also be necessary to assure crew safety. Even at low concentrations long term exposure to methanol can lead to health complications.233,234
Formic acid is one of the top produced chemicals and thus handling and transport of this liquid is well known. Tanks and pumps to transport this hydrogen carrier are thus available at a large scale and can be adopted for a shipping fuel. However, formic acid is still a corrosive chemical that causes severe burns to the skin and eyes. Its vapour pressure at 20 °C is roughly twice that of water, so although less likely to evaporate than methanol, it should still be monitored in closed spaces on the ship to ensure that the air is not polluted with formic acid on the ship.114
Based on several factors, ammonia seems to be a viable candidate for a new fuel. It has a zero-carbon content, it has a decent energy density under moderate conditions, it is widely produced around the globe and an infrastructure is already in place to produce and transport the fuel on a megaton scale. The major downside of this fuel is its toxicity; the lethal dose after 10 min of exposure is estimated already at 2700 ppm, with severe irritation already at 220 ppm. The lethal dose of ammonia after 8 hours of exposure can be as low as 390 ppm.235 Ammonia, like hydrogen is an invisible gas under ambient conditions, but ammonia has a very strong odour, already at 50 ppm the human olfactory sense is able to detect ammonia in the air. The toxicity comes from ammonia's high affinity for water; when in contact with moist surfaces ammonia forms the ammonium ion (NH4+), which is highly alkaline and causes burns on animal tissue, especially in the respiratory system and the eyes.236 Ammonia exposed in large quantities will form clouds that are heavier and moved by air currents. The potential deadly area of the ammonia cloud can extend up to a few hundred meters, causing fatalities even when far removed from the point of exposure.235
DBT does have a very beneficial safety record. It has a high flash point and auto-ignition temperature and boiling temperature; this means that there are only very low fire or explosion risks with this carrier. DBT and the hydrogenated H18–DBT are physio-chemically similar to diesel fuels, but they are expected to be less toxic and less persistent in the environment than diesel. However, DBT is not a single component mixture, but contains several regioisomers, which makes a correct assessment of the toxicology more difficult. According to a set of rules of thumb, published by Boethling et al.,237 DBT shows no features that hinder its biodegradability: it does not have a high degree of halogenation, it contains only 3 aromatic rings, and there is no excessive branching of the molecule. This does not mean that this molecule is inherently bio-degradable or environmentally friendly, but neither are diesel fuels that are used today. Hydrogen storage in DBT does offer the potential to use a zero-carbon fuel, with safety risks similar to fossil fuels that do emit CO2.
Of the solid-state hydrogen storage methods there is a distinction in the stability of the carriers. Best practice is to store the hydrogen carriers away from air and moisture.238 For NaBH4, NaAlH4 and MgH2 the material safety Data Sheet shows that these products have to be stored away from moisture. If not, the hydrogen is released from the carrier and this could become hazardous if it occurs during bunkering or while sailing. NH3BH3 is more stable than the other powder storage carriers and exposure to moisture is less of an issue. For the intermetallic hydrides the system is envisioned as hybrid hydrogen storage vessels, closed containers filled with the alloy, and then charged as with compressed hydrogen. The exposure to air and moisture is thus more easily avoided than during transfer of the powder samples.
6 Conclusions
In this work several different aspects of hydrogen as an alternative fuel for shipping are discussed. Firstly, we highlight that new production methods for hydrogen should be used to gain an environmental benefit over the use of diesel fuel, and we highlighted specifically the use of electrolysis for hydrogen production since this offers the potential of a zero-carbon fuel in the entire lifecycle. The major challenge with hydrogen however is likely to be the storage. Therefore, several methods of hydrogen storage have been assessed on their usefulness as a storage technique for hydrogen applications in the maritime industry. These storage methods are compressed hydrogen, liquid hydrogen, ammonia, Fischer–Tropsch diesels, synthetic natural gas, methanol, formic acid, aromatic liquid organic hydrogen carriers and several solid-state hydrogen carriers: MgH2, NaAlH4, AB2-laves phase alloys, NaBH4 and NH3BH3. From this assessment it can be concluded that there is no magic bullet solution; not one storage method combines a high energy density, a low energy input, has all resources readily available, is non-toxic and both easy to handle and store. Ship owners, ports and regulatory institutions like the IMO, will have to make strategic choices on the methods of hydrogen storage for shipping. In this work we also provided an overview of the energy requirements of the different hydrogen storage methods over the lifetime of the storage method, using simple assumptions. This analysis showed that compressed hydrogen required the least amount of energy; the most energy consuming hydrogen storage method is FTS-diesel fuel which would require almost threefold the energy needed for compressed hydrogen. On the level of energy density however, FTS-fuel outperforms the other hydrogen carriers. The analysis of energy density also showed that while in theory the solid-state hydrogen storage carriers show decent hydrogen storage capacities, there is a need for an efficient and lightweight system to store the carriers. The analysis on energy efficiency also showed that for chemical hydrogen storage the overall efficiency of the process can be greatly improved if heat from an external source can be used. At the end of this work we highlight the most important challenges with the use of hydrogen and the different hydrogen storage methods for maritime use. Important to note is that except for FTS-fuels, LNG and methanol, there currently is no bunkering infrastructure available for large ships. The development of new bunkering infrastructure is likely to be one of the major challenges for hydrogen use in maritime shipping. Other challenges we see, are proper reactor development for the chemical hydrogen carriers (NaBH4, DBT, FA, ammonia). Not all the fuel types discussed are without danger, therefore we briefly highlighted crucial safety factors of all the different hydrogen storage carriers. With this review we hope to aid in making these strategic choices by providing a clear overview of commonly sighted hydrogen storage methods and the advantages and disadvantages of these storage methods for the maritime industry.
Conflicts of interest
There are no conflicts to declare.
Acknowledgements
For the completion of this work we would like to thank, Compagnie Maritime Belge for initial funding of the research into maritime hydrogen storage and the University of Antwerp for funding of the Doctoral Project that allowed for the completion of this work.
References
-
M. Crippa, G. Oreggioni, D. Quizzardi, M. Muntean, E. Schaaf and E. Lo Vullo, et al., Fossil CO2 and GHG emissions of all world countries [internet], European Comission, 2019 [cited on 2020 Mar 10], available from: https://edgar.jrc.ec.europa.eu/overview.php?v=booklet2019.
- E. A. Bouman, E. Lindstad, A. I. Rialland and A. H. Strømman, State-of-the-art technologies, measures, and potential for reducing GHG emissions from shipping – A review, Transp. Res. Part D: Transp. Environ., 2017, 52, 408–421, DOI:10.1016/j.trd.2017.03.022.
- European-Commission. Integrating maritime transport emissions in the EU's greenhouse gas reduction policies EN [Internet]. Communication From the Commission To the European Parliament, the Council, the European Economic and Social Committee and the Committee of the Regions. 2013. [cited on 2020 Mar 10]. Available from: https://ec.europa.eu/clima/sites/clima/files/transport/shipping/docs/com_2013_479_en.pdf.
- OECD/ITF. Reducing Shipping Greenhouse Gas Emissions: Lessons From Port-Based Incentives [Internet]. Paris; 2018 [cited on 2020 Mar 10]. Available from: https://www.itf-oecd.org/sites/default/files/docs/reducing-shipping-greenhouse-gas-emissions.pdf.
- MEPC. Annex 11 Resolution MEPC.304(72): Initial IMO Strategy on Reduction of GHG Emissions from Ships [Internet]. 2018 [cited on 2020 Mar 10]. Available from: https://wwwcdn.imo.org/localresources/en/OurWork/Environment/Documents/ResolutionMEPC.304(72)_E.pdf.
-
T. W. P. Smith, J. P. Jalkanen, B. A. Anderson, J. J. Corbett, J. Faber and S. Hanayama, et al. Third IMO Greenhouse Gas Study 2014 [Internet]. 2015 [cited on 2020 Mar 10]. Available from: https://glomeep.imo.org/wp-content/uploads/2016/06/GHG3-Executive-Summary-and-Report_web.pdf.
- D. R. Johnson, R. Heltzel, A. C. Nix, N. Clark and M. Darzi, Greenhouse gas emissions and fuel efficiency of in-use high horsepower diesel, dual fuel, and natural gas engines for unconventional well development, Appl. Energy, 2017, 206, 739–750 CrossRef CAS.
