DOI:
10.1039/D0CS01363C
(Review Article)
Chem. Soc. Rev., 2021,
50, 6221-6239
Natural and artificial humic substances to manage minerals, ions, water, and soil microorganisms
Received
27th October 2020
First published on 1st April 2021
Abstract
The chemistry of humic substances (HSs) occurs hidden from our sight, but is of key importance to agriculture and the environment, and nowadays even to medicine and technology. HSs are nowadays not only natural, but extracted and engineered, and in the past 20 years such products have been widely used in soil improvement and environment governance. In this review, we collate and summarize the applications and working principles of such HSs in agriculture and environmental ecology, mainly to elaborate the multiple roles of this functional polymer along with physical chemical quantification. Then several of the latest synthesis technologies, including hydrothermal humification technology (HTH), hydrothermal carbonization technology (HTC) and hydrogen peroxide oxidation technology (HOT) are presented, which were introduced to prepare artificial humic substances (A-HSs). The availability of reproducible and tunable synthetic A-HSs is a new chemical tool, and effects such as solubilization of insoluble phosphorus minerals, recovery of phosphorus, improvement of soil fertility for crop growth and reduction of toxicity of typical pollutants, can now be analyzed in detail and quantified. As a result, we can provide an effective chemical technology for utilizing biomass side products (“biowaste”) to generate A-HSs of different types, thus realizing improvement in agricultural production and control of environmental pollution by the macro-synthesis of A-HSs-.
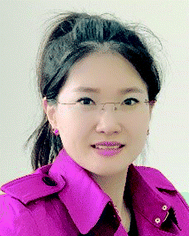
Fan Yang
| Fan Yang is a professor at the College of Water Conservancy and Civil Engineering of Northeast Agricultural University Harbin, as well as the Head of the International Joint Laboratory “Smart Soil” between NEAU and the MPI of Colloids and Interfaces. Her research interests include simulated synthesis of unique soil-inspired polymers, preparation of functional nanocomposites for soil applications and rational utilization of redox buffering in humic matter. This includes solid biomass refining, as well as interfacing to the soil microbiome and regulatory mechanisms. |
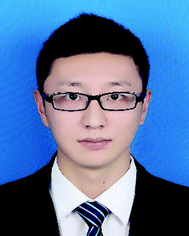
Chunyu Tang
| Chunyu Tang is a doctor candidate at the College of Water Conservancy and Civil Engineering of Northeast Agricultural University Harbin, as well as a member of the International Joint Laboratory of Northeast Agricultural University and Max Planck Institute of Colloids and Interfaces (NEAU-MPICI), under the supervision of Prof. Fan Yang. Currently, he devotes himself to engaging in the treatment of soil pollution and soil carbon fixation by artificial humic acid. |
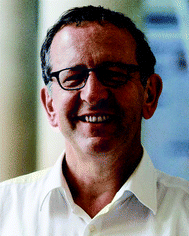
Markus Antonietti
| Markus Antonietti is the Materials Chemistry Director of the Max Planck Institute of Colloids and Interfaces in Potsdam-Golm. He is an expert in polymers and covalent materials and has focused his attention in the last years to the fields of green chemistry, and sustainable materials, but also to the new global cycles of energy, food, water, and CO2 constituting the Anthropocene. In his free time, he enjoys cooking and performing in a rock band. |
1. Introduction
Humus, or more precisely humic substance (HS), is a well-known, heterogeneous high-molecular-weight organic material, which widely exists in various environments.1,2 It is the product of humification, which is essentially the transformation of debris from living organisms into refractory organic compounds.3,4 Humification is considered to be the second largest process after photosynthesis in the carbon cycle.5 As the largest component of soil organic matter,6 HS accounts for 60–80% of it7 and plays an important role in maintaining soil ecosystem services.8,9 This is due to its specific structure, i.e. a condensed carbon structure with abundant oxygen-containing functional groups, including carboxyl, phenolic hydroxyl, alcoholic hydroxyl, carbonyl, quinone and methoxy groups, and the connected superior physicochemical properties.2,10
Since HS was firstly described in modern science literature in 1761,11 its impact on soils has been increasingly studied. HSs can be subdivided into humin (HM) (neither soluble in alkali nor in acid), humic acid (HA) (only soluble in water under alkaline conditions) and fulvic acid (FA) (soluble in water under all pH conditions) based on the solubility in water, described in more detail by Schnitzer.12 To get a rough overview of the research (including the number of articles and research orientation), which was related to HS in the last twenty years (1999–2019), “humic substance”, “humin”, “humic acid” and “fulvic acid” were used as the retrieving subjects, and the specific results are displayed in Fig. 1. The largest accounts of studies on HM, HA, FA, and the various components of HS, come from Environmental Sciences, reflecting the use of HS for soil improvement and water and soil pollution remediation. This is all due to some specific physical and chemical functions of HS, such as binding metals/ions/molecules/other biopolymers, retaining water, improving soil structure, taking part in redox reactions, and many more.3,9,13
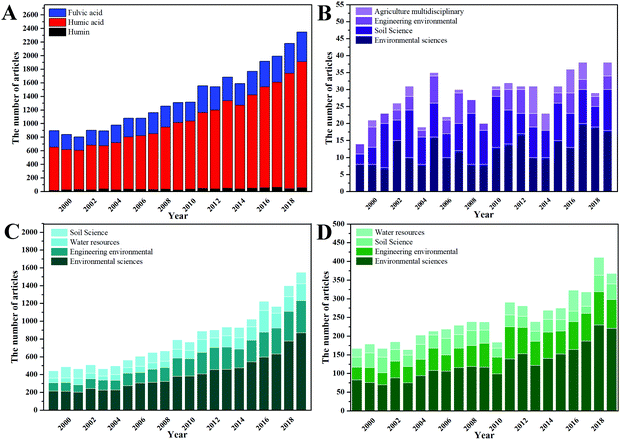 |
| Fig. 1 The number of articles related to “Humic Substance” in Web of Science from 1999 to 2019 (A: the numbers of articles on “Humin”, “Humic acid” and “Fulvic acid”, respectively; B: the top four research fields dealing with humin and the number of articles; C: the same for humic acid; D: the same for fulvic acid). | |
The HS used for soil improvement and environmental remediation is typically sourced from commercial humic substances (CHSs, also sometimes named humates), which are mostly obtained through extraction from natural carbon-rich deposits, such as leonardite, peat, sapropel and coal, or compost.3,14,15 As the products of geochemical processes, peat and coals are non-renewable resources.16 Larger-scale practical applications thereby automatically face a sustainability issue. This review focuses on a second option: to generate HSs by human-made processes along the concepts of circular economy from stranded biomass. Most prominent and traditional, composting is a biochemical process that promotes the conversion of biodegradable organic matter from solid waste to stable HS through the presence of microorganisms in the compost.17–20 Although composting is a known method to improve and restore soils, it has a risk of introducing heavy metals, organic plastics and pathogens into soil.21 On top, it is chemical-engineering-wise rather ineffective, as most of the organic matter is indeed metabolized throughout composting and liberated as methane and CO2 to the atmosphere. In order to solve the problem of efficiency and pollution, the use of agricultural side products as precursors for the synthesis of A-HSs in more carbon-effective processes seem to be the direction to go, especially in times of redesigning the carbon cycle towards a carbon neutral economy.
To understand the functions of HS in soil improvement, fertility, and remediation, we will first review central previous studies, mainly concerning the applications and mechanisms of extracted humic substance (E-HS) in agriculture and environment. Then we will summarize the current research in macro-syntheses of A-HS, and end with a description of the resulting further prospects of A-HS for the carbon cycle and other industrial fields.
2. The chemistry of humic matter synthesis
Humic substance is obviously one of the chemical compounds on which life depends upon, and there are indeed the above-mentioned articles and complete books on various aspects of its structure. As this is not the central focus of this review, we will repeat some essentials only in this review for better understanding of its chemical structure.
Natural HS is usually the product of biomass degradation through biological, physical and chemical transformation processes, while the conjugated humin complexes with soil minerals can be stable up to thousands of years. The term “humic acid” comes from the early, even pre-chemistry observation that strong bases dissolve significant parts of the humic matter of black soil, while this extract can be isolated by reprecipitation with acid. Other parts do not reprecipitate, and the third part is called “fulvic acid”. Larger parts of humic matter are also acidic, but only swell with base (and change physico-chemical properties as described below), but do not dissolve. As analytical techniques such as pyrolysis-MS or solid-state NMR reveal a very similar chemical character, we feel motivated to the simplification that this is only the more hydrophobic or cross-linked part of the same, a chemically broad material. HA and HS are generated from lignocellulosic biomass, and the incomplete biological degradation and follow-up chemical conversion to the final humins indeed leaves elements of carbohydrates, mostly lignin, amino acids and fatty acids copolymerized into one final polycondensate. This is discussed in detail in a previous article.23 In spite of the very many different biomasses and the many different pH and biological conditions to start from, all humic substances are – within typical variations for copolymers – nevertheless remarkably similar, and the use of one name for all these structures is justified. The molecular structure can be schematically simplified as shown in Fig. 2. Titration determines typically one acidic carboxylate group per 1000 mass units, and these linked organic acids come from the alkaline or anaerobic digestion of carbohydrates. They condense with the phenolic groups of the lignin to a mixed polyamphiphile, where also the phenols contribute to ion binding and mineral adhesion. Phenols can be quantified in electrochemical experiments by their redox capacity, and there is about 1 electron storage capacity in about 1000 mass units.
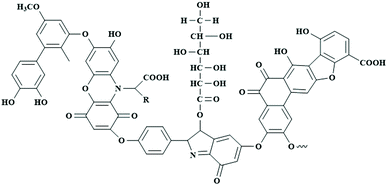 |
| Fig. 2 Schematic structure of a typical humic acid molecule, in agreement with pyrolysis-MS, solid state NMR, and other physical characterization techniques (R represents aliphatic chains such as –CH3, –CH2, and CH).22 (Figure has been adapted from ref. 22 with permission from Elservier, copyright 2020.) | |
Besides minor condensation with amino acids in Maillard-like reactions giving a still comparably low nitrogen content, up to 7 wt% of hydrocarbon tails can be detected, coming from waxes and (less degradable, cross-linked) fatty acids of the biomass. The three major entities COOH, phenolic OH, and hydrophobic tails vary to a certain extent due to different biological origins, but are contained in all samples we ever analyzed. We will illustrate a typical composition determination along with one of our own previous workpieces,23 where we have prepared artificial humic acid (A-HA) through a hydrothermal humification reaction and also analysed, as a reference, the chemical nature and structure with extracted natural humic matter from black soils (N-HA, collected from Harbin, China). Results from elemental composition analysis revealed that the contents of C, N, H, O, and S are 58.91%, 3.93%, 6.35%, 25.59% and 2.45% in the N-HA sample, which was similar to those of A-HA. FT-IR analysis demonstrated the existence of –OH, –COOH, –CH3, –CH2, and C–H groups and aromatic structures in A-HA and N-HA (spectra not shown). Solid state nuclear magnetic resonance (NMR) spectra (Fig. 3A) essentially show four chemical regions, that is, aliphatic carbons (0–50 ppm), oxygenated aliphatic carbons (50–100 ppm), aromatic carbons (100–160 ppm), and carboxyl/carbonyl carbons (160–220 ppm) in both A-HA and N-HA. SS-NMR in this simple mode is not quantitative, but is for instance remarkably useful to determine the alkyl-content of a HA,24 which again often relates to wax content and the leftovers of lipid membranes of microorganisms. In our case, the A-HA was designed to be “fat-free”. Pyrolysis-gas-chromatography/mass spectrometry (Py-GC/MS) analysis (Fig. 3B) is useful to analyse the fragmentable, volatile part of the organic structure, which in the case of HAs covers about 80% of the mass, the remainder is turned into non-volatile carbon (and mostly relatable to aromatic entities). The “fingerprint plots” show astonishing similarities between A-HA and N-HA. Generally, we can attribute the typical peaks to four categories, namely, protein- (Pr), polysaccharide- (Ps), lignin- (Lg) and lipid-based (Lp) peaks. Some representative fragments such as pyrrole, pyridine, and other N-containing compounds are also detected in the A-HA and N-HA samples and relate to amin and ammonia in the starting products. The natural extraction product has a relatively larger abundance of N-containing compounds released in the pyrograms (5.96% in A-HA versus 7.34% in N-HA). Guaiacol and its derivatives are the main fragments of lignin, and the signals of these compounds in pyrolysis products of all humic substances underline the contribution of lignin structures to the humic acid structure. In addition, a significant proportion of long-chain fatty acids was detected in the products of both A-HA and N-HA, and the main aliphatic compounds identified are octene, nonene, and undecene, i.e. typical decarboxylation products of fatty acids. Fulvic acids are more polar, largely constituted only of low molecular weight organic (poly)acids, and rather free of lignin and hydrocarbon fragments. Insoluble humins show just gradually different composition and for instance very similar pyrolysis-MS data, which means that they are related structures and created by the same (bio)chemical cascade processes. We will discuss below that artificial humic acids made in the absence of biology and only by chemical means are also remarkably similar in structure to natural extracts. We take this as a point that humification is a spontaneous exothermal process controlled by energy minimization, which can be approached both by biocatalysis or classical chemical reaction engineering.