- P. Balcombe, J. Brierley, C. Lewis, L. Skatvedt, J. Speirs and A. Hawkes,
et al., How to decarbonise international shipping: Options for fuels, technologies and policies, Energy Convers. Manage., 2019, 182, 72–88 CrossRef CAS.
- R. Halim, L. Kirstein, O. Merk and L. Martinez, Decarbonization Pathways for International Maritime Transport: A Model-Based Policy Impact Assessment, Sustainability, 2018, 10(7), 2243, DOI:10.3390/su10072243.
- IEA. The Future of Hydrogen, Seizing toaday's opportunities [Internet]. Reported prepared by the IEA for the G20, Japan. 2019 [cited on 2020 Mar 10]. Available from: https://www.capenergies.fr/wp-content/uploads/2019/07/the_future_of_hydrogen.pdf.
- J. A. Turner, Sustainable hydrogen production, Science, 2004, 305(5686), 972–974 CrossRef CAS.
- M. Liu, C. Li, E. K. Koh, Z. Ang and J. S. Lee Lam, Is methanol a future marine fuel for shipping?, J. Phys.: Conf. Ser., 2019, 1357, 1–10 CrossRef . Available from https://iopscience.iop.org/article/10.1088/1742-6596/1357/1/012014/pdf.
- R. F. Service, The hydrogen backlash, Science, 2004, 305(5686), 958–961 CrossRef CAS.
- F. Zhang, P. Zhao, M. Niu and J. Maddy, The survey of key technologies in hydrogen energy storage, Int. J. Hydrogen Energy, 2016, 41, 14535–14552 CrossRef CAS.
- L. J. Murray, M. Dinc and J. R. Long, Hydrogen storage in metal-organic frameworks, Chem. Soc. Rev., 2009, 38(5), 1294–1314 RSC.
- F. Lamari Darkrim, P. Malbrunot and G. P. Tartaglia, Review of hydrogen storage by adsorption in carbon nanotubes, Int. J. Hydrogen Energy, 2002, 27(2), 193–202 CrossRef.
- A. Züttel, P. Sudan, P. Mauron, T. Kiyobayashi, C. Emmenegger and L. Schlapbach, Hydrogen storage in carbon nanostructures, Int. J. Hydrogen Energy, 2002, 27(2), 203–212 CrossRef.
- Q. L. Zhu and Q. Xu, Liquid organic and inorganic chemical hydrides for high-capacity hydrogen storage, Energy Environ. Sci., 2015, 8(2), 478–512 RSC.
- P. T. Aakko-Saksa, C. Cook, J. Kiviaho and T. Repo, Liquid organic hydrogen carriers for transportation and storing of renewable energy – Review and discussion, J. Power Sources, 2018, 396, 803–823, DOI:10.1016/j.jpowsour.2018.04.011.
- P. Makowski, A. Thomas, P. Kuhn and F. Goettmann, Organic materials for hydrogen storage applications: from physisorption on organic solids to chemisorption in organic molecules, Energy Environ. Sci., 2009, 2(5), 480 RSC . Available from: http://xlink.rsc.org/?DOI=b822279g.
- M. Yadav and Q. Xu, Liquid-phase chemical hydrogen storage materials, Energy Environ. Sci., 2012, 5(12), 9698–9725 RSC.
- L. Schlapbach and A. Züttel, Hydrogen-storage materials for mobile applications, Nature, 2001, 414(6861), 353–358 CrossRef CAS.
- A. N. Rollinson, J. Jones, V. Dupont and M. V. Twigg, Urea as a hydrogen carrier: A perspective on its potential for safe, sustainable and long-term energy supply, Energy Environ. Sci., 2011, 4(4), 1216–1224 RSC.
- S. Kar, J. Kothandaraman, A. Goeppert and G. K. S. Prakash, Advances in catalytic homogeneous hydrogenation of carbon dioxide to methanol, J. CO2 Util., 2018, 23, 212–218 CrossRef CAS.
- G. A. Olah, G. K. S. Prakash and A. Goeppert, Anthropogenic chemical carbon cycle for a sustainable future, J. Am. Chem. Soc., 2011, 133(33), 12881–12898 CrossRef CAS.
- S. Saeidi, N. A. S. Amin and M. R. Rahimpour, Hydrogenation of CO2 to value-added products – A review and potential future developments, J. CO2 Util., 2014, 5, 66–81, DOI:10.1016/j.jcou.2013.12.005.
- G. Centi, E. A. Quadrelli and S. Perathoner, Catalysis for CO2 conversion: A key technology for rapid introduction of renewable energy in the value chain of chemical industries, Energy Environ. Sci., 2013, 6, 1711–1731 RSC.
- T. Sinigaglia, F. Lewiski, M. E. Santos Martins and J. C. Mairesse Siluk, Production, storage, fuel stations of hydrogen and its utilization in automotive applications-a review, Int. J. Hydrogen Energy, 2017, 42(39), 24597–24611, DOI:10.1016/j.ijhydene.2017.08.063.
- A. Sartbaeva, V. L. Kuznetsov, S. A. Wells and P. P. Edwards, Hydrogen nexus in a sustainable energy future, Energy Environ. Sci., 2008, 1(1), 79–85 RSC.
- F. Safari and I. Dincer, A review and comparative evaluation of thermochemical water splitting cycles for hydrogen production, Energy Convers. Manage., 2020, 205, 112182 CrossRef CAS.
- K. M. van Geem, V. V. Galvita and G. B. Marin, Making chemicals with electricity, Science, 2019, 364(6442), 734–735 CrossRef CAS.
- P. Nikolaidis and A. Poullikkas, A comparative overview of hydrogen production processes, Renewable Sustainable Energy Rev., 2017, 67, 597–611, DOI:10.1016/j.rser.2016.09.044.
- A. Iulianelli, S. Liguori, J. Wilcox and A. Basile, Advances on methane steam reforming to produce hydrogen through membrane reactors technology: A review, Catal. Rev.: Sci. Eng., 2016, 58(1), 1–35 CrossRef CAS.
-
P. Sun, A. Elgowainy and E. S. Division, Updates of Hydrogen Production from SMR Process in GREET®. [Internet] 2019. [cited on 2020 Apr 10] Available from https://greet.es.anl.gov/publication-smr_h2_2019.
-
A. Q. Jakhrani, A. R. H. Rigit, A. K. Othman, S. R. Samo and S. A. Kamboh, Estimation of carbon footprints from diesel generator emissions, Proc. of the 2012 Int. Conf. in Green and Ubiquitous Tech., 2012, pp. 78–81, available from https://ieeexplore.ieee.org/document/6344193.
- S. T. Wismann, J. S. Engbaek, S. B. Vendelbo, F. B. Bendixen, W. L. Eriksen and K. Aasberg-petersen,
et al., Electrified methane reforming: a compact approach to greener industrial hydrogen production, Science, 2019, 759, 756–759 CrossRef.
-
P. L. Spath and M. K. Mann, Life Cycle Assessment of Hydrogen Production via Natural Gas Steam Reforming [Internet]. ECOS 2005 – Proc of the 18th Intl Conf on Efficiency, Cost, Optimization, Simulation, and Env Impct of En Sys, 2000,
available from: http://www.osti.gov/servlets/purl/764485/.
- M. Usman, W. M. A. Wan Daud and H. F. Abbas, Dry reforming of methane: Influence of process parameters – A review, Renewable Sustainable Energy Rev., 2015, 45, 710–744 CrossRef CAS.
-
U. P. M. Ashik, A. Viswan, S. Kudo and J. Hayashi, Chapter 3 Nanomaterials as Catalysts, Applications of Nanomaterials, Elsevier, 2018, pp. 45–82 Search PubMed.
- R. K. Parsapur, S. Chatterjee and K.-W. Huang, The Insignificant Role of Dry Reforming of Methane in CO2 Emission Relief, ACS Energy Lett., 2020, 5(9), 2881–2885 CrossRef CAS.
- Z. Bao and F. Yu, Catalytic Conversion of Biogas to Syngas via Dry Reforming Process, Adv. Bioenergy, 2018, 3, 43–76 CAS.
- D. Pakhare and J. Spivey, A review of dry (CO2) reforming of methane over noble metal catalysts, Chem. Soc. Rev., 2014, 43(22), 7813–7837 RSC.
- A. Buttler and H. Spliethoff, Current status of water electrolysis for energy storage, grid balancing and sector coupling via power-to-gas and power-to-liquids: A review, Renewable Sustainable Energy Rev., 2018, 82, 2440–2454 CrossRef CAS.
- B. Parkinson, P. Balcombe, J. F. Speirs, A. D. Hawkes and K. Hellgardt, Levelized cost of CO2 mitigation from hydrogen production routes, Energy Environ. Sci., 2019, 12(1), 19–40 RSC.
- S. A. Grigoriev, V. N. Fateev, D. G. Bessarabov and P. Millet, Current status, research trends, and challenges in water electrolysis science and technology, Int. J. Hydrogen Energy, 2020, 45(49), 26036–26058 CrossRef CAS.
- P. Rodríguez, A. Sánchez-Molina and C. Mais, Simple and Precise Approach for Determination of Ohmic Contribution of Diaphragms in Alkaline Water Electrolysis, Membranes, 2019, 9(129), 1–19 Search PubMed . Available from: https://www.mdpi.com/2077-0375/9/10/129.
- V. M. Rosa, M. B. F. Santos and E. P. da Silva, New materials for water electrolysis diaphragms, Int. J. Hydrogen Energy, 1995, 20(9), 697–700 CrossRef CAS.
- K. Zeng and D. Zhang, Recent progress in alkaline water electrolysis for hydrogen production and applications, Prog. Energy Combust. Sci., 2010, 36, 307–326 CrossRef CAS.
-
A. Keçebaş, M. Kayfeci and M. Bayat, Chapter 9 – Electrochemical hydrogen generation, in Solar Hydrogen Production: Processes, Systems and Technologies, ed. F. Calise, M. Dentice D'Accadia, M. Santarelli, A. Lanzini and D. Ferrero, Elsevier, 2019, pp. 299–317 Search PubMed.
- Ø. Ulleberg, Modeling of advanced alkaline electrolyzers: A system simulation approach, Int. J. Hydrogen Energy, 2003, 28(1), 21–33 CrossRef.
- R. Bhandari, C. A. Trudewind and P. Zapp, Life cycle assessment of hydrogen production via electrolysis e a review, J. Cleaner Prod., 2014, 85, 151–163, DOI:10.1016/j.jclepro.2013.07.048.
- X. Shi, X. Liao and Y. Li, Quantification of fresh water consumption and scarcity footprints of hydrogen from water electrolysis: A methodology framework, Renewable Energy, 2020, 154, 786–796 CrossRef CAS.
- U. Babic, M. Suermann, F. N. Büchi, L. Gubler and T. J. Schmidt, Review – Identifying critical gaps for polymer electrolyte water electrolysis development, J. Electrochem. Soc., 2017, 164(4), 387–399 CrossRef.
- A. Pandiyan, A. Uthayakumar, R. Subrayan, S. W. Cha and S. B. Krishna Moorthy, Review of solid oxide electrolysis cells: a clean energy strategy for hydrogen generation, Nanomater. Energy, 2019, 8(1), 2–22 CrossRef.
- S. P. Jiang, Challenges in the development of reversible solid oxide cell technologies: a mini review, Asia-Pac. J. Chem. Eng., 2016, 11, 386–391 CrossRef CAS.
- O. O. Talabi, A. E. Dorfi, G. D. O’Neil and D. V. Esposito, Membraneless electrolyzers for the simultaneous production of acid and base, Chem. Commun., 2017, 53(57), 8006–8009, 10.1039/C7CC02361H.
- S. M. H. Hashemi, P. Karnakov, P. Hadikhani, E. Chinello, S. Litvinov and C. Moser,
et al., A versatile and membrane-less electrochemical reactor for the electrolysis of water and brine, Energy Environ. Sci., 2019, 12(5), 1592–1604 RSC.
- D. V. Esposito, Membraneless Electrolyzers for Low-Cost Hydrogen Production in a Renewable Energy Future, Joule, 2017, 1(4), 651–658, DOI:10.1016/j.joule.2017.07.003.
- G. D. O’Neil, C. D. Christian, D. E. Brown and D. V. Esposito, Hydrogen Production with a Simple and Scalable Membraneless Electrolyzer, J. Electrochem. Soc., 2016, 163(11), 3012–3019 CrossRef.
- M. Ni, Computational fluid dynamics modeling of a solid oxide electrolyzer cell for hydrogen production, Int. J. Hydrogen Energy, 2009, 34(18), 7795–7806 CrossRef CAS.
- H. Barthelemy, M. Weber and F. Barbier, Hydrogen storage: Recent improvements and industrial perspectives, Int. J. Hydrogen Energy, 2017, 42(11), 7254–7262 CrossRef CAS . Available from: https://linkinghub.elsevier.com/retrieve/pii/S0360319916305559.
- R. Rafiee and M. A. Torabi, Stochastic prediction of burst pressure in composite pressure vessels, Compos. Struct., 2018, 185, 573–583 CrossRef.
- D. J. Durbin and C. Malardier-Jugroot, Review of hydrogen storage techniques for on board vehicle applications, Int. J. Hydrogen Energy, 2013, 38(34), 14595–14617 CrossRef CAS.
-
M. Tupper, P. Fabian, K. Mallick, J. Barker and A. Haight, High pressure carbon composite pressure vessel, US 2015/0192251 A1, 2015, available from: https://patents.google.com/patent/US20150192251A1/en Search PubMed.
- K. Meyer, F. Pignagnoli, D. Potts and G. Hunter, Lightweighting matters in energy storage, Reinf. Plast., 2014, 58(4), 20–23 CrossRef.
- A. Yamashita, M. Kondo, S. Goto and N. Ogami, Development of High-Pressure Hydrogen Storage System for the Toyota “mirai”, SAE Tech. Pap., 2015, 1–8 Search PubMed.
- E. Rivard, M. Trudeau and K. Zaghib, Hydrogen storage for mobility: A review, Materials, 2019, 12(12), 1–22 CrossRef.
-
M. Gardiner, Energy requirements for hydrogen gas compressions and liquefaction as related to vehicle storage needs, U.S. Department Of Energy Hydrogen and Fuel Cells Program Record, 2009, available from: http://www.hydrogen.energy.gov/storage.html.
-
J. Choi, KAERI/TR-2723/20: Liquid Hydrogen Properties [Internet]. 2004. [cited on 2020 May 4], available from: https://www.osti.gov/etdeweb/servlets/purl/20599211.
- M. Aasadnia and M. Mehrpooya, Large-scale liquid hydrogen production methods and approaches: A review, Appl. Energy, 2018, 212, 57–83, DOI:10.1016/j.apenergy.2017.12.033.
- M. Kanoglu, I. Dincer and M. A. Rosen, Geothermal energy use in hydrogen liquefaction, Int. J. Hydrogen Energy, 2007, 32(17), 4250–4257 CrossRef CAS.
- M. Aasadnia, M. Mehrpooya and H. Ansarinasab, A 3E evaluation on the interaction between environmental impacts and costs in a hydrogen liquefier combined with absorption refrigeration systems, Appl. Therm. Eng., 2019, 159, 113798 CrossRef CAS.
- T. K. Nandi and S. Sarangi, Performance and optimization of hydrogen liquefaction cycles, Int. J. Hydrogen Energy, 1993, 18(2), 131–139 CrossRef CAS.
- S. Krasae-in, J. H. Stang and P. Neksa, Development of large-scale hydrogen liquefaction processes from 1898 to 2009, Int. J. Hydrogen Energy, 2010, 35(10), 4524–4533, DOI:10.1016/j.ijhydene.2010.02.109.
- M. Bracha, G. Lorenz, A. Patzelt and M. Wanner, Large-scale hydrogen liquefaction in Germany, Int. J. Hydrogen Energy, 1994, 19(1), 53–59 CrossRef CAS.
-
K. Stolzenburg, D. Berstad, L. Decker, A. Elliott, C. Haberstroh and C. Hatto, et al., Efficient Liquefaction of Hydrogen: Results of the IDEALHY Project, 2013, pp. 7–9. Available from: https://www.idealhy.eu/uploads/documents/IDEALHY_XX_Energie-Symposium_2013_web.pdf.