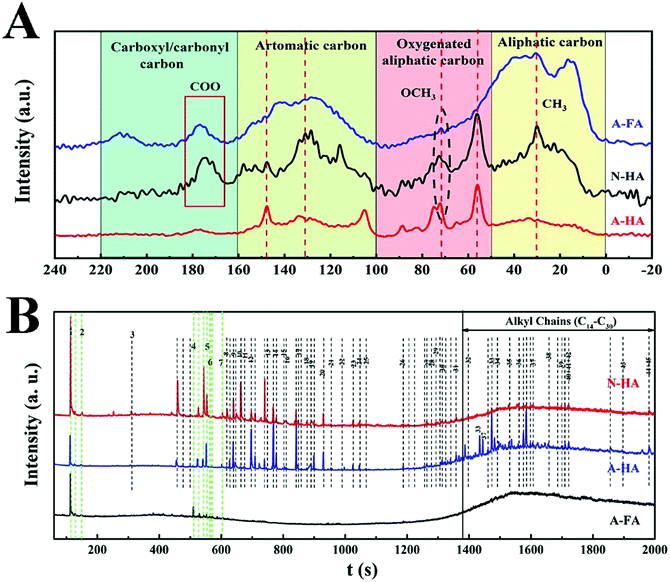 |
| Fig. 3 Illustration of some options for compositional and structural analysis of HAs. (A) Solid state 13C-NMR spectra from artificial and natural humic substances. (B) Pyrolysis-MS-data and assignment to diverse fragments by biomass origin for natural and artificial humic acids and artificial fulvic acid (main peaks labelled with numbers 8, 9, 10, 11, 17, 18, 35, 36, 38, 41, and 42 represent protein-based fragments; main peaks labelled with numbers 1, 2, 3, 6, 7, 13, 15, 16, 25, 26, 28, and 30 represent polysaccharide-based fragments; main peaks labelled with numbers 29, 34, 39, 43, and 45 represent lignin-based fragments; main peaks labelled with numbers 14, 22, 23, 31, 37, and 44 represent lipid-based fragments).23 (Figures have been adapted from ref. 23 with permission from Elservier, copyright 2019.) | |
3. Applications
Soil is mostly composed of mineral grains of diverse composition and size, and soil colloids are the finest particles of these mixtures of solids and the most active substances in soils.25 As they come with the highest specific surface area, soil colloids determine the adsorptive properties and all other interface-related physical and chemical properties of the soil.26 HA is on the same colloidal scale and the most important component of organic soil colloids (also named “humus colloids”).27 It strongly interacts with inorganic surfaces, mostly the soil colloids, and this chemical interaction of HA is the key step for soil improvement and HA-related environmental remediation as it directly modifies and improves the interface related properties in a variety of functions.
3.1 Agriculture
3.1.1 Alteration of soil texture.
With the continuous tillage and overuse of farmland with the wrong crops and crop sequences, the soil structure and composition have been seriously damaged, a phenomenon that is hastening the oxidative loss of soil organic matter, loss of macro-porosity and the creation of puddled conditions is observed.7,28,29 After long continued research, many soil scientists, such as Tisdall and Oades30 and Swift,31 concluded that HS plays a central role in the formation and stability of soil aggregates, the basis of soil texture. The presence of HS promotes the formation of larger, “soft” aggregates and makes the soil become resistive to hardening. In brief, the principle of improving the structure of soils is the colloidal interaction of soft and hard matter, binding the mineral grains to loose and open aggregates, removing cementation, and further promoting the formation of porous and water-stable hetero-aggregates with a stabilized pore structure (Fig. 4A). In simple words, fertile soil is compressible and elastic. Motivated by these models, E-HSs are commonly applied as soil conditioners to improve soil structure, and a large number of experiments prove that E-HSs promote the formation and stability of soil aggregates. Piccolo32 long ago claimed that the application of HSs not only significantly improved soil aggregate stability, but also reduced the disaggregating effects of wetting and drying cycles substantially. In addition, Mamedov et al.33 also demonstrated that commercial HAs and activated sludge markedly improved macro-porosity. Not only that, the stability of the macro-aggregates (>250 nm) was also strengthened.
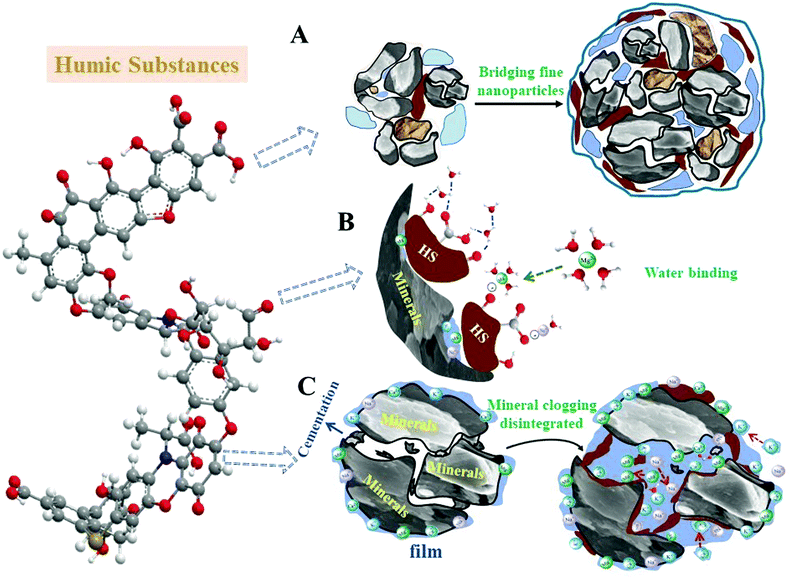 |
| Fig. 4 Schematic diagram of the effect of HSs on the physical and chemical properties of soil (A: soil structure; B: water retention capacity; C: cation exchange capacity). | |
3.1.2 Enhancement of water retention capacity.
The uptake and release of water is of obvious importance for soils.7 Large-scale over-usage caused serious water loss and soil erosion,34 especially for black soils.35 HS is recognized for its water-retaining properties because of its partial hydrophilicity (hydroxy, and carboxyl groups) and porous character36,37 (a schematic diagram is given as Fig. 4B). Brady et al.7 described that “negatively charged clay surfaces attract water through the hydrogen end of the molecule” in his book The Nature and Properties of Soils, i.e., he pointed to a strong role of hydrogen acceptor interactions with minerals. Piccolo et al.38 applied HS derived from coal to improve water retention, and the results showed that the higher the HS content, the better the soil water retention. Compared with the control group, the field capacity was increased by 25.9% by adding only 0.1 wt% HA. This indicated that the water uptake is by far higher than that explained by water-binding to HSs alone, and includes textural effects. In order to improve structural stability of lignite-based HAs and to further modify the water holding capacity, formaldehyde cross-linking of the phenol-based humic acid moieties was applied by Cihlář et al.37 Compared with unmodified HA, cross-linked HA displayed a faster water absorption rate and approximately 3 times the water holding capacity.
3.1.3 Improvement of cation exchange capacity (CEC).
The CEC of soil refers to the total amount of various exchangeable cations adsorbed by soil colloids7 (Ca2+, Mg2+, K+, Na+, and H+ make up the majority of exchangeable cations in most soils), and it is one of the important indexes to evaluate soil fertility.39,40 In general, the content of CEC in different soils is determined by the amounts of different colloidal structures, e.g. both by specific surface area and chemical nature of the minerals. As a part of the soil colloid system, HS plays a prominent role, if not a conclusive role, on the change of CEC.3 The higher the molecular weight and the more complex the HS, the higher the affinity to metal ions. Summing up the current literature, the main mechanisms of HS affecting the CEC are as follows (Fig. 4C): (1) HS keeps a larger specific surface area of the inorganic colloids open for adsorption of exchangeable cations, which are otherwise blocked by cementation and mineral–mineral contacts;25 (2) the carboxyl group and phenolic hydroxyl groups in HS dissociate to produce a large number of anions (and protons), which directly contribute to the CEC;41,42 (3) HS can accelerate the disintegration of soil minerals, which increases the specific surface area.27 Yang et al.43 accidentally found a favourable influence of FA on CEC in soils when they studied the effect of FA on the availability of phosphorus, and the higher the FA content, the higher the CEC. Mindari et al.41 conducted a study on salinized soil modified by coal-derived HS, compost and manure for rice growth and yield, and the results indicated that the content of CEC under the action of coal-derived HS (104.09 cmol kg−1) was significantly higher than that under the action of compost (80.72 cmol kg−1) and manure (59.48 cmol kg−1).
3.1.4 Carbon sequestration in soil.
Global warming is related to the ever-increasing concentrations of CO2 – among other gases – in the atmosphere.44,45 To tackle global warming, the Paris Agreement (2016), following the Kyoto protocol (1997), proposed some measures to run our planet in a more sustainable manner. Many stakeholders have proposed that slowing the use and emissions of fossil fuels would meet the proposed target of 2 °C and 1.5 °C.46–48 Indeed, emissions of fossil fuels are substantially responsible for the increase of CO2 into the atmosphere,49 but this view is incomplete. In fact, the destruction of soil organic matter (SOM) also releases CO2 into the atmosphere,50,51 and the C emissions from soil, which arise from microbial transformations of SOM, are considered to be about 60 Pg (1 Pg = 1015 g), which is about 10 times higher than the emissions from fossil fuels.44 As fossil fuel emissions are reduced in the future, we may find out that the change in the atmosphere is only minor, and that the question of how to invert C emissions from soils is much more pressing. Positively phrased, the question of how to sequester CO2 from the atmosphere and make soil a better sink again for C seems to be a most attractive research issue. A change of HS content on the scale of larger soil areas has the potential to cure the climate crisis completely. At the Paris Agreement, the so-called “4 per 1000” initiative claimed that an increase by 0.4 wt% of soil carbon in farmed areas every year is enough for a complete cure of the CO2 crisis,52 and considering on top the possible larger contribution of non-fertile, carbon-poor badlands, this looks not difficult at all. To date, soil scientists and ecologists have described ways to enhance soil C sequestration well.53–57 HS is well known for its central contribution to soil C sequestration,2 but interestingly it operates in a non-linear fashion, i.e. the addition of HS sequesters more carbon than only by the chemically added carbon. This has been pointed out by Swift,58 and the potential of soil C sequestration was clearly correlated with the amount of soil HS, or in simpler words: humification auto-catalyses further humification. In that, the chemical characteristics of HS and its ability to resist microbial decomposition, while supporting at the same time microbial growth is a key point to look at.58,59 Autotrophic bacteria in black soils, like nitrifying bacteria or sulfur bacteria, are also able to fix C from CO2, which is a powerful pathway to sequester additional C in soils.7 In that direction, some publications have proved that HS can promote the growth and activity of soil autotrophic bacteria.60,61 As a baseline of this non-linear biological mechanism of increasing soil C content, the replenishment of HS into soils directly increases the soil C content because of its carbon content, although this looks sometimes so little compared to the overall soil environment. We indeed talk about permille to percent additions, which however – appropriately applied – possess an enormous biological lever. To sum up, HS does influence soil C sequestration in potentially manifold ways, and HS can be effectively applied for C sequestration in fertile soils.