- S. A. Sherif, N. Zeytinoglu and T. N. Veziroǧlu, Liquid hydrogen: Potential, problems, and a proposed research program, Int. J. Hydrogen Energy, 1997, 22(7), 683–688 CrossRef CAS.
- W. U. Notardonato, A. M. Swanger, J. E. Fesmire, K. M. Jumper, W. L. Johnson and T. M. Tomsik, Zero boil-off methods for large-scale liquid hydrogen tanks using integrated refrigeration and storage, IOP Conf. Ser.: Mater. Sci. Eng., 2017, 278, 1–10 Search PubMed.
- G. Arnold and J. Wolf, Liquid Hydrogen for Automotive Application Next Generation Fuel for FC and ICE Vehicles, Teion Kogaku, 2005, 40(6), 221–230 CrossRef CAS.
- T. Wallner, H. Lohse-Busch, S. Gurski, M. Duoba, W. Thiel and D. Martin,
et al., Fuel economy and emissions evaluation of BMW Hydrogen 7 Mono-Fuel demonstration vehicles, Int. J. Hydrogen Energy, 2008, 33(24), 7607–7618, DOI:10.1016/j.ijhydene.2008.08.067.
- H. T. Hwang and A. Varma, Hydrogen storage for fuel cell vehicles, Curr. Opin. Chem. Eng., 2014, 5, 42–48, DOI:10.1016/j.coche.2014.04.004.
-
C. Müller, S. Fürst and V. Klitzing, Hydrogen safety: new challenges based on BMW Hydrogen 7. Proc Second Int Conf Hydrog Saf, 2007, pp. 11–13. Available from: http://conference.ing.unipi.it/ichs2007/fileadmin/user_upload/CD/PAPERS/12SEPT/3.1.59.pdf.
- R. Raj, S. Ghandehariun, A. Kumar, J. Geng and M. Linwei, A techno-economic study of shipping LNG to the Asia-Pacific from Western Canada by LNG carrier, J. Nat. Gas Sci. Eng., 2016, 34, 979–992, DOI:10.1016/j.jngse.2016.07.024.
- J. Gretz, J. P. Baselt, O. Ullmann and H. Wendt, The 100 MW euro-Quebec hydro-hydrogen pilot project, Int. J. Hydrogen Energy, 1990, 15(6), 419–424 CrossRef CAS.
- S. Kamiya, M. Nishimura and E. Harada, Study on introduction of CO2 free energy to Japan with liquid hydrogen, Phys. Procedia, 2015, 67, 11–19, DOI:10.1016/j.phpro.2015.06.004.
- D. A. Russel and G. G. Williams, History of Chemical Fertilizer Development1, Soil Sci. Soc. Am. J., 1977, 41(2), 260 CrossRef CAS.
- S. L. Foster, S. I. P. Bakovic, R. D. Duda, S. Maheshwari, R. D. Milton and S. D. Minteer,
et al., Catalysts for nitrogen reduction to ammonia, Nat. Catal., 2018, 1(7), 490–500 CrossRef.
- C. Smith, A. K. Hill and L. Torrente-Murciano, Current and future role of Haber–Bosch ammonia in a carbon-free energy landscape, Energy Environ. Sci., 2020, 13, 331–344 RSC.
- S. Giddey, S. P. S. Badwal, C. Munnings and M. Dolan, Ammonia as a Renewable Energy Transportation Media, ACS Sustainable Chem. Eng., 2017, 5(11), 10231–10239 CrossRef CAS.
- S. Frigo and R. Gentili, Analysis of the behaviour of a 4-stroke Si engine fuelled with ammonia and hydrogen, Int. J. Hydrogen Energy, 2013, 38(3), 1607–1615 CrossRef CAS.
- T. E. Bell and L. Torrente-Murciano, H2 Production via Ammonia Decomposition Using Non-Noble Metal Catalysts: A Review, Top. Catal., 2016, 59(15–16), 1438–1457 CrossRef CAS.
- K. E. Lamb, D. M. Viano, M. J. Langley, S. S. Hla and M. D. Dolan, High-Purity H2 Produced from NH3 via a Ruthenium-Based Decomposition Catalyst and Vanadium-Based Membrane, Ind. Eng. Chem. Res., 2018, 57(23), 7811–7816 CrossRef CAS.
- A. K. Hill and L. Torrente-Murciano, Low temperature H2 production from ammonia using ruthenium-based catalysts: Synergetic effect of promoter and support, Appl. Catal., B, 2015, 172–173, 129–135, DOI:10.1016/j.apcatb.2015.02.011.
- H. Mahmoudi, M. Mahmoudi, O. Doustdar, H. Jahangiri, A. Tsolakis and S. Gu,
et al., A review of Fischer Tropsch synthesis process, mechanism, surface chemistry and catalyst formulation, Biofuels, 2018, 2(1), 11–31 Search PubMed.
- S. Saeidi, M. T. Amiri, N. A. S. Amin and M. R. Rahimpour, Progress in reactors for high temperature Fischer-Tropsch process: Determination place of intensifier reactor perspective, Int. J. Chem. React. Eng., 2014, 12(1), 639–664 Search PubMed.
-
C. Song, C. S. Hsu and I. Mochida, Chemistry of Diesel Fuels, Taylor & Francis, New York, 2000, p. 316 Search PubMed.
- Y. H. Choi, Y. J. Jang, H. Park, W. Y. Kim, Y. H. Lee and S. H. Choi,
et al., Carbon dioxide Fischer-Tropsch synthesis: A new path to carbon-neutral fuels, Appl. Catal., B, 2017, 202, 605–610, DOI:10.1016/j.apcatb.2016.09.072.
- S. M. Jarvis and S. Samsatli, Technologies and infrastructures underpinning future CO2 value chains: A comprehensive review and comparative analysis, Renewable Sustainable Energy Rev., 2018, 85, 46–68, DOI:10.1016/j.rser.2018.01.007.
- M. E. Dry, High quality diesel via the Fischer-Tropsch process – A review, J. Chem. Technol. Biotechnol., 2002, 77(1), 43–50 CrossRef CAS.
- C. Vogt, M. Monai, G. J. Kramer and B. M. Weckhuysen, The renaissance of the Sabatier reaction and its applications on Earth and in space, Nat. Catal., 2019, 2(3), 188–197, DOI:10.1038/s41929-019-0244-4.
- M. Thema, F. Bauer and M. Sterner, Power-to-Gas: Electrolysis and methanation status review, Renewable Sustainable Energy Rev., 2019, 112, 775–787 CrossRef CAS.
- H. Blanco and A. Faaij, A review at the role of storage in energy systems with a focus on Power to Gas and long-term storage, Renewable Sustainable Energy Rev., 2018, 81, 1049–1086 CrossRef.
- M. Bailera, P. Lisbona, L. M. Romeo and S. Espatolero, Power to Gas projects review: Lab, pilot and demo plants for storing renewable energy and CO2, Renewable Sustainable Energy Rev., 2017, 69, 292–312 CrossRef CAS.
- C. Wulf, J. Linßen and P. Zapp, Review of power-to-gas projects in Europe, Energy Proc., 2018, 155, 367–378 CrossRef.
- S. König, Q. Bchini, R. McKenna, W. Köppel, M. Bachseitz and J. Entress,
et al., Analysing the regional potential and social acceptance of power-to-gas in the context of decentralized co-generation in Baden-Württemberg, J. Energy Storage, 2018, 16, 93–107 CrossRef.
- M. Götz, J. Lefebvre, F. Mörs, A. McDaniel Koch, F. Graf and S. Bajohr,
et al., Renewable Power-to-Gas: A technological and economic review. Renew, Energy, 2016, 85, 1371–1390 Search PubMed.
- K. Müller, M. Städter, F. Rachow, D. Hoffmannbeck and D. Schmeißer, Sabatier-based CO2-methanation by catalytic conversion, Environ. Earth Sci., 2013, 70(8), 3771–3778 CrossRef.
- G. A. Mills and F. W. Steffgen, Catalytic methanation, Catal. Rev., 1974, 8(1), 159–210 CrossRef.
- J. Lefebvre, M. Götz, S. Bajohr, R. Reimert and T. Kolb, Improvement of three-phase methanation reactor performance for steady-state and transient operation, Fuel Process. Technol., 2015, 132, 83–90 CrossRef CAS.