3.1.5 Increase of nutrient availability.
As mentioned above, HS has a strong ability to absorb exchangeable cations (K+, Ca2+, Mg2+, etc.), wherein they can be taken up by plants, but cannot be easily leached out by percolating waters.7 In general, the development and growth of plants require 13 essential nutrients, including C, hydrogen (H), oxygen (O), nitrogen (N), phosphorus (P), sulfur (S), iron (Fe), manganese (Mn), copper (Cu), zinc (Zn), boron (B), molybdenum (Mo) and chlorine (Cl), in addition to K+, Ca2+, and Mg2+.62 C, H and O come mainly from the atmosphere and water. The remaining elements are divided into three categories: macro-nutrients, medium-nutrients, and micro-nutrients.62
P is one of the most important macro-nutrients in plant growth63 and exists in phosphate minerals, which are found nearly everywhere in the soil, unlike N.64 However, most of these phosphates are not plant-accessible because of their low solubility.65 Not only that, most of the added phosphate fertilizer is rapidly recalcined in the soil (i.e. turned into insoluble minerals), and only a small percentage is then directly available to the plants.66 Food demands of a rapidly growing population however demand an increase in food production by using phosphate fertilizer on a large scale to make crops take up as much phosphorus as possible.64,67 A survey of P levels at the surface of the soil in 12 countries with intensive agriculture during 1961 to 2007, indicated that P increased from 6 to 21 kg P ha−1, a roughly threefold increase.68 For the mining of phosphate rock, it is estimated that approximately 21 ± 4 Mt per year of P (calculated as only P) in phosphate rock is mined and cleaned.69 From 2006 to 2016, the per capita consumption of phosphate fertilizer increased by 4.44% annually on average, and in 2016, the per capita output rose to approximately 36 kg per cap per year, a record high.70
The dilemma of such a proceeding is obvious: in the long run, it leaves more and more insoluble P in the soil, while at the same time we deplete and waste our phosphate resources,71 and using phosphate fertilizer only increases crop yields in the short term. Accordingly, the world faces an unprecedented crisis of P shortage and food security, if more clever chemistry solutions are not applied.72 As every crisis contains an opportunity and a challenge (as the Chinese proverb says “if you take the opportunity out of a crisis, only danger is left”), we can also understand that most of the land used to grow crops for a number of years is an untapped treasure trove of P, which is just locked up by insolubility, especially by the black soils. The addition of HS to soil makes it possible to solubilize the otherwise insoluble P in soil and to provide sufficient available phosphorus for plant growth.73,74 The mechanism by which HS can increase the available phosphorus content (AP) in soil for plant growth can be summarized as follows (Fig. 5A):
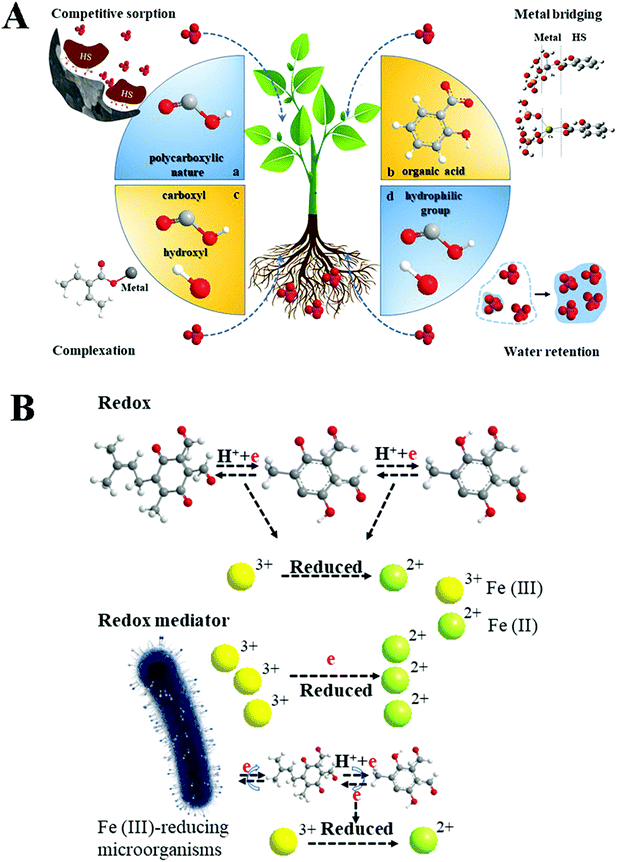 |
| Fig. 5 Mechanism of HS promoting nutrient availability (taking P and Fe as examples, in which, A-a: competitive sorption; A-b: metal bridging,81 figures have been adapted from ref. 81 with permission from Elservier, copyright 2018); A-c: complexation; A-d: water retention; (B: the pathways for increasing Fe(II) content include the redox properties of HS and its participation as a medium in redox reactions under anaerobic conditions). | |
(1) The polycarboxylic nature of HS allows strong adsorption along with some aluminium and iron oxide surfaces in soils, which in turn adsorb less phosphate and increase the AP concentration;75,76
(2) Parts of HS, especially organic acids and phenols, can react with the phosphate mineral surface and change the metal complexation, surface charge, or metal bridging, i.e. the mineral is etched. As a side product of etching, the concentration of AP is increased.77
(3) HS also changes water retention and thereby dissolution capacity in soil. This further enhances the vertical migration of AP.77
Some researchers have used HS to stimulate the redissolution of insoluble phosphates. The effect of commercial HS on the phosphate solubility in acidic and ferrosols was studied by Hua,78 and the final results revealed that the addition of HS enhances the solubility of P in soil under both low and high P conditions. The group of Du79 found that the combined application of humic acid and monocalcium phosphate increases the distance of P vertical migration and the concentration of extractable P in calcareous soil. The ability of HS to enhance the solubility of phosphate in iron and aluminum oxides is controversial. For example, Borggaard et al.80 studied the influence of HS isolated from a peat soil on phosphate adsorption by aluminium (aluminium oxide) and iron oxides (ferrihydrite and goethite) and found that HS only had a limited influence on phosphate adsorption by aluminium and iron oxides.
Fe(II), as one of the representatives of micro-nutrients, has important effects on plant growth rate, root-cap ratio, root activity and material synthesis and accumulation.82 However, Fe is generally present in the soil as Fe(III). HS plays an important role in turning Fe(III) into Fe(II) in soils for plant growth. There are two main mechanisms (Fig. 5B). One of the mechanisms is the direct reduction of Fe(III) by the photocatalytic properties of HS. HS is a macromolecular compound containing chromophores, which promote the photochemical generation of active charge carrier species with both sunlight and ultraviolet light. The chemical energy produced by light absorption is capable to be transferred to photoredox pairs, here the photoelectron to Fe(III) species or other oxidated metal ions.83 Irradiation studies show that HS uses the absorbed photons to generate a larger number of characteristic radical species, e.g. catechol-based species, and synchronously reduces Fe(III) to Fe(II).1 Meanwhile, catechuic sites are strong iron binding sites that are capable of binding ferrous species via chelation with the 1,2-benzenediol moiety,1,84 so as to support plant growth.85 In short, a complex process covering several reactions is needed to describe the photochemical systems of HS. Evidenced by the investigation of catechol abiotic transformation by Colarieti,84 the mechanisms of Fe(III) reduction and Fe(II) complexation by catechol are synchronous. Yet, not all Fe(III) is reduced to Fe(II) in the HS photochemical reaction. Roughly, the reduction rate constant of Fe(III) varies from 2.7 × 10−4 s−1 to 5.4 × 10−4 s−1 (pH = 7.0) and correlates with the content of free radicals (r = 0.54, P < 0.0586). Of course, the reaction can only occur in topsoil, limited by the penetration of light into soil.
Another important mechanism is the participation of HS as redox mediators in the process of reducing Fe(III) by Fe-reducing microorganisms.87 Fe(III)-reducing microorganisms themselves can reduce iron directly, but they need contact with Fe(III) oxides to deliver the electrons. The presence of HS as redox mediators can speed up the process. Firstly, Fe(III)-reducing microorganisms can accept electron equivalents from the oxidation of organic compounds and/or H2 and donate them to HS. Secondly, the microbially reduced HS can then abiotically transfer electrons to Fe(III) oxides.
3.1.6 Promotion of plant growth.
Already the improvement of soil structure and properties, and better water uptake, as well as regulation of recycling and availability of nutrient elements by humic substances has massively promoted plant growth. Worth noting, is that the hormone-like activity of HS on plants is another key factor.88–90 So far, numerous investigations have demonstrated that plant growth hormones, e.g. indole acetic acid (IAA) and gibberellins,90,91 can be extracted from humic substances. These molecules induce the increment of plasma membrane H+-adenosine triphosphatase (ATPase) and stimulate the elongation of plant roots, together with lateral root growth points. As such, root activity and the contact area between plant roots and soil nutrients are increased, i.e. absorption and utilization of plant nutrients are increased.92 The group of Canellas93 mainly evaluated the effects of HA and FA extracted from the topsoil of seven different oxisols on the growth of Arabidopsis thaliana. It was observed that both HA and FA are capable of improving the root development and increasing the number of lateral roots. The auxin-like activity of HS on the growth of tomatoes was further demonstrated by testing the effect of HS on the growth of mic-tom tomatoes, which are less sensitive to auxin. Trevisan et al.94 also reported experiments on the role of HS, which were extracted from faeces, on the growth of Arabidopsis thaliana, while the methods focused more on genetics. The results showed that HS exhibited auxin activity, and promoted the growth of Arabidopsis thaliana mainly through activating the auxin synthetic reporter DR5:GUS and strengthening transcription of the early auxin-responsive gene IAA19. Indole acetic acid (IAA) molecules were also identified by Pizzegehello et al.95 in the structure of HS, which was extracted from soils, compost, and vermicompost, according to the methods of immunoassays.
Excess HS amendment will also inhibit the activity of ATPase. HS activates the synthesis of auxin in plants other than possessing auxin activity by itself.96–98 For example, Mora et al.97 observed that the application of purified HS not only enhances a primary influence on root H+-ATPase activity and nitrate root-shoot distribution, but also further facilitates the concentration of several active cytokinins and polyamines related with nitrate. However, it is not the case that the more HS is added, the more favourable it is for plant growth. It was proposed that 50–300 mg L−1 HS is effective in stimulating root initiation and elongation, and that 5–25 mg L−1 HA is effective in enhancing root cell elongation.99 The antioxidant activity of HS also provides the function of scavenging free radicals resulting100 from stress (including drought, heat, ultraviolet light, herbicide use, and similar) to provide the plant with protection from oxidative damage (the damage of lipids, proteins and DNA within plant cells) by reactive oxygen species (ROS).101,102 The role of ROS on plant growth is well known and includes cell wall formation regulation,103 activation of the Ca2+ channel,104 induction of seed germination,105 balancing the transition from cell proliferation to cell differentiation,106 as well as others.107 The regulation of ROS at the cellular level in plants by HS was subjected to deeper analysis in recent years.98 Overall, HS is capable of increasing and regulating ROS production and accumulation in roots to enhance the oxidative metabolism of plant cells.108
3.1.7 Humins as an active functional matrix material to enhance microbial growth and activity.
Besides, HS can also provide both stimulation or toxic effects on microorganisms, fungi, and soil fauna.109,110 In the present review, we will focus primarily on the benefits of HS. In general, redox buffering of HS promotes the growth and activity of microorganisms. The mechanism of redox action of HS on microorganisms is complex, as it participates in redox reactions as both an electron acceptor and electron donor for microbial respiration under anaerobic conditions,87,112–114 cycling the conversion between hydroquinone–semiquinone–benzoquinone states.115Geobacter metallireducens, a Fe(III) – and humic – reducing microorganism, can stimulate the electron transfer to reduce quinone groups to hydroquinone groups to supply its own growth. In the progress, quinone groups of HS act as an electron acceptor, and acetate is metabolized and acts as an electron donor.115 HS can also act as electron donors in the respiration of G. metallireducens. In the progress, G. metallireducens uses the energy generated by the electron transfer during the oxidation of hydroquinone to quinone to supply its own growth, and hydroquinone of HS acts as an electron (or hydrogen) donor and nitrate operates as an electron acceptor.116 On the other hand, the detoxification effect of HS on organic contaminants and heavy metals also has a positive and indirect effect on the growth of microorganisms.117 The detailed mechanism and application of the effect of HS on organic contaminants and heavy metals will be presented in Section 3.2.
Even without redox dependence, HS can also promote the uptake of nutrients by microorganisms, as well as affect primary and secondary metabolism,118e.g. by primary and secondary membrane irritation depending on physical and chemical membrane interactions (“insertion”). The effect of HA extracted from leonardite on the activity and growth of Nitrosomonas europaea and Nitrobacter agilis was investigated by Vallini et al.61in vitro under axenic conditions. The experimental results showed that although nitrifying bacteria had a distinct growth effect, nitrifiers did not use HA as an alternative carbon and energy source. This was attributed to a stimulating effect of HS on the microbial cell membrane, making it more permeable and promoting the absorption of nutrients.119
3.2 Environmental remediation
Soil has the ability of self-remediation through tight binding and inclusion of inorganic chemicals into minerals or providing sources of essential nutrients for microorganisms to break down organic residues.7 However, millions of tons of potentially toxic industrial chemicals, plastic pollution, household waste, and agricultural wastes enter the soil system every year,120–122 by far exceeding the capacity of soil to hold them. The presence of HS in the soil effectively alleviates the pollution because it can interact with all classes of eco-toxins (ETs) in the polluted environment, mainly absorbing heavy metals, organic pollutants, but even radionuclides,123,124 as presented in Fig. 6. This immobilization or detoxification is due to the nature of HS, such as its redox ability, complexation, and ion exchange.3,125 We will describe in detail the mechanism and the effects of HS on eco-toxins and some of the reactions involved in actual applications.
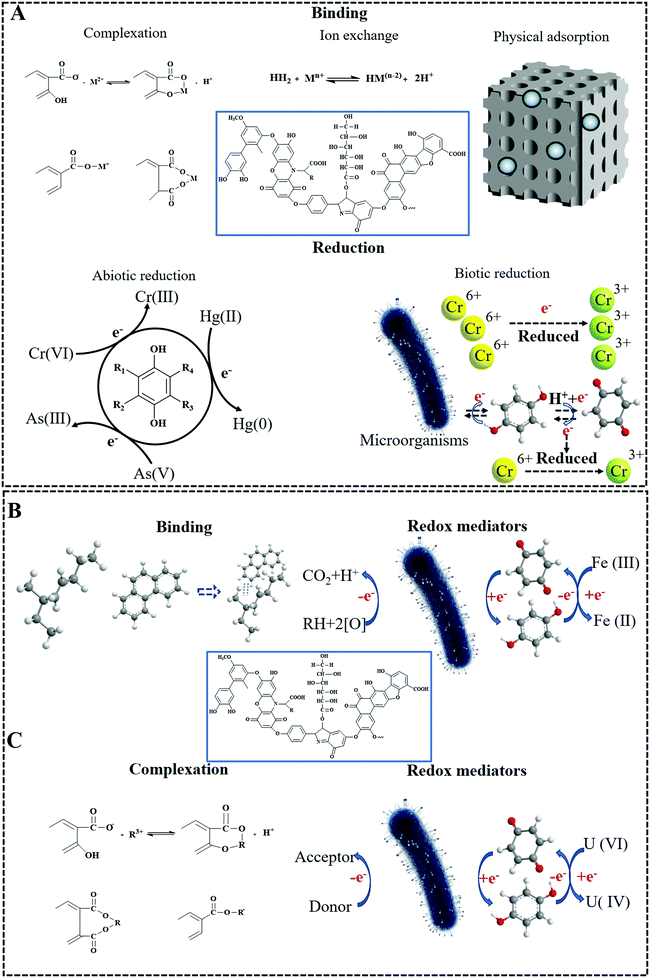 |
| Fig. 6 The mechanism and the effects of humic substances on eco-toxins (A: heavy metals,111 figures have been adapted from ref. 111 with permission from Elservier, copyright 2019; B: organic contaminants; C: radionuclides). | |
3.2.1 Heavy metal binding.
Immobilization of heavy metals by HS is mainly through two ways, binding (complexation, ion exchange, and physical adsorption) or redox reaction126–128 (Fig. 6A). Binding heavy metals can fix and further reduce their fluidity in the soil, while the redox reaction with heavy metals can reduce or eliminate their toxicity. One of the important pathways of binding is complexation, in which carboxylic (–COOH) and phenolic (–OH) groups of HS are mainly involved in the formation of metal-humic complexes.129 Weng et al.130 also demonstrated that HS is more effective in complexing with Pb and Cu than with Cd, Zn, and Ni in aqueous soils at pH 3.7–6.1. Electrostatic interactions are also a way for HS to bind heavy metals.131 Wei et al.132 observed that heavy metals (Cu2+, Zn2+, and Cd2+) were bound to HS and proteins via Coulomb forces. HS was discussed to be redox active (the reduction potential of HS is from 0.15 to −0.3 V in neutral environments), making it relevant in geochemical and environmental processes.87,133,134 Tipping and co-workers27 listed several redox reactions on the reduction of heavy metals involving HS in the book Cation binding by humic substances, such as Cr(VI) to Cr(III), and Hg(II) to Hg(0). Peat and coal-derived HSs were used as reducing agents to reduce toxic Cr(VI) to non-toxic Cr(III) by Zhilin et al.15 The detailed results demonstrated that coal-based HS has long-lasting capabilities for reducing Cr(VI) to Cr(III), although, its capacity for reduction of Cr(VI) was lower than that of peat-based HS.
The binding capacity of HS to metal ions, including heavy metals, can be further quantified by a variety of data from the literature. Ghabbour et al. devoted numerous time to study the metal-binding capacity of solid soil-derived HA (NHA), such as Fe(III), Pb(II), Cu(II),135 Mn(II), Co(NH3)6aq3+, and Hg(II)136 based on earlier work on Ca(II), Mg(II), and Co(II).137 In detail, the highest binding capacity of NHA with Fe(III), Pb(II), Cu(II), Mn(II), Co(NH3)6aq3+, Hg(II), Ca(II), Mg(II) and Co(II) reached 0.9 mmol g−1, 2.0 mmol g−1, 1.4 mmol g−1, 1.5 mmol g−1, 0.2 mmol g−1, 1.52 mmol g−1, 0.53 mmol g−1, 0.36 mmol g−1 and 1.7 mmol g−1, respectively. The higher numbers are in the range of industrial ion exchange resins, i.e. in terms of their sustainability humics are performing rather well. The metal-binding ability of synthetic HS has also been studied. The results showed that the non-soil-derived HS, when appropriately synthesized, had an even higher metal-binding capacity. For example, Wei et al.132 found that the adsorption capacity of humic acid isolated from activated sewage sludge to Zn(II), Cu(II) and Cd(II) reached 2597.9 mg g−1, 2013.5 mg g−1 and 1633.5 mg g−1, respectively; this is for Zn(II) 40 mmol g−1, i.e. the binding capacity of A-HA is really extraordinary and by far exceeds those of even specialized ion exchange resins. Notably, no difference on the detoxification of Cu/Zn (transition metals) and Cd by the fraction of HS was found in the above investigation, which is attributed to electrostatic counterion binding. It is worth noting that HS has strong, substantial chelation properties, which by the rules of ligand chemistry are more effective for transition metals rather than other metals.
Ion binding is not limited to applying humic substances to salt containing solutions for coulombic binding, but allows an advanced technique we call “reactive removal” of heavy metals from water and waste biomaterials, such as sludge, while preparing the A-HS. The work done by Zhang138 suggested that hydrothermal humification (HTH) technology can not only prepare HA fertilizer, which is beneficial to the growth of crops, but also removes at the same time heavy metals from the sludge effectively. For example, the content of Cu, Zn and Pb in densified waste sharply dropped from 0.46, 0.98 and 0.14 to 0.07, 0.15 and 0.07 mg g−1, respectively. Such concepts can be transferred back to aqueous phases. Our own work139 on the absorption of heavy metal ions by A-HS, such as the binding of Pb2+ by A-HS, revealed that the combination of A-HS and nano-sized zero-valent iron displayed a superior kinetic removal efficiency of Pb(II) (>99.2%), and a huge Langmuir removal capacity, reaching 649.0 mg g−1, which exceeds the previous gold standard of 2.0 mmol g−1, i.e. 414.4 mg g−1, the results produced by Ghabbour et al.135
In addition to the abiotic chemical reduction of heavy metals, the redox potential of HS can be mediated by microorganisms under anaerobic conditions, which is a very effective approach for reducing pollution. Work done by Gu's group140 also revealed that HS can act as a redox mediator, i.e., HS participates in the process of heavy metal reduction by microorganisms as an electron transporter. The results indicated that soil HA had a good mediating effect on the reduction of Cr(VI) and U(VI) by microorganisms under anaerobic, circumneutral pH conditions. Taking Cr(VI) as an example, regardless of the presence of HS, Cr(VI) can be directly reduced by Deinococcus radiodurans in anaerobic culture with lactate, while the presence of HS will enhance the reduction efficiency of Cr(VI), as HS acts as an electron mediator in the process.
3.2.2 Organic contaminants.
The mechanisms on removal of organic contaminants by HS in actual applications are also diverse and can be summarized as follows (Fig. 6B). Firstly, the structure of aliphatic substructures are mostly responsible for the hydrophobic property of HS, and the organic contaminants adhere on those pools.141,142 Mao et al.143 demonstrated that the amorphous aliphatic fraction of HS does have the function to bind organic contaminants. They chose phenanthrene as a model hydrophobic organic contaminant, and studied the sorption behavior on HS. The sorption capacity of HS was quantified by a modified Freundlich coefficient (Kf′), while the compositional data of HA, HM, and a peat were obtained from quantitative 13C solid-state NMR spectroscopy. Their results showed that Kf′ was strongly related to the amorphous nonpolar aliphatic domains formed by fatty acid tails, but not to aromaticity or polarity indices. Secondly, HS promotes reductive biodegradation by transferring electrons from microorganisms to various oxidized organic pollutants, due to its redox properties, that is, HS is involved as a redox mediator.144–146 This process is essentially a continuation of the discussion that HS promotes the reduction of Fe(III) by reducing microorganisms (a detailed description is displayed in Section 3.1.5), while Fe(III) species are the most abundant and potential electron acceptors for the anaerobic oxidation of organic contaminants: as a result, the degradation rate of organic contaminants in the experiments is largely improved. In addition, the reductive antioxidant activity of HS enables its function as a scavenger of free radicals produced by herbicides and other organic contaminants.147 The scavenging activity of HS has a positive correlation with HS concentration.
3.2.3 Radionuclides.
The complexation and redox properties of HS can also alleviate the contamination of soil caused by radionuclides.148–150 A series of studies have confirmed that HA can form ternary complexes with radionuclides in various valence states by hydroxide or carbonate complexation, such as Cm(III), Th(IV), U(VI), Cr(VI) and others.151–154 The method of time-resolved laser induced fluorescence (TRLIF) was specifically applied by Panak et al.154 to detect the stability constants for Cm(III)-HA complexes. TRLIF results showed that Cm(III) formed three complexes with HA, which were Cm(OH)HA, Cm(OH)2HA and Cm(CO3)HA, and the stability constants for the formation of Cm(OH)HA, Cm(OH)2HA and Cm(CO3)HA were 6.94 ± 0.04, 4.2 ± 0.2 and 6.50 ± 0.03, respectively. Reiller et al.153 also reported that the presence of HS has a positive effect on the retention of Th(IV) onto the surface of silica colloids. In addition, HS can transform the radionuclides from the soluble state to the insoluble state by reduction. For example, HS is also involved as an electron donor in the D. radiodurans reduction of U(VI) and Tc(VII).155Fig. 6C shows the reduction principle of U(VI) to U(IV).
4. Advances in synthesis and application of artificial humic substances (A-HS)
Practically all HS species mentioned in the previous sections refer to those formed in natural environments such as soil, peatland, or lakes.156 This comes with obvious restrictions for larger scale application considering the available resources, effective utilization schemes and environmental protection. It obviously makes little sense to plunder one ecological environment to cure the other. The unpredictable diversity and structural heterogeneity of natural compounds in general are also factors restricting predictable wider applications of HS.37 To deal with these problems, some research groups have developed synthetic methods to generate A-HS. This research is very promising, as indeed most of the described properties of HS could be replicated in A-HS, while the starting compounds were mostly taken from omnipresent plant and agricultural side products, or “waste biomass”. In addition, it allows the formation of “designer-humins” for scientific cross-tests, for instance nitrogen-free humins or humins unusually rich in fatty acid residues. In the following sections, we will review the current developments in A-HS synthesis and applications.