- G. A. Olah, Beyond oil and gas: The methanol economy, Angew. Chem., Int. Ed., 2005, 44(18), 2636–2639 CrossRef CAS.
- M. Bowker, Methanol Synthesis from CO2 Hydrogenation, ChemCatChem, 2019, 11(17), 4238–4246 CrossRef CAS.
- É. S. Van-Dal and C. Bouallou, Design and simulation of a methanol production plant from CO2 hydrogenation, J. Cleaner Prod., 2013, 57, 38–45, DOI:10.1016/j.jclepro.2013.06.008.
- S. Enthaler, J. Von Langermann and T. Schmidt, Carbon dioxide and formic acid – The couple for environmental-friendly hydrogen storage?, Energy Environ. Sci., 2010, 3(9), 1207–1217 RSC.
- J. Hietala, A. Vuori, P. Johnsson, I. Pollari, W. Reutemann and H. Kieczka, Formic Acid, Ullmann's Encycl. Ind. Chem., 2017, 1(29), 1–20 Search PubMed.
- D. A. Bulushev and J. R. H. Ross, Towards Sustainable Production of Formic Acid, ChemSusChem, 2018, 11(5), 821–836, DOI:10.1002/cssc.201702075.
- K. Sordakis, C. Tang, L. K. Vogt, H. Junge, P. J. Dyson and M. Beller,
et al., Homogeneous Catalysis for Sustainable Hydrogen Storage in Formic Acid and Alcohols, Chem. Rev., 2018, 118(2), 372–433 CrossRef CAS.
- W. Cai, L. Liang, Y. Zhang, W. Xing and C. Liu, Real contribution of formic acid in direct formic acid fuel cell: Investigation of origin and guiding for micro structure design, Int. J. Hydrogen Energy, 2013, 38(1), 212–218 CrossRef CAS.
- R. van Putten, T. Wissink, T. Swinkels and E. A. Pidko, Fuelling the hydrogen economy: Scale-up of an integrated formic acid-to-power system, Int. J. Hydrogen Energy, 2019, 44(53), 28533–28541, DOI:10.1016/j.ijhydene.2019.01.153.
- Global CCS Institute, The Global Status of CCS, 2019, pp. 1–46, available from https://www.globalccsinstitute.com/wp-content/uploads/2019/12/GCC_GLOBAL_STATUS_REPORT_2019.pdf.
- X. Luo and M. Wang, Study of solvent-based carbon capture for cargo ships through process modelling and simulation, Appl. Energy, 2017, 195, 402–413 CrossRef CAS.
- O. Babacan, S. De Causmaecker, A. Gambhir, M. Fajardy, A. W. Rutherford and A. Fantuzzi,
et al., Assessing the feasibility of carbon dioxide mitigation options in terms of energy usage, Nat. Energy, 2020, 5(9), 720–728 CrossRef CAS.
- N. Mac Dowell, P. S. Fennell, N. Shah and G. C. Maitland, The role of CO2 capture and utilization in mitigating climate change, Nat. Clim. Change, 2017, 7(4), 243–249 CrossRef CAS.
- P. Preuster, A. Alekseev and P. Wasserscheid, Hydrogen Storage Technologies for Future Energy Systems, Annu. Rev. Chem. Biomol. Eng., 2017, 8(1), 445–471 CrossRef CAS.
- P. Preuster, Q. Fang, R. Peters, R. Deja, V. N. Nguyen and L. Blum,
et al., Solid oxide fuel cell operating on liquid organic hydrogen carrier-based hydrogen – making full use of heat integration potentials, Int. J. Hydrogen Energy, 2018, 43(3), 1758–1768, DOI:10.1016/j.ijhydene.2017.11.054.
-
J. Kjølholt, J. Maag, M. Warming, S. Hagen Mikkelsen, D. Elsa Nielsen and D. Food, et al., Survey of toluene Authors and contributors, 2014, Available from: http://www.mst.dk/english Search PubMed.
- A. Stanislaus and H. C. Barry, Aromatic hydrogenation catalysis: A review, Catal. Rev., 1994, 36(1), 75–123 CrossRef CAS.
- M. F. Wilson, P. R. Mainwaring, J. R. Brown and J. F. Kriz, Large pore nickel/silica-alumina catalysts for hydrogenation of synthetic distillates. Effects of composition and structure, Appl. Catal., 1988, 41, 177–198 CrossRef CAS.
- N. Brückner, K. Obesser, A. Bösmann, D. Teichmann, W. Arlt and J. Dungs,
et al., Evaluation of industrially applied heat-transfer fluids as liquid organic hydrogen carrier systems, ChemSusChem, 2014, 7(1), 229–235 CrossRef.
- F. Auer, D. Blaumeiser, T. Bauer, A. Bösmann, N. Szesni and J. Libuda,
et al., Boosting the activity of hydrogen release from liquid organic hydrogen carrier systems by sulfur-additives to Pt on alumina catalysts, Catal. Sci. Technol., 2019, 9(13), 3537–3547 RSC.
- P. T. Aakko-Saksa, M. Vehkamäki, M. Kemell, L. Keskiväli, P. Simell and M. Reinikainen,
et al., Hydrogen release from liquid organic hydrogen carriers catalysed by platinum on rutile-anatase structured titania, Chem. Commun., 2020, 56(11), 1657–1660 RSC.
- S. Nagatake, T. Higo, S. Ogo, Y. Sugiura, R. Watanabe and C. Fukuhara,
et al., Dehydrogenation of Methylcyclohexane over Pt/TiO2 Catalyst, Catal. Lett., 2016, 146(1), 54–60 CrossRef CAS.
- L. P. Lindfors, T. Salmi and S. Smeds, Kinetics of toluene hydrogenation on Ni/Al2O3 catalyst, Chem. Eng. Sci., 1993, 48(22), 3813–3828 CrossRef CAS.
- S. R. Kirumakki, B. G. Shpeizer, G. V. Sagar, K. V. R. Chary and A. Clearfield, Hydrogenation of Naphthalene over NiO/SiO2-Al2O3 catalysts: Structure-activity correlation, J. Catal., 2006, 242(2), 319–331 CrossRef CAS.
- D. Teichmann, W. Arlt and P. Wasserscheid, Liquid Organic Hydrogen Carriers as an efficient vector for the transport and storage of renewable energy, Int. J. Hydrogen Energy, 2012, 37(23), 18118–18132, DOI:10.1016/j.ijhydene.2012.08.066.
- Eastman. Marlotherm SH Heat Transfer Fluid MSDS, 2020, available from: https://www.eastman.com/Pages/ProductHome.aspx?product=71114174&pn=Marlotherm+SH+Heat+Transfer+Fluid.
- N. A. A. Rusman and M. Dahari, A review on the current progress of metal hydrides material for solid-state hydrogen storage applications, Int. J. Hydrogen Energy, 2016, 41(28), 12108–12126, DOI:10.1016/j.ijhydene.2016.05.244.
- L. Pickering, M. V. Lototskyy, M. W. Davids, C. Sita and V. Linkov, Induction melted AB2-Type metal hydrides for hydrogen storage and compression applications, Mater. Today: Proc., 2018, 4(2), 10470–10478 Search PubMed.
- J. Bellosta von Colbe, J. R. Ares, J. Barale, M. Baricco, C. Buckley and G. Capurso,
et al., Application of hydrides in hydrogen storage and compression: Achievements, outlook and perspectives, Int. J. Hydrogen Energy, 2019, 44(15), 7780–7808 CrossRef CAS.
- O. V. Netskina, E. S. Tayban, I. P. Prosvirin, O. V. Komova and V. I. Simagina, Hydrogen storage systems based on solid-state NaBH4/Co composite: Effect of catalyst precursor on hydrogen generation rate, Renewable Energy, 2020, 151, 278–285 CrossRef CAS.
- R. Kumar, A. Karkamkar, M. Bowden and T. Autrey, Solid-state hydrogen rich boron-nitrogen compounds for energy storage, Chem. Soc. Rev., 2019, 48, 5350–5380 RSC.
- S. Kumar, A. Jain, H. Miyaoka, T. Ichikawa and Y. Kojima, Study on the thermal decomposition of NaBH4 catalyzed by ZrCl4, Int. J. Hydrogen Energy, 2017, 42(35), 22432–22437 CrossRef CAS.