4.1 Hydrothermal humification technology (HTH)
The group of Yang23 was the first to develop a chemical synthesis method to prepare A-HS through hydrothermal processing of plant residues in the presence of ashes or bases, creating an accelerated artificial humification technology. Bento, a driver in hydrothermal carbonization, evaluated HTH as “HTH may be a new technological means of producing HS similar to those found in soil for use as plant growth promotors”.157 Hydrothermal processing under autogenous pressure of wet biomass can be considered a mild chemical process simulating natural coalification or humification, but with an acceleration factor on time of up to 109. In addition, as an abiotic process, hydrothermal processing usually achieves excellent carbon yields: the majority of the carbon bound in biomass ends up in the humified product. Notably, the invention of HTH provided guidance and reference for later synthesis technologies of A-HS.157,158
4.1.1 The route of A-HS synthesis.
The specific process is shown in the following Fig. 7.
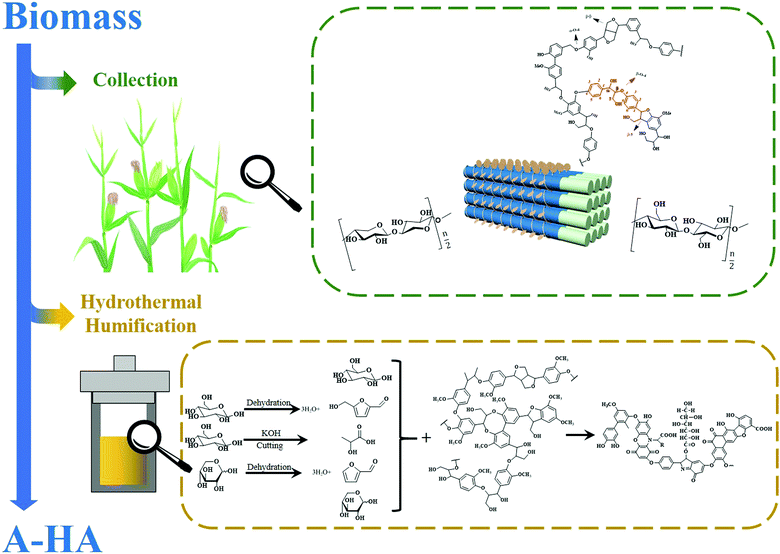 |
| Fig. 7 The preparation scheme of artificial humic substances23 (figures have been adapted from ref. 23 with permission from Elsevier, copyright 2019). | |
4.1.2 The structure characteristics of A-HS.
Yang et al. summarized the structural characteristics of AHS in a previous review,10,22 and the schematic structure formula in Fig. 2, which agrees with the analytical data is set into a mechanistic context in Fig. 8.
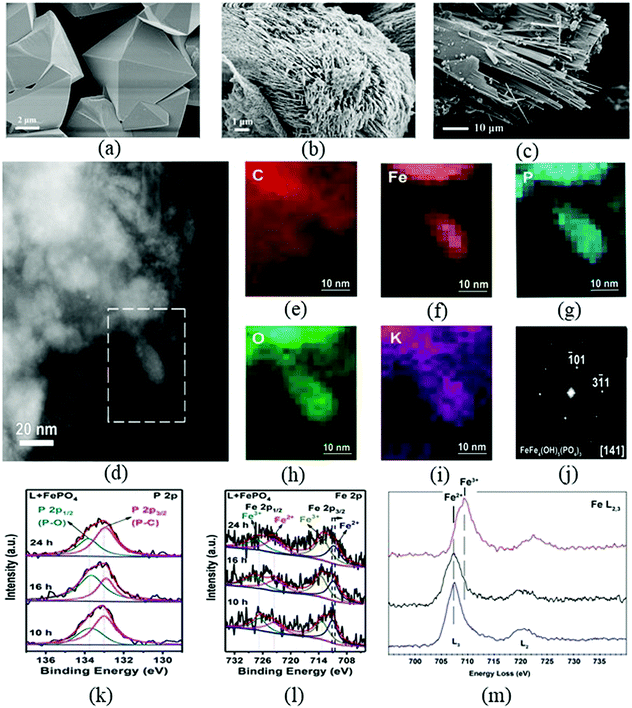 |
| Fig. 8 SEM images of original insoluble phosphate crystals (a) and those reconstituted by humic acids from leaves (b and c); (d) ADF-STEM image of FePO4 samples after A-HA etching; (e–i) EDX maps representing signals of C, Fe, P, O and K, collected from the area marked by the dotted line in (d) and the overall EDX spectrum of this area; (j) the fast Fourier transform curve of FePO4 samples after A-HA etching; (k) P 2p and (l) Fe 2p spectra of FePO4 samples after A-HA etching with different time; (m) Electron Energy Loss (EEL) spectra collected from FePO4 samples after A-HA etching160 (figures have been adapted from ref. 160 with permission from Wiley, copyright 2019). | |
4.1.3 Advance in the applications of A-HS.
Based on the development of HTH, initial but indicative research work on the effect of A-HS on agricultural production and environmental remediation was performed. We will summarize here the results of the application of A-HS according to the different application fields.
(1) Agricultural production.
Initially, work was done exploring the influence of A-HS on land restoration.159 The synthetic method of artificial soil was put forward, i.e. diverse starting biomass was mixed with soil, then HTH was used to obtain the artificial soil by colloidal surface conjugation of as-prepared A-HS with soil minerals. It was shown that the as-created artificial soil organic matter (A-SOM) does closely bind to the activated mineral surfaces, thus forming “artificial soil” (AS), according to the examination of the structure and morphology of soil particles. Not only that, humification increases the function of hydroxyl on the mineral surface, making it more hydrophilic, and the subsequent formation of A-HS promotes the close binding of organic substances to the mineral surface. Worth noting, artificial soil had a similar water retention capacity as compared to that of black soil (collected in Harbin, China), and the adsorption capacity of 40 g dry artificial soil of N, P and K dispersed in 200 mL was found to be 0.55 g, 1.60 g and 1.23 g, respectively. These values are 7.58, 1.32 and 78.78 times more than the original values of sandy soil (original soil).
It was already discussed that P is one of the most important elements in the biosphere and agriculture, especially for promoting plant growth.78 Available phosphate (AP) is a main bottleneck restricting crop growth in terrestrial ecosystems.65 The solubilization of insoluble phosphorus (P) by A-HS was reported.160 As seen, A-HS derived from high lignin containing dried leaves results in a profound disintegration and restructuration of the crystalline bodies (Fig. 8a) into a “sponge” – or “noodle” like structure (Fig. 8b and c). Detailed electron microscopy and spectrum analysis confirmed the etching of primary iron phosphate crystals by carboxyl and phenolic groups of A-HA and demonstrated the importance of the redox property of A-HA on the nanometre scale (Fig. 8d–m).
Planting experiments nicely underlined that A-HA was capable of dissolving insoluble phosphate minerals into AP for plant growth and increase water content in the roots and stems of plants. Du et al.161 also used animal bone as a precursor of biomass to synthesize A-HA. Notably, the bones dispersed completely in only 12 h, and A-HA was able to solubilize the insoluble P present in animal bones into dissolved phosphorus (DP) up to 98.65 mg L−1, accounting for 6.48% of total phosphorus (TP) in the bone waste. This action not only solves the environmental issues caused by kitchen waste, but also can provide a secondary phosphorus source to increase AP content in soil.
Based on the above research results, our group162 further studied the effect of A-HS on soil improvement in detail, including cation exchange amount, carbon fixation, migration distance of AP and bacterial abundance. The combination of three-dimensional excitation–emission matrix (3D-EEM) fluorescence spectroscopy, synchronous fluorescence spectroscopy (SFS) and principal component analysis (PCA) was used to reveal the induced changes of the soil ecosystem with time. Results demonstrated that the content of dissolved organic matter (DOM) greatly increases in AHA treated groups, accompanied with the increase of total organic carbon (TOC) and dissolved organic carbon (DOC). PCA analysis revealed that TOC, DOC, bacterial abundance, migration distance of AP and total exchangeable bases (TEBs) also significantly increased. The highest improvement of TOC, DOC, bacterial abundance, and migration distance of AP is up to 17.7%, 24.1%, 203.5%, and 66.7% after 28-day cultivation, respectively, and TEB can reach 11.3% after 7-day cultivation. Surprisingly, the carbon sequestration capacity of A-HS is so strong that it can be calculated so that the carbon increment can reach up to 21.4 g kg−1. And several hypotheses can be proposed for the huge increase in soil carbon content: (1) biological photochemistry: A-HS promoted the growth of autotrophic bacteria, which are able to fix carbon from CO2; (2) pure chemistry: the redox properties of A-HS directly allow it to fix CO2; (3) hybrid photocatalysis: A-HA can photoreduce itself, and part of the microbes are feeding on the redox equivalents and converting CO2, without being able to do photocatalysis themselves.
(2) Environmental remediation.
As is well known, nano-sized zero-valent iron (nZVI) particles containing composite materials have a broad application prospect because of their comparably low costs and high removal efficiency of heavy metals.163 However, application of nZVI in environmental applications has a very serious problem, i.e., Fe nanoparticles are prone to oxidation, corrosion and aggregation into large particles due to their high surface energies, chemical reactivity and intrinsic magnetic interactions.164 Improving oxidation stability of nZVI in natural environments is a new problem. In a research context, our previous work139 came up with the hypothesis that the affinity of humus to metal ions and its inherent reduction power can be used to enwrap and stabilize nZVI. A novel hybrid material with highly dispersed nZVI stabilized by A-HA (AHA-nZVI) was made by hydrothermal humification (HTH) technology. The experimental results showed that the introduction of AHA can effectively minimize oxidation and agglomeration of nZVI. Moreover, a superior kinetic removal efficiency of Pb2+ (>99.2%) was described, and a huge Langmuir removal capacity was found, reaching 649.0 mg g−1, according to multiple interaction mechanisms. The process progresses in a multifarious way and involves reduction reactions, complexation and co-precipitation between heavy metals and AHA-nZVI samples. The results provide a reference for strengthening the long-time adsorption of heavy metals by other materials with A-HA as a non-innocent support.
Our group is committed to the utilization of biomass side products, but also biological wastes for the achievement of environmental remediation. Based on the HTH method, Zhang et al.138 proposed a simple and low-cost scheme using sewage sludge (SS), alkali ash and biomass as precursors for the synthesis of liquid fertilizer rich in AP and A-HS, realizing a near-complete recovery of valuable phosphate and an “all-waste-strategy” route for sludge valorization. The removal efficiency of heavy metals and the recovery efficiency of P were also studied. It was found that the introduction of biomass into sludge-derived samples and adjustment of KOH or alkali ash mass effectively improved the recovery of P element and achieved a high content of dissolved phosphorus (DP) (from 7045 mg L−1 to 10
075 mg L−1) at appropriate pH values (6.5–7.7). HTH also solves the problem of a potentially too high heavy metal content in sludge, as they are concentrated in the solid residues of the process. ICP-AES results showed that the content of Cr and Cd was so low that it could not be detected, and the content of Cu, Zn and Pb sharply decreased from 0.46, 0.98 and 0.14 to 0.07, 0.15 and 0.07 mg g−1, respectively, in sludge-derived liquid products. The solid side product then can be separately used for heavy metal recycling. The results of pot planting experiments indicated that the product is a very effective liquid fertilizer.
4.2 Hydrothermal carbonization technology (HTC)
HTC was reinvented in history not only once but several times. Bento stated: “The inspiration for HTC came from the presence of anthropogenic organic matter in highly fertile Amazonian Dark Earth (ADE), which is attributed to the transformation of organic matter over thousands of years”.157 The synthesized humic-like substance (HLS) was for instance obtained by HTC with bagasse and vinasse as precursors. HTC promotes the recycling of biowaste into organic soil conditioners, thereby transforming waste management issues into economic opportunities to improve environmental resilience and promote agricultural economic recycling.
4.2.1 Humic-like substances from HTC.
The synthesis of HLS mainly consists of two steps: synthesis and extraction. In brief, hydrochar was synthesized from HB/HV/HB + HV under the conditions of 230 °C and 13 h. Then, the HLS were extracted by NaOH.
4.2.2 The structure characteristics of HLS.
Bento et al.157 compared the structure of the synthesized HLS with that extracted from the soil. The results of elemental and UV-vis analyses are displayed as follows:
(i) HLS samples are rich in carbon (63.0 to 67.0%), while the carbon content of all HLS samples was more similar to that of Amazonian Dark Earth-derived HS (HS-ADE); (ii) the addition of H3PO4 decreased HLS nitrogen content (increase of the C/N ratio) and the ratio of E4/E6, a measure of aromaticity;165 (iii) soil-derived HSs (HS-ADE and HS-SR) are more hydrophilic than HLS, showing lower H/O ratios and higher O/C ratios.
The products were further analysed by fluorescence analysis, 13C CP-MAS NMR, and offline thermochemolysis GC-MS. For more detail we suggest the original articles. The different products were then tested in the germination of maize seeds. The results suggested that HLS with abundant phenolic moieties promoted the germination of maize seeds more than those samples rich in alkyl compounds.
4.3 Hydrogen peroxide oxidation technology (HOT)
A synthesized “humic-like acid” material (HLA) was obtained by HOT from biomass pretreatment liquor in alkaline solution as a precursor.158 The intention of the authors by integration of this step was to promote cleaner production processes in a biorefinery, the circular economy of agriculture and a proof-of- concept for resource recovery.
4.3.1 The route of HLA synthesis.
Compared with the above two hydrothermal synthesis techniques (HTH and HLS), HLA has one more step of pretreatment. Firstly, a pretreatment liquor was obtained by continuous treatment of biomass with NaOH at 130° for 0.5 h, i.e. in HTH this corresponds to artificial fulvic acid formation.23 Then, 8% H2O2 was applied to synthesize HLA by oxidation of the pretreatment liquor as the precursor. Finally, the rather pure HLA was extracted with HCl. As compared to AHA technology, this process increases the carboxylate content by peroxide oxidation.
By detecting the contents of total phenols, total sugars, proteins and amino acids in the pretreatment solution and HLA, it was found that the recovery rates of these substances reached 82.8%, 56.2%, 78.1% and 64.2%, respectively. At the same time, Wang et al.158 compared the structure of HLA with that of commercial humic acid (CHA). The results showed that HLA has a more uniform polymer size and higher C (22.5%), N (2.3%), O (33.8%), K (4.5%), and P (11.2%) contents. The hydroxyl and carboxyl group contents in HLA were 3.3 and 2.0 times higher than that in CHA, respectively.
In addition to the analysis of the structure of HLA, Wang et al.158 also studied the electron transfer capacity of HLA and the effect of HLA on the binding of heavy metals. They discovered158 that HLA has a higher electron transferring capacity. The findings depicted that the electron donating capacity (EDC) and electron accepting capacity (EAC) values of HLA were slightly higher than those of CHA. The specific EDC and EAC values of HLA are 630 to 1102 mmol (gC)1 and 450 to 890 mmol (gC)1, respectively. The specific EDC and EAC values of CHA are 480 to 870 mmol (gC)1 and 401 to 810 mmol (gC)1, respectively. The results of metal-binding potential of HLA revealed that HLA has a greater binding ability for metals than CHA.
5. Conclusion and outlook
We summarized in the first part how as-extracted humic substances can be beneficially used in agricultural production and environmental remediation, while we focused on the underlying molecular mechanisms and colloidal processes. Based on its special structure and superior properties, HS can be widely applied for binding pollutants (cleaning water resources), improving availability of soil nutrients (ensuring food security), softening and opening soil texture (increasing soil quality), regulating carbon sequestration (controlling global warming), promoting plant and microbial growth (enhancing soil micro-environment) and so on (as described in Fig. 9).
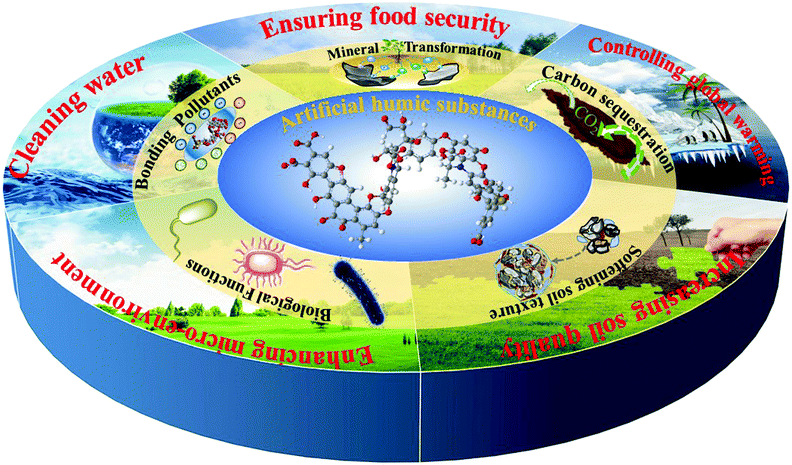 |
| Fig. 9 Application and prospective uses of humic substances. | |
Most of the previous HS technologies are based on humic acids extracted by chemical processes from natural resources, e.g. peat or lignite. This is why we presented in the second part recent, sometimes very efficient techniques of chemical HS-synthesis, including HTH, HTC and HOT, and analyzed the advances of such A-HS tailor-made from specific biomass and by specific processes, to the respective applications. The three technologies have a common feature, namely, they are based on biomass side streams and biowaste, such as corn straw or sewage sludge. From these resources, A-HS can be effectively synthesized, and the products have indeed high similarity with naturally-extracted HS.
The actual research already proves that A-HS greatly contributes to solubilize insoluble phosphate, to promote the recovery of phosphate lost in previous generations, to improve soil fertility for crop growth, and to reduce toxicity of typical pollutants. It can be speculated that HTH technology and the as-prepared A-HS open even more radiating development prospects, such as terraforming wasteland or making inner cities more green, clean water, and of course improving food security.
From a chemical perspective, humic acids are amphiphilic polymers, which stabilize many interfaces, and thereby we see larger perspectives in particle stabilization, but also nanoparticle bridging and immobilization, where the specific performance and selectivity can – in synthesized humic acids – be addressed and optimized by composition and molecular weight. Using humic acids for this stabilization and destabilization is especially relevant in environmentally open applications, e.g. mining and the treatment of industrial sludges such as power plant ashes. This can be even extended to oral drug delivery, where humic acids and fulvic acids are already favourably explored to deliver hardly soluble actives.166 It should however be also mentioned that humic matter even without added drug molecules is part of many treatment protocols in traditional medicine, e.g., against dermal diseases, and inflammatory afflictions, to improve gastrointestinal function and thereby finally indirectly fight diabetes.167
Humic matter is in the very end a group of biological polymers, and modifications in any chemical direction can be performed using the ballpark of classical polymer chemistry. Grafting for instance polystyrene onto a humic acid backbone improves the binding of organic substances by several orders of magnitude.168 From a polymer perspective, humic matter free of inorganic salts and particles is a very affordable, bio-sourced polyacid, and after esterification the resulting products are biodegradable, thermoplastic polymers with a glass transition around 100 °C, which can be turned into “humin-plastic” products, which can be left in the environment, e.g. planting pots, geofabrics, and even as golf tees. There are other clear visions in fields of industrial chemicals, medicine, or phytohormones, but these are still further in the future.
The purpose of this review is to make more people aware of the possibility of preparing A-HS from biomass side streams with great variations on a cost level well below that of extraction, with the potential benefits being well documented already. We can only hope that the availability of such condensed information will make more people interested in this special class of sustainable polymers with unparalleled redox capacities, and unexpected biological interactions, as well as a base for a new agricultural chemistry.
Conflicts of interest
There are no conflicts to declare.
Acknowledgements
The authors thank Prof. Kui Cheng and Mr. Yibo Lan for helpful discussions of the text and figures. The authors appreciate the financial support from Longjiang Scholar for Young Scientist, the open funding of State Key Laboratory of Geomechanics and Geotechnical Engineering (Z019005), the Natural Science Foundation of Heilongjiang Province of China (QC2018019), and the financial support from University Nursing Program for Young Scholars with Creative Talents in Heilongjiang Province (UNPYSCT-2017018). Open Access funding provided by the Max Planck Society.
Notes and references
-
E. A. Ghabbour and G. Davies, Humic Substances: Structures, Properties and Uses, Woodhead Publishing, England, 2014 Search PubMed.
- S. C. B. Myneni, J. Brown, G. Martinez and W. Meyer-Ilse, Science, 1999, 286, 1335–1337 CrossRef CAS PubMed.
-
I. V. Perminova, K. Hatfield and N. Hertkorn, Use of humic substances to remediate polluted environments: from theory to practice, Springer, Netherlands, 2005 Search PubMed.
- J. Lehmann and M. Kleber, Nature, 2015, 528, 60–68 CrossRef CAS PubMed.
- J. Hedges and J. Oades, Org. Geochem., 1997, 27, 319–361 CrossRef CAS.
- P. Puget, C. Chenu and J. Balesdent, Eur. J. Soil Sci., 2000, 51, 595–605 CrossRef.
-
N. C. Brady, R. R. Weil and R. R. Weil, The nature and properties of soils, Prentice Hall, Upper Saddle River, NJ, 2008 Search PubMed.
-
N. Senesi, Molecular Environmental Soil Science at the Interfaces in the Earth's Critical Zone, Springer, 2010, pp. 249–250 Search PubMed.
- R. Lal, Science, 2004, 304, 1623–1627 CrossRef CAS PubMed.
- F. Yang and M. Antonietti, Adv. Sci., 2020, 7, 1–7 Search PubMed.
-
F. J. Stevenson, Humus chemistry: genesis, composition, reactions, John Wiley & Sons, 1994 Search PubMed.
-
M. Schnitzer, Developments in soil science, Elsevier, 1978, vol. 8, pp. 1–64 Search PubMed.
-
R. P. Schwarzenbach, P. M. Gschwend and D. M. Imboden, Environmental organic chemistry, John Wiley & Sons, New York, 2016 Search PubMed.
-
M. T. Rose, A. F. Patti, K. R. Little, A. L. Brown, W. R. Jackson and T. R. Cavagnaro, Advances in agronomy, Elsevier, 2014, vol. 124, pp. 37–89 Search PubMed.
- D. M. Zhilin, P. Schmitt-Kopplin and I. V. Perminova, Environ. Chem. Lett., 2004, 2, 141–145 CrossRef CAS.
- B.-Q. Lin and J.-H. Liu, Energy Policy, 2010, 38, 512–519 CrossRef.
- J. Santos, L. Nunes, W. Melo and A. Araújo, Eur. J. Soil Biol., 2011, 47, 146–151 CrossRef.
- J. Wu, Y. Zhao, H. Qi, Z. Wei, X. Zhao, T. Yang, Y. Du and H. Zhang, Bioresour. Technol., 2017, 244, 1193–1196 CrossRef CAS PubMed.
- J.-H. Hsu and S.-L. Lo, Environ. Pollut., 1999, 104, 189–196 CrossRef CAS.
- M. Imbeah, Bioresour. Technol., 1998, 63, 197–203 CrossRef CAS.
- J. Wong, G. Li and M. H. Wong, Bioresour. Technol., 1996, 58, 309–313 CrossRef CAS.
- F. Yang and M. Antonietti, Prog. Polym. Sci., 2020, 100, 101182 CrossRef CAS.
- F. Yang, S. Zhang, K. Cheng and M. Antonietti, Sci. Total Environ., 2019, 686, 1140–1151 CrossRef CAS PubMed.
- C. Saiz-Jimenez and J. W. D. Leeuw, J. Anal. Appl. Pyrolysis, 1987, 11, 367–376 CrossRef CAS.
-
M. H. B. Hayes and R. Swift, The Chemistry of Soil Organic Colloids, 1978, pp. 179–320 Search PubMed.
- L.-M. Huang, X.-H. Zhang, M.-A. Shao, D. Rossiter and G.-L. Zhang, Geoderma, 2016, 274, 45–53 CrossRef CAS.