- A. Lale, S. Bernard and U. B. Demirci, Boron Nitride for Hydrogen Storage, ChemPlusChem, 2018, 83(10), 893–903, DOI:10.1002/cplu.201800168.
- M. A. Abbas, D. M. Grant, M. Brunelli, T. C. Hansen and G. S. Walker, Reducing the dehydrogenation temperature of lithium hydride through alloying with germanium, Phys. Chem. Chem. Phys., 2013, 15(29), 12139–12146 RSC.
- B. Sakintuna, F. Lamari-Darkrim and M. Hirscher, Metal hydride materials for solid hydrogen storage: A review, Int. J. Hydrogen Energy, 2007, 32(9), 1121–1140 CrossRef CAS.
- J. Zhang, Z. Li, Y. Wu, X. Guo, J. Ye and B. Yuan,
et al., Recent advances on the thermal destabilization of Mg-based hydrogen storage materials, RSC Adv., 2019, 9, 408–428 RSC.
- J. G. Park, H. Y. Jang, S. C. Han, P. S. Lee and J. Y. Lee, Hydrogen storage properties of TiMn2-based alloys for metal hydride heat pump, Mater. Sci. Eng., A, 2002, 329–331, 351–355 CrossRef.
- A. I. Bevan, A. Züttel, D. Book and I. R. Harris, Performance of a metal hydride store on the “ross Barlow” hydrogen powered canal boat, Faraday Discuss., 2011, 151, 353–367 RSC.
- N. Takeichi, H. Senoh, T. Yokota, H. Tsuruta, K. Hamada and H. T. Takeshita,
et al., “Hybrid hydrogen storage vessel”, a novel high-pressure hydrogen storage vessel combined with hydrogen storage material, Int. J. Hydrogen Energy, 2003, 28(10), 1121–1129 CAS.
- R. Zacharia and S. U. Rather, Review of solid state hydrogen storage methods adopting different kinds of novel materials, J. Nanomater., 2015, 2015, 1–18 CrossRef . Available from https://www.hindawi.com/journals/jnm/2015/914845/.
- P. Martelli, R. Caputo, A. Remhof, P. Mauron, A. Borgschulte and A. Züttel, Stability and decomposition of NaBH4, J. Phys. Chem. C, 2010, 114(15), 7173–7177 CrossRef CAS.
- R. Retnamma, A. Q. Novais and C. M. Rangel, Kinetics of hydrolysis of sodium borohydride for hydrogen production in fuel cell applications: A review, Int. J. Hydrogen Energy, 2011, 36, 9772–9790 CrossRef CAS.
- J. Manna, B. Roy, D. Pareek and P. Sharma, Hydrogen generation from NaBH4 hydrolysis using Co-B/AlPO4 and Co-B/bentonite catalysts, Catal., Struct. React., 2017, 3(4), 157–164 CrossRef CAS.
- L. Shi, W. Xie, Z. Jian, X. Liao and Y. Wang, Graphene modified Co–B catalysts for rapid hydrogen production from NaBH4 hydrolysis, Int. J. Hydrogen Energy, 2019, 44(33), 17954–17962 CrossRef CAS.
- X. Zhang, Q. Zhang, B. Xu, X. Liu, K. Zhang and G. Fan,
et al., Efficient Hydrogen Generation from the NaBH4 Hydrolysis by Cobalt-Based Catalysts: Positive Roles of Sulfur-Containing Salts, ACS Appl. Mater. Interfaces, 2020, 12(8), 9376–9386 CrossRef CAS.
- V. Sit, R. A. Geanangel and W. W. Wendlandt, The thermal dissociation of NH3BH3, Thermochim. Acta, 1987, 113, 379–382 CrossRef CAS.
- F. H. Stephens, V. Pons and R. Tom Baker, Ammonia–borane: The hydrogen source par excellence?, J. Chem. Soc., Dalton Trans., 2007, 2(25), 2613–2626 RSC.
- A. D. Sutton, A. K. Burrell, D. A. Dixon, E. B. Garner, J. C. Gordon and T. Nakagawa,
et al., Regeneration of ammonia borane spent fuel by direct reaction with hydrazine and liquid ammonia, Science, 2011, 331(6023), 1426–1429 CrossRef CAS.
-
G. S. McGrady, Regeneration of Spent Hydride Fuel. United States: United States Patent and Trademark Office, US 2015/0315017 A1, 2015 Search PubMed.
- O. T. Summerscales and J. C. Gordon, Regeneration of ammonia borane from spent fuel materials, Dalton Trans., 2013, 42(28), 10075–10084 RSC.
- M. Lucquiaud, X. Liang, O. Errey, H. Chalmers and J. Gibbins, Addressing technology uncertainties in power plants with post-combustion capture, Energy Procedia, 2013, 37, 2359–2368, DOI:10.1016/j.egypro.2013.06.117.
-
S. Som, D. E. Longman, Z. Luo, M. Plomer and T. Lu, Three dimensional simulations of diesel sprays using n-dodecane as a Surrogate, Fall Technical Meeting of the Eastern States Section of the Combustion Institute, 2011, pp. 241–54. Available from https://www.researchgate.net/profile/Tianfeng_Lu/publication/267204075_Three_Dimensional_Simulations_of_Diesel_Sprays_Using_n-_Dodecane_as_a_Surrogate/links/546638b30cf25b85d17f5cbf/Three-Dimensional-Simulations-of-Diesel-Sprays-Using-n-Dodecane-as-a-Surrogate.pdf Search PubMed.
- J. Andersson and S. Grönkvist, Large-scale storage of hydrogen, Int. J. Hydrogen Energy, 2019, 44(23), 11901–11919 CrossRef CAS.
-
I. Seemann, C. Haberstroh and H. Quack, Efficient Large Scale Hydrogen Liquefaction, 4th European PEFC and H2 forum, 2013, pp. 1–7, available from: https://www.idealhy.eu/uploads/documents/IDEALHY_EFCF-2013_Efficient_Large_Scale_Hydrogen_Liquefaction_web.pdf Search PubMed.
- S. T. Anderson, Economics, Helium, and the U.S. Federal Helium Reserve: Summary and Outlook, Resour. Res., 2018, 27, 455–477 CrossRef CAS.
- M. Reuß, T. Grube, M. Robinius, P. Preuster, P. Wasserscheid and D. Stolten, Seasonal storage and alternative carriers: A flexible hydrogen supply chain model, Appl. Energy, 2017, 200, 290–302 CrossRef.
- M. W. Davids, M. Lototskyy, M. Malinowski, D. van Schalkwyk, A. Parsons and S. Pasupathi,
et al., Metal hydride hydrogen storage tank for light fuel cell vehicle, Int. J. Hydrogen Energy, 2019, 44(55), 29263–29272 CrossRef CAS.
- C. Na Ranong, M. Höhne, J. Franzen, J. Hapke, G. Fieg and M. Dornheim,
et al., Concept, Design and Manufacture of a Prototype Hydrogen Storage Tank Based on Sodium Alanate, Chem. Eng. Technol., 2009, 32(8), 1154–1163, DOI:10.1002/ceat.200900095.
- T. Kim, NaBH4 (sodium borohydride) hydrogen generator with a volume-exchange fuel tank for small unmanned aerial vehicles powered by a PEM (proton exchange membrane) fuel cell, Energy, 2014, 69, 721–727 CrossRef CAS.
- The CMB Group. Hydroville [Internet]. 2020 [cited 2020 Apr 22]. Available from: http://www.hydroville.be/en/.
- The Maritime Journal. Hydrogen fuelled engines [Internet]. [cited 2020 Apr 22]. Available from: https://www.maritimejournal.com/news101/power-and-propulsion/hydrogen-fuelled-engines.
- S. Garmsiri, I. Dincer and G. F. Naterer, Comparisons of automotive, locomotive, aircraft and marine conversion to hydrogen propulsion using six-sigma methodologies, Int. J. Hydrogen Energy, 2013, 38(5), 2020–2028 CrossRef CAS.
-
J. C. Gomez Trillos, D. Wilken, U. Brand and T. Vogt, HySeas III: The World's First Sea-Going Hydrogen-Powered Ferry–A Look at its Technical Aspects, Market Perspectives and Environmental Impacts, Nutzung Regen Energiequellen und Wasserstofftechnik, 2019, vol. 2019, pp. 57–80 Search PubMed.