-
E. Tipping, Cation binding by humic substances, Cambridge University Press, Cambridge, UK, 2002 Search PubMed.
- A. Bauer and A. Black, Soil Sci. Soc. Am. J., 1992, 56, 248–254 CrossRef.
- K. A. Farley, E. F. Kelly and R. G. Hofstede, Ecosystems, 2004, 7, 729–739 CrossRef.
- J. M. Tisdall and J. M. Oades, J. Soil Sci., 1982, 33, 141–163 CrossRef CAS.
-
R. Swift, Advances in Soil Organic Matter Research, 1991, pp. 153–162 Search PubMed.
- A. Piccolo, G. Pietramellara and J. Mbagwu, Geoderma, 1997, 75, 267–277 CrossRef CAS.
- A. Mamedov, B. Bar-Yosef, I. Levkovich, R. Rosenberg, A. Silber, P. Fine and G. Levy, Soil Res., 2014, 52, 317–326 CrossRef.
- W. Ouyang, Y. Wu, Z. Hao, Q. Zhang, Q. Bu and X. Gao, Sci. Total Environ., 2018, 613, 798–809 CrossRef PubMed.
- X. Xu, Y. Xu, S. Chen, S. Xu and H. Zhang, Environ. Sci. Policy, 2010, 13, 793–800 CrossRef CAS.
-
M. Schnitzer and C. Fuchsman, Water retention by humic substances, Elsevier Applied Science Publishers, London, 1986 Search PubMed.
- Z. Cihlář, L. Vojtová, P. Conte, S. Nasir and J. Kučerík, Geoderma, 2014, 230, 151–160 CrossRef.
- A. Piccolo, G. Pietramellara and J. Mbagwu, Soil Use Manage., 1996, 12, 209–213 CrossRef.
- T. Sinore, E. Kissi and A. Aticho, Int. Soil Water Conservat. Res., 2018, 6, 123–130 CrossRef.
- Y. Khaledian, E. C. Brevik, P. Pereira, A. Cerdà, M. A. Fattah and H. Tazikeh, Catena, 2017, 158, 194–200 CrossRef CAS.
-
W. Mindari, P. E. Sasongko, Z. Kusuma, Syekhfani and N. Aini, presented in part at the AIP Conference Proceedings, 2018.
- H. Xu, Z. Yan, H. Cai, G. Yu, L. Yang and H. Jiang, Ecotoxicol. Environ. Saf., 2013, 98, 266–272 CrossRef CAS PubMed.
- S. Yang, Z. Zhang, L. Cong, X. Wang and S. Shi, J. Soil Sci. Plant Nutr., 2013, 13, 526–533 Search PubMed.
- M. H. B. Hayes and C. E. Clapp, Soil Sci., 2001, 166, 723–737 CrossRef CAS.
- J. Rogelj, M. Schaeffer, P. Friedlingstein, N. P. Gillett, D. P. Van Vuuren, K. Riahi, M. Allen and R. Knutti, Nat. Clim. Change, 2016, 6, 245–252 CrossRef.
- C. McGlade and P. Ekins, Nature, 2015, 517, 187–190 CrossRef CAS PubMed.
- G. Luderer, Z. Vrontisi and C. Bertram, Nat. Clim. Change, 2018, 8, 626 CrossRef CAS.
- C. J. Smithes, P. M. Torstcr, M. Allen, J. Fuglestvedt, R. J. Millar, J. Rogelj and K. Zlckfeld, Nat. Commun., 2019, 10, 1–10 CrossRef PubMed.
- R. Heede and N. Oreskes, Global Environmental Change Part A: Human & Policy Dimensions, 2016, 36, 12–20 Search PubMed.
- M. G. Kramer and O. A. Chadwick, Nat. Clim. Change, 2018, 8, 1104–1108 CrossRef CAS.
-
D. Qin, G. Plattner, M. Tignor, S. Allen, J. Boschung, A. Nauels, Y. Xia, V. Bex and P. Midgley, Climate change 2013: the physical science basis, 2014 Search PubMed.
- J.-F. Soussana, S. Lutfalla, F. Ehrhardt, T. Rosenstock, C. Lamanna, P. Havlík, M. Richards, J.-L. Chotte, E. Torquebiau and P. Ciais, Soil Tillage Res., 2019, 188, 3–15 CrossRef.
- H. Nan, L. Zhao, F. Yang, Y. Liu, Z. Xiao, X. Cao and H. Qiu, J. Cleaner Prod., 2020, 255, 120162 CrossRef CAS.
- P. Smith, Global Change Biology, 2016, 22, 1315–1324 CrossRef PubMed.
- M. Li, H. Hu, X. He, J. Jia, M. Drosos, G. Wang, F. Liu, Z. Hu and B. Xi, J. Agric. Food Chem., 2019, 67, 3106–3113 CrossRef CAS PubMed.
- M. Tatzber, M. Stemmer, H. Spiegel, C. Katzlberger, G. Haberhauer and M. H. Gerzabek, Sci. Total Environ., 2008, 406, 256–268 CrossRef CAS PubMed.
- O. Mašek, W. Buss, P. Brownsort, M. Rovere, A. Tagliaferro, L. Zhao, X. Cao and G. Xu, Sci. Rep., 2019, 9, 1–8 Search PubMed.
- R. S. Swift, Soil Sci., 2001, 166, 858–871 CrossRef CAS.
- J. D. Jastrow, C. Liang and J. P. Schimel, Nature Microbiol., 2017, 2, 17105 CrossRef PubMed.
- S. A. Vissera, Soil Biol. Biochem., 1985, 17, 457–462 CrossRef.
- G. Vallini, A. Pera, M. Agnolucci and M. M. Valdrighi, Biol. Fertil. Soils, 1997, 24, 243–248 CrossRef CAS.
-
E. Ronen, Practical Hydroponics and Greenhouses, 2016, vol. 35 Search PubMed.
- J. Zhu, M. Li and M. Whelan, Sci. Total Environ., 2018, 612, 522–537 CrossRef CAS PubMed.
- D. Cordell and S. White, Annu. Rev. Environ. Resour., 2014, 39, 161–188 CrossRef.
- E. Hou, S. Tang, C. Chen, Y. Kuang, X. Lu, M. Heenan and D. Wen, Geoderma, 2018, 313, 172–180 CrossRef CAS.
- C. Salm, J. C. Middelkoop, P. A. I. Ehlert and M. Goss, Soil Use Manage., 2017, 33, 2–12 CrossRef.
- Z. Yuan, S. Jiang, H. Sheng, X. Liu, H. Hua, X. Liu and Y. Zhang, Environ. Sci. Technol., 2018, 52, 2438–2450 CrossRef CAS PubMed.
- M. E. Schipanski and E. M. Bennett, Ecosystems, 2012, 15, 256–268 CrossRef CAS.
-
M. PRud’hoMMe, presented in part at the International Fertilizer Industry Association, Phosphates 2010 International Conference, 2010.
- D. A. Vaccari, S. M. Powers and X. Liu, Environ. Sci. Technol., 2019, 53, 10417–10425 CrossRef CAS PubMed.
- E. Viruel, M. E. Lucca and F. Siñeriz, Arch. Microbiol., 2011, 193, 489–496 CrossRef CAS PubMed.
- W.-F. Cong, L. D. Suriyagoda and H. Lambers, Trends Plant Sci., 2020, 25, 967–975 CrossRef CAS PubMed.
- M. Clarholm, U. Skyllberg and A. Rosling, Soil Biol. Biochem., 2015, 84, 168–176 CrossRef CAS.
- A. Delgado, A. Madrid, S. Kassem, L. Andreu and M. D. C. D. Campillo, Plant Soil, 2002, 245, 277–286 CrossRef CAS.
- J. D. Filius, J. C. Meeussen, D. G. Lumsdon, T. Hiemstra and W. H. van Riemsdijk, Geochim. Cosmochim. Acta, 2003, 67, 1463–1474 CrossRef CAS.
- B. Gu, J. Schmitt, Z. Chen, L. Liang and J. F. McCarthy, Geochim. Cosmochim. Acta, 1995, 59, 219–229 CrossRef CAS.
- C. N. Guppy, N. Menzies, P. W. Moody and F. Blamey, Soil Res., 2005, 43, 189–202 CrossRef CAS.
- Q. Hua, J. Li, J. Zhou, H. Wang, C. Du and X. Chen, Pedosphere, 2008, 18, 533–538 CrossRef CAS.
- Z. Du, Q. Wang, F. Liu, H. Ma, B. Ma and S. S. Malh, Pedosphere, 2013, 23, 229–235 CrossRef CAS.
- O. K. Borggaard, B. Raben-Lange, A. L. Gimsing and B. W. Strobel, Geoderma, 2005, 127, 270–279 CrossRef CAS.
- M. Olaetxea, D. De Hita, C. A. Garcia, M. Fuentes, R. Baigorri, V. Mora, M. Garnica, O. Urrutia, J. Erro and A. M. Zamarreño, Appl. Soil Ecol., 2018, 123, 521–537 CrossRef.
-
A. Kabata-Pendias, Trace elements in soils and plants, CRC Press, Boca Raton, 2010 Search PubMed.
- C. M. Sharpless and N. V. Blough, Environ. Sci.: Processes Impacts, 2014, 16, 654–671 RSC.
- M. L. Colarieti, G. Toscano, M. R. Ardi and G. Greco, J. Hazard. Mater., 2006, 134, 161–168 CrossRef CAS.
- F. De Cesare, F. Pietrini, M. Zacchini, G. Scarascia Mugnozza and A. Macagnano, Nanomaterials, 2019, 9, 1315 CrossRef CAS PubMed.
- P. Ying, M. Lee, T. Fuji, K. Kikuchi and C. Terao, PLoS One, 2017, 12, e0176484 CrossRef PubMed.
- D. R. Lovley, J. D. Coates, E. L. Blunt-Harris, E. J. Phillips and J. C. Woodward, Nature, 1996, 382, 445–448 CrossRef CAS.
- W. B. Bottomley, Proc. R. Soc. London, Ser. B, 1914, 88, 237–247 CAS.
- D. Pizzeghello, G. Nicolini and S. Nardi, New Phytol., 2002, 155, 393–402 CrossRef CAS PubMed.
- A. Muscolo, F. Bovalo, F. Gionfriddo and S. Nardi, Soil Biol. Biochem., 1999, 31, 1303–1311 CrossRef CAS.
- A. Müller, C. Guan, L. Glweiler, P. Tnzler and K. Palme, EMBO J., 2014, 17, 6903–6911 CrossRef PubMed.
- L. P. Canellas, F. L. Olivares, A. L. Okorokova-Façanha and A. R. Façanha, Plant Physiol., 2002, 130, 1951–1957 CrossRef CAS PubMed.
- L. B. Dobbss, L. O. Medici, L. E. P. Peres, L. E. Pino-Nunes, V. M. Rumjanek, A. R. Façanha and L. P. Canellas, Ann. Appl. Biol., 2007, 151, 199–211 CrossRef CAS.
- S. Trevisan, D. Pizzeghello, B. Ruperti, O. Francioso, A. Sassi, K. Palme, S. Quaggiotti and S. Nardi, Plant Biol., 2010, 12, 604–614 CAS.
- D. Pizzeghello, G. Nicolini and S. Nardi, New Phytol., 2001, 151, 647–657 CrossRef CAS.
- V. Mora, E. Bacaicoa, R. Baigorri, A. M. Zamarreno and J. M. Garcia-Mina, J. Plant Growth Regul., 2014, 33, 430–439 CrossRef CAS.
- V. Mora, E. Bacaicoa, A.-M. Zamarreno, E. Aguirre, M. Garnica, M. Fuentes and J.-M. García-Mina, J. Plant Physiol., 2010, 167, 633–642 CrossRef CAS.
- A. C. García, M. Olaetxea, L. A. Santos, V. Mora, R. Baigorri, M. Fuentes, A. M. Zamarreño, R. L. L. Berbara and J. M. Garcia-Mina, BioMed Res. Int., 2016, 2016, 3747501 Search PubMed.
-
Y. Chen and T. Aviad, Humic substances in soil and crop sciences: selected readings, 1990, pp. 161–186 Search PubMed.
- J. Goldstone, M. Pullin, S. Bertilsson and B. Voelker, Environ. Sci. Technol., 2002, 36, 364–372 CrossRef CAS PubMed.
-
J. Barber, The photosystems: structure, function and molecular biology, Elsevier, 2015 Search PubMed.
- P. Davison, C. Hunter and P. Horton, Nature, 2002, 418, 203–206 CrossRef CAS PubMed.
- E. F. Elstner, Annu. Rev. Plant Physiol., 1982, 33, 73–96 CrossRef CAS.
- J. Foreman, V. Demidchik, J. H. Bothwell, P. Mylona, H. Miedema, M. A. Torres, P. Linstead, S. Costa, C. Brownlee and J. D. Jones, Nature, 2003, 422, 442–446 CrossRef CAS PubMed.
- D. Lu, T. Wang, S. Persson, B. Mueller-Roeber and J. H. Schippers, Nat. Commun., 2014, 5, 1–9 Search PubMed.
- H. Tsukagoshi, W. Busch and P. N. Benfey, Cell, 2010, 143, 606–616 CrossRef CAS PubMed.
- Q. Chen and G. Yang, J. Plant Growth Regul., 2019, 39, 1–15 Search PubMed.
- A. C. García, L. A. Santos, F. G. Izquierdo, V. M. Rumjanek, R. N. Castro, F. S. dos Santos, L. G. A. de Souza and R. L. L. Berbara, J. Geochem. Explor., 2014, 136, 48–54 CrossRef.
- M. Pukalchik, K. Kydralieva, O. Yakimenko, E. Fedoseeva and V. Terekhova, Front. Environ. Sci., 2019, 7, 80 CrossRef.
- X.-X. Guo, H.-T. Liu and S.-B. Wu, Sci. Total Environ., 2019, 662, 501–510 CrossRef CAS PubMed.
- S. Lee, Y. Roh and D.-C. Koh, Chemosphere, 2019, 220, 86–97 CrossRef CAS PubMed.
- J. D. Coates, K. A. Cole, R. Chakraborty, S. M. O'Connor and L. A. Achenbach, Appl. Environ. Microbiol., 2002, 68, 2445–2452 CrossRef CAS PubMed.
- L. Klüpfel, A. Piepenbrock, A. Kappler and M. Sander, Nat. Geosci., 2014, 7, 195–200 CrossRef.
- I. J. Van Trump, K. C. Wrighton, J. C. Thrash, K. A. Weber, G. L. Andersen and J. D. Coates, mBio, 2011, 2, e00044-11 CrossRef PubMed.
- D. T. Scott, D. M. McKnight, E. L. Blunt-Harris, S. E. Kolesar and D. R. Lovley, Environ. Sci. Technol., 1998, 32, 2984–2989 CrossRef CAS.
- D. R. Lovley, J. L. Fraga, J. D. Coates and E. L. Blunt-Harris, Environ. Microbiol., 1999, 1, 89–98 CrossRef CAS PubMed.
-
N. Kulikova, E. Stepanova and O. Koroleva, Use of humic substances to remediate polluted environments: from theory to practice, Springer, 2005, pp. 285–309 Search PubMed.
- E. Lipczynska-Kochany, Chemosphere, 2018, 202, 420–437 CrossRef CAS PubMed.
- M. M. Valdrighi, A. Per, M. Agnolucci, S. Frassinetti, D. Lunardi and G. Vallini, Agric., Ecosyst. Environ., 1996, 58, 133–144 CrossRef.
-
N. Rodríguez-Eugenio, M. McLaughlin and D. Pennock, Soil pollution: a hidden reality, FAO, 2018 Search PubMed.
- Y. Chae and Y.-J. An, Environ. Pollut., 2018, 240, 387–395 CrossRef CAS PubMed.
- Q. Duan, J. Lee, Y. Liu, H. Chen and H. Hu, Bull. Environ. Contam. Toxicol., 2016, 97, 303–309 CrossRef CAS PubMed.
- H. Wu, C. Lai, G. Zeng, J. Liang, J. Chen, J. Xu, J. Dai, X. Li, J. Liu and M. Chen, Crit. Rev. Biotechnol., 2017, 37, 754–764 CrossRef CAS PubMed.
- F. Tong, X. Gu, C. Gu, J. Xie, X. Xie, B. Jiang, Y. Wang, T. Ertunc, A. Schffer and R. Ji, Environ. Sci. Technol., 2016, 50, 6257–6266 CrossRef CAS PubMed.
- N. A. Kulikova and I. V. Perminova, Environ. Sci. Technol., 2002, 36, 3720–3724 CrossRef CAS PubMed.
- W.-W. Tang, G.-M. Zeng, J.-L. Gong, J. Liang, P. Xu, C. Zhang and B.-B. Huang, Sci. Total Environ., 2014, 468, 1014–1027 CrossRef PubMed.
- M. Havelcov, J. Mizera, I. Skorov and M. Pekař, J. Hazard. Mater., 2009, 161, 559–564 CrossRef PubMed.
- J. Yao, Q. Kong, Z. Qiu, L. Chen and D. Shen, Chem. Eng. J., 2019, 375, 122060 CrossRef CAS.
- A. Datta, S. Sanyal and S. Saha, Plant Soil, 2001, 235, 115–125 CrossRef CAS.
- L. Weng, E. J. Temminghoff, S. Lofts, E. Tipping and W. H. Van Riemsdijk, Environ. Sci. Technol., 2002, 36, 4804–4810 CrossRef CAS PubMed.
- R. Jain, D. Dominic, N. Jordan, E. R. Rene, S. Weiss, E. D. van Hullebusch, R. Hübner and P. N. Lens, Chem. Eng. J., 2016, 284, 917–925 CrossRef CAS.
- L. Wei, J. Li, M. Xue, S. Wang, Q. Li, K. Qin, J. Jiang, J. Ding and Q. Zhao, Bioresour. Technol., 2019, 291, 121868 CrossRef CAS PubMed.
- R. K. Skogerboe and S. A. Wilson, Anal. Chem., 2002, 53, 228–232 CrossRef.
-
G. Sposito, Aquatic Redox Chemistry, 2011, vol. 1071, pp. 113–127 Search PubMed.
- E. A. Ghabbour, M. Shaker, A. El-Toukhy, I. M. Abid and G. Davies, Chemosphere, 2006, 63, 477–483 CrossRef CAS PubMed.
- E. A. Ghabbour, M. Shaker, A. El-Toukhy, I. M. Abid and G. Davies, Chemosphere, 2006, 64, 826–833 CrossRef CAS PubMed.
- E. A. Ghabbour, G. Davies, N. K. Ghali and M. D. Mulligan, Can. J. Soil Sci., 2001, 81, 331–336 CrossRef CAS.
- S. Zhang, Q. Du, K. Cheng, M. Antonietti and F. Yang, Chem. Eng. J., 2020, 394, 124832 CrossRef CAS.
- Q. Du, G. Li, S. Zhang, J. Song and F. Yang, J. Hazard. Mater., 2020, 383, 121170 CrossRef CAS PubMed.
- B. Gu and J. Chen, Geochim. Cosmochim. Acta, 2003, 67, 3575–3582 CrossRef CAS.
-
E. O’Loughlin, H. Ma and D. Burris, presented in part at the Proceedings of the 11th Int. Meeting of IHSS “Humic substances: nature's most versatile materials”, 2002.
- M. J. Salloum, B. Chefetz and P. G. Hatcher, Environ. Sci. Technol., 2002, 36, 1953–1958 CrossRef CAS PubMed.
- J.-D. Mao, L. Hundal, M. Thompson and K. Schmidt-Rohr, Environ. Sci. Technol., 2002, 36, 929–936 CrossRef CAS PubMed.
- P. M. Bradley, F. H. Chapelle and D. R. Lovley, Appl. Environ. Microbiol., 1998, 64, 3102–3105 CrossRef CAS PubMed.
- F. J. Cervantes, W. Dijksma, T. Duong-Dac, A. Ivanova, G. Lettinga and J. A. Field, Appl. Environ. Microbiol., 2001, 67, 4471–4478 CrossRef CAS PubMed.
- K. T. Finneran and D. R. Lovley, Environ. Sci. Technol., 2001, 35, 1785–1790 CrossRef CAS PubMed.
- P. Westerhoff, G. Aiken, G. Amy and J. Debroux, Water Res., 1999, 33, 2265–2276 CrossRef CAS.
-
V. Moulin, Use of humic substances to remediate polluted environments: from theory to practice, Springer, 2005, pp. 155–173 Search PubMed.
- G. Bidoglio, I. Grenthe, P. Qi, P. Robouch and N. Omentto, Talanta, 1991, 38, 999–1008 CrossRef CAS.
- P. R. Wittbrodt and C. D. Palmer, Environ. Sci. Technol., 1995, 29, 255–263 CrossRef CAS PubMed.
- V. Moulin, B. Amekraz, N. Barre, G. Planque, F. Mercier, P. Reiller and C. Moulin, Humic Substances: Nature's Most Versatile Materials, 2004, 275–286 CAS.
-
V. Moulin, P. Reiller, C. Dautel, G. Plancque, I. Laszak and C. Moulin, in Effects of humic substances on the migration of radionuclides: Complexation and transport of actinides, ed. G. Buckau, Institut fur Nukleare Entsorgungstechnik, 1999 Search PubMed.
- P. Reiller, V. Moulin, F. Casanova and C. Dautel, Radiochim. Acta, 2003, 91, 513–524 CrossRef CAS.
- P. Panak, R. Klenze and J. Kim, Radiochim. Acta, 1996, 74, 141–146 CrossRef CAS.
- J. K. Fredrickson, H. M. Kostandarithes, S. Li, A. E. Plymale and M. Daly, Appl. Environ. Microbiol., 2000, 66, 2006–2011 CrossRef CAS PubMed.
- M. Tahir, M. Khurshid, M. Khan, M. Abbasi and M. Kazmi, Pedosphere, 2011, 21, 124–131 CrossRef CAS.
- L. R. Bento, C. A. Melo, O. P. Ferreira, A. B. Moreira, S. Mounier, A. Piccolo, R. Spaccini and M. C. Bisinoti, Sci. Total Environ., 2020, 708, 135000 CrossRef CAS PubMed.
- X. Wang, A. Muhmood, R. Dong and S. Wu, J. Cleaner Prod., 2020, 255, 120243 CrossRef CAS.
- F. Yang, S. Zhang, Q. Fu and M. Antonietti, Land Degradation Development, 2020, 31, 884–893 CrossRef.
- F. Yang, S. Zhang, J. Song, Q. Du, G. Li, N. V. Tarakina and M. Antonietti, Angew. Chem., Int. Ed., 2019, 58, 18813–18816 CrossRef CAS PubMed.
- Q. Du, S. Zhang, M. Antonietti and F. Yang, ACS Sustainable Chem. Eng., 2020, 8, 9775–9782 CrossRef CAS.
- C. Tang, Y. Li, J. Song, M. Antoniettic and F. Yang, iScience, 2020 Search PubMed.
- Z.-Z. Zhang, J.-J. Xu, Z.-J. Shi, Y.-H. Bai, Y.-F. Cheng, H.-Y. Hu and R.-C. Jin, Bioresour. Technol., 2017, 243, 883–892 CrossRef CAS.
- P. Huang, Z. Ye, W. Xie, Q. Chen, J. Li, Z. Xu and M. Yao, Water Res., 2013, 47, 4050–4058 CrossRef CAS.
- Y. Chen, N. Senesi and M. Schnitzer, Soil Sci. Soc. Am. J., 1977, 41, 352–358 CrossRef CAS.
- M. A. Mirza, N. Ahmad, S. P. Agarwal, D. Mahmood, M. K. Anwer and Z. Iqbal, Results Pharma Sci., 2011, 1, 16–26 CrossRef CAS PubMed.
- J. Winkler and S. Ghosh, J. Diabetes Res., 2018, 2018, 5391014 Search PubMed.
- C. Yang, Q. Zeng, Y. Yang, R. Xiao, Y. Wang and H. Shi, J. Ind. Eng. Chem., 2014, 20, 1133–1139 CrossRef CAS.
|
This journal is © The Royal Society of Chemistry 2021 |