-
T. Tronstad, Å. H. Høgmoen, G. P. Haugom and L. Langfeldt, Study on the use of fuel cells in shipping, 2017, pp. 1–108, available from http://www.emsa.europa.eu/emsa-homepage/2-news-a-press-centre/news/2921-emsa-study-on-the-use-of-fuel-cells-in-shipping.html.
-
J. W. Pratt and L. E. Klebanoff, Optimization of Zero Emission Hydrogen Fuel Cell Ferry Design, With Comparisons to the SF-BREEZE, Sand2018-0421, 2018, pp. 1–190.
-
J. W. Pratt and L. E. Klebanoff, Feasibility of the SF-BREEZE: a Zero-Emission, Hydrogen Fuel Cell, High-Speed Passenger Ferry, 2016, pp. 1–340 Search PubMed.
- P. Dimitriou and T. Tsujimura, A review of hydrogen as a compression ignition engine fuel, Int. J. Hydrogen Energy, 2017, 42(38), 24470–24486, DOI:10.1016/j.ijhydene.2017.07.232.
- J. W. Heffel, NOx emission and performance data for a hydrogen fueled internal combustion engine at 1500 rpm using exhaust gas recirculation, Int. J. Hydrogen Energy, 2003, 28(8), 901–908 CrossRef CAS.
- L. B. Braga, J. L. Silveira, M. Evaristo Da Silva, E. B. Machin, D. T. Pedroso and C. E. Tuna, Comparative analysis between a PEM fuel cell and an internal combustion engine driving an electricity generator: Technical, economical and ecological aspects, Appl. Therm. Eng., 2014, 63(1), 354–361 CrossRef CAS.
- P. Ahmadi, S. H. Torabi, H. Afsaneh, Y. Sadegheih, H. Ganjehsarabi and M. Ashjaee, The effects of driving patterns and PEM fuel cell degradation on the lifecycle assessment of hydrogen fuel cell vehicles, Int. J. Hydrogen Energy, 2020, 45(5), 3595–3608 CrossRef CAS.
-
A. Coralli, B. J. M. Sarruf, P. Emı and V. De, Fuel Cells, Science and Engineering of Hydrogen-Based Energy Technologies, Elsevier Inc., 2019, pp. 39–122, Available from: DOI:10.1016/B978-0-12-814251-6.00002-2.
- R. Lan and S. Tao, Ammonia as a suitable fuel for fuel cells, Front. Energy Res., 2014, 2, 1–4 Search PubMed.
- J. H. Wee, Applications of proton exchange membrane fuel cell systems, Renewable Sustainable Energy Rev., 2007, 11, 1720–1738 CrossRef CAS.
- K. Sasaki, K. Susuki, A. Iyoshi, M. Uchimura, N. Imamura and H. Kusaba,
et al., H2S Poisoning of Solid Oxide Fuel Cells, J. Electrochem. Soc., 2006, 153(11), 1–8 CrossRef.
- A. Verma and K. Scott, Development of higherature PEMFC based on heteropolyacids
and polybenzimidazole, J. Solid State Electrochem., 2010, 14(2), 213–219 CrossRef CAS.
- A. Wojcik, H. Middleton, I. Damopoulos and J. Van Herle, Ammonia as a fuel in solid oxide fuel cells, J. Power Sources, 2003, 342–348 CrossRef CAS.
- F. Chuahy and S. L. Kokjohn, Solid oxide fuel cell and advanced combustion engine combined cycle: A pathway to 70% electrical efficiency, Appl. Energy, 2019, 235, 391–408 CrossRef CAS.
- R. Lan and S. Tao, Direct ammonia alkaline anion-exchange membrane fuel cells, Electrochem. Solid-State Lett., 2010, 13(8), 83–86 CrossRef.
- A. Hamnett, Mechanism and electrocatalysis in the direct methanol fuel cell, Catal. Today, 1997, 38(4), 445–457 CrossRef CAS.
- C. Rice, S. Ha, R. I. Masel, P. Waszczuk, A. Wieckowski and T. Barnard, Direct formic acid fuel cells, J. Power Sources, 2002, 111(1), 83–89 CrossRef CAS.
- P. G. Aleiferis and M. F. Rosati, Controlled autoignition of hydrogen in a direct-injection optical engine, Combust. Flame, 2012, 159(7), 2500–2515 CrossRef CAS.
- S. Verhelst, S. Verstraeten and R. Sierens, A comprehensive overview of hydrogen engine design features, Proc. Inst. Mech. Eng., Part D, 2007, 221(8), 911–920, DOI:10.1243/09544070JAUTO141.
- M. Comotti and S. Frigo, Hydrogen generation system for ammonia-hydrogen fuelled internal combustion engines, Int. J. Hydrogen Energy, 2015, 40(33), 10673–10686, DOI:10.1016/j.ijhydene.2015.06.080.
- S. M. Sarathy, P. Brequigny, A. Katoch, A. M. Elbaz, W. L. Roberts and R. W. Dibble,
et al., Laminar Burning Velocities and Kinetic Modeling of a Renewable E-Fuel: Formic Acid and Its Mixtures with H2and CO2, Energy Fuels, 2020, 34(6), 7564–7572 CrossRef CAS.
- A. Boretti, Advances in Diesel-LNG Internal Combustion Engines, Appl. Sci., 2020, 10(4), 1296 CrossRef CAS.
- C. T. Chong and S. Hochgreb, Measurements of laminar flame speeds of liquid fuels: Jet-A1, diesel, palm methyl esters and blends using particle imaging velocimetry (PIV), Proc. Combust. Inst., 2011, 33(1), 979–986 CrossRef CAS.
- P. Dirrenberger, H. Le Gall, R. Bounaceur, O. Herbinet, P. A. Glaude and A. Konnov,
et al., Measurements of laminar flame velocity for components of natural gas, Energy Fuels, 2011, 25(9), 3875–3884 CrossRef CAS.
- S. Verhelst, J. W. Turner, L. Sileghem and J. Vancoillie, Methanol as a fuel for internal combustion engines, Prog. Energy Combust. Sci., 2019, 70, 43–88 CrossRef.
- K. Liang and R. Stone, Laminar burning velocity measurement of hydrous methanol at elevated temperatures and pressures, Fuel, 2017, 204, 206–213 CrossRef CAS.
- International Chemical Safety Cards – FORMIC ACID [Internet]. [cited 2020 Sep 23]. Available from: http://www.inchem.org/documents/icsc/icsc/eics0485.htm.
- K. Reddi, A. Elgowainy, N. Rustagi and E. Gupta, Impact of hydrogen SAE J2601 fueling methods on fueling time of light-duty fuel cell electric vehicles, Int. J. Hydrogen Energy, 2017, 42(26), 16675–16685, DOI:10.1016/j.ijhydene.2017.04.233.
- T. Bourgeois, F. Ammouri, M. Weber and C. Knapik, Evaluating the temperature inside a tank during a filling with highly-pressurized gas, Int. J. Hydrogen Energy, 2015, 40(35), 11748–11755, DOI:10.1016/j.ijhydene.2015.01.096.
-
K. Hyde and A. Ellis, DUAL Ports De-carbonising port business today Feasibility of Hydrogen Bunkering[Internet]. 2020. [cited 2020 Sep 3]. Available from https://northsearegion.eu/media/9385/feasibility-of-hydrogen-bunkering-final-080419.pdf.
- Society for Gas as a Marine Fuel. Gas As a Marine Fuel [Internet].; 2019. [cited 2020 May 15]. Available from: https://www.sgmf.info/assets/docs/sgmf-guide.pdf.
-
B. Granath, Liquid Hydrogen--the Fuel of Choice for Space Exploration [Internet]. 2015 [cited 2019 Mar 20]. Available from: https://www.nasa.gov/content/liquid-hydrogen-the-fuel-of-choice-for-space-exploration.
- X. W. Sun, Z. Y. Guo and W. Huang, Passive zero-boil-off storage of liquid hydrogen for long-time space missions, Int. J. Hydrogen Energy, 2015, 40(30), 9347–9351, DOI:10.1016/j.ijhydene.2015.05.184.
- L. Wang, Y. Li, F. Zhang and Y. Ma, Performance analysis of no-vent fill process for liquid hydrogen tank in terrestrial and on-orbit environments, Cryogenics, 2015, 72, 161–171, DOI:10.1016/j.cryogenics.2015.10.001.
- Kennedy Space Center Operations Directorate. Liquid Hydrogen Consumption During Space Shuttle Program [Internet]. 2011. [cited 2020 Mar 20]. Available from: https://ntrs.nasa.gov/archive/nasa/casi.ntrs.nasa.gov/20110008561.pdf.
- J. Zheng, L. Chen, X. Xu, L. Guo, Y. Zhou and J. Wang, A novel insulation system based on active cooling without power input for liquid hydrogen storage, Energy, 2019, 182, 1–10, DOI:10.1016/j.energy.2019.06.050.
- J. K. Partridge, Fractional consumption of liquid hydrogen and liquid oxygen during the space shuttle program, AIP Conf. Proc., 2012, 1434(57), 1765–1770 CrossRef CAS.
- P. Krata, The Impact of Sloshing Liquids on Ship Stability for Various Dimensions of Partly Filled Tanks. TransNav, Int. J. Mar. Navig. Saf. Sea Transp., 2013, 7(4), 481–489 Search PubMed.
-
P. Bosma and R. K. Nagelvoort, Liquefaction Technology; Developments through History, Proceedings of the 1st Annual Gas Processing Symposium, Elsevier, 2009, pp. 19–31.
- E. Vanem, P. Antão, I. Østvik and F. D. C. de Comas, Analysing the risk of LNG carrier operations, Reliab. Eng. Syst. Saf., 2008, 93(9), 1328–1344 CrossRef.
- M. Svanberg, J. Ellis, J. Lundgren and I. Landälv, Renewable methanol as a fuel for the shipping industry, Renewable Sustainable Energy Rev., 2018, 94, 1217–1228, DOI:10.1016/j.rser.2018.06.058.
- O. Boucher, P. Friedlingstein, B. Collins and K. P. Shine, The indirect global warming potential and global temperature change potential due to methane oxidation, Environ. Res. Lett., 2009, 4(4), 044007 CrossRef.
- O. Schinas and M. Butler, Feasibility and commercial considerations of LNG-fueled ships, Ocean Eng., 2016, 122, 84–96, DOI:10.1016/j.oceaneng.2016.04.031.
-
D. Lensing, A study on the integra-tion of a novel NaBH4 fuelled hybrid system for a small inland vessel [Internet]. 2020 [cited 2020 Sep 22]. Available from: http://repository.tudelft.nl/.
- T. Kim and S. Kwon, Design and development of a fuel cell-powered small unmanned aircraft, Int. J. Hydrogen Energy, 2012, 37(1), 615–622 CrossRef CAS.
- E. Y. Marrero-Alfonso, J. R. Gray, T. A. Davis and M. A. Matthews, Minimizing water utilization in hydrolysis of sodium borohydride: The role of sodium metaborate hydrates, Int. J. Hydrogen Energy, 2007, 32(18), 4723–4730 CrossRef CAS.
- B. Bogdanović and M. Schwickardi, Ti-doped NaAlH4 as a hydrogen-storage material – Preparation by Ti-catalyzed hydrogenation of aluminum powder in conjunction with sodium hydride, Appl. Phys. A: Mater. Sci. Process., 2001, 72(2), 221–223 CrossRef.
- P. M. Modisha, C. N. M. Ouma, R. Garidzirai, P. Wasserscheid and D. Bessarabov, The Prospect of Hydrogen Storage Using Liquid Organic Hydrogen Carriers, Energy Fuels, 2019, 33(4), 2778–2796 CrossRef CAS.
- H. Jorschick, S. Dürr, P. Preuster, A. Bösmann and P. Wasserscheid, Operational Stability of a LOHC-Based Hot Pressure Swing Reactor for Hydrogen Storage, Energy Technol., 2019, 7(1), 146–152 CrossRef CAS.
- M. Caiti, D. Padovan and C. Hammond, Continuous Production of Hydrogen from Formic Acid Decomposition over Heterogeneous Nanoparticle Catalysts: From Batch to Continuous Flow, ACS Catal., 2019, 9(10), 9188–9198 CrossRef CAS.
-
H. T. GmbH, Reactor apparatus for dehydrogenating a carrier medium, US Pat., PCT/EP2016/073896, 2018 Search PubMed.
-
J. Rathke, M. Kusche, F. Westerath, B. Melcher and C. Randig, Reactor apparatus for dehydrogenating a carrier medium [Internet]. United States; 2019 [cited 2020 Mar 16]. p. 14. Available from: https://patents.google.com/patent/US10350566B2/en.
- A. Gora, D. A. P. Tanaka, F. Mizukami and T. M. Suzuki, Lower Temperature Dehydrogenation of Methylcyclohexane by Membrane-assisted Equilibrium Shift, Chem. Lett., 2006, 35(12), 1372–1373, DOI:10.1246/cl.2006.1372.
- D. Alique, D. Martinez-Diaz, R. Sanz and J. A. Calles, Review of supported pd-based membranes preparation by electroless plating for ultra-pure hydrogen production, Membranes, 2018, 8, 1–39 CrossRef.
- A. Gonzalez-Quiroga, P. A. Reyniers, S. R. Kulkarni, M. M. Torregrosa, P. Perreault and G. J. Heynderickx,
et al., Design and cold flow testing of a Gas-Solid Vortex Reactor demonstration unit for biomass fast pyrolysis, Chem. Eng. J., 2017, 329, 198–210 CrossRef CAS.
-
J. B. Saffers and V. V. Molkov, Hydrogen safety engineering framework and elementary design safety tools, International Journal of Hydrogen Energy, Elsevier Ltd, 2014, p. 6268–85 Search PubMed.
- M. Xiao, S. Liang, J. Han, D. Zhong, J. Liu and Z. Zhang,
et al., Batch Fabrication of Ultrasensitive Carbon Nanotube Hydrogen Sensors with Sub-ppm Detection Limit. ACS, Sensors, 2018, 3(4), 749–756 CAS.
-
B. Paczkowski, Ballistic Testing of Pressurized Hydrogen Storage Cylinders, Power Sources Conference, 2004, pp. 1–4.
-
S. Mokhatab, J. Valappil, J. Y. Mak and D. A. Wood, Chapter 9 – LNG Safety and Security Aspects, in Handbook of Liquefied Natural Gas, ed. S. Mokhatab, J. Valappil, J. Y. Mak and D. A. Wood, Gulf Professional Publishing, 2014, pp. 359–435 Search PubMed.
- C. S. Moon, Estimations of the lethal and exposure doses for representative methanol symptoms in humans, Ann. Occup. Environ. Med., 2017, 29(44), 1–6 Search PubMed.
- S. W. Verbruggen, TiO2 photocatalysis for the degradation of pollutants in gas phase: From morphological design to plasmonic enhancement, J. Photochem. Photobiol., C, 2015, 24, 64–82 CrossRef CAS.
- A. Apter, A. Bracker, M. Hodgson, J. Sidman and W. Y. Leung, Epidemiology of the sick building syndrome, J. Allergy Clin. Immunol., 1994, 94, 277–288 CrossRef CAS.
- National Research Council (US) Committee on Acute Exposure Guideline. Ammonia Acute Exposure Guideline Levels, in Acute Exposure Guideline Levels for Selected Airborne Chemicals [Internet]. 2008 [cited 2020 Mar 26]. 58–114. Available from: https://www.ncbi.nlm.nih.gov/books/NBK207883/.
-
J. Nowatzki, Anhydrous Ammonia: Managing The Risks—Publications [Internet]. 2016 [cited 2020 Mar 26]. Available from: https://www.ag.ndsu.edu/publications/crops/anhydrous-ammonia-managing-the-risks.
- R. S. Boethling, E. Sommer and D. DiFiore, Designing small molecules for biodegradability. Chemical Reviews, J. Am. Chem. Soc., 2007, 107, 2207–2227 CAS.
- H. Cao, P. Georgopanos, G. Capurso, C. Pistidda, F. Weigelt and A. L. Chaudhary,
et al., Air-stable metal hydride-polymer composites of Mg(NH2)2–LiH and TPXTM, Mater. Today Energy, 2018, 10, 98–107 CrossRef.
|
This journal is © The Royal Society of Chemistry 2021 |