DOI:
10.1039/D0CE01507E
(Paper)
CrystEngComm, 2021,
23, 56-63
Novel silver(I) cluster-based coordination polymers as efficient luminescent thermometers†
Received
15th October 2020
, Accepted 9th November 2020
First published on 11th November 2020
Abstract
By employing a triazole–pyridine-containing ligand, two novel coordination polymers with Ag(I) clusters exhibiting different architectures have been successfully constructed. Structural analysis indicates that diverse coordination modes of the N-donor linker are responsible for the network difference for these two compounds, affording an isolated cluster for 1 and a 2D framework for 2, respectively. Compound 1 features a butterfly-shaped [Ag8(bptp)4(H2O)2] cluster wherein strong argentophilic interactions could be observed. The linkage of Ag(I) with N and I centers gives rise to a tetranuclear building unit [Ag4(bptp)3I] in 2, which is further extended to a 2D network with the assistance of the N-donor linkers. The syntheses and crystal structures have been investigated. Solid-state photoluminescence emissions as well as luminescence lifetimes of 1 and 2 have also been studied. Moreover, temperature-dependent luminescence tests show that an excellent linear relationship between temperature and emission intensity in the ranges of 77–298 K and 77–200 K could be observed for compounds 1 and 2, respectively. The wide temperature sensing range especially for 1 implies that they could act as promising ratiometric fluorescence molecular thermometers.
Introduction
Metal–organic coordination polymers (CPs), stitched by metal centers or clusters and organic linkers through coordination bonds, not only possess fascinating ordered one-, two- or three-dimensional architectures but also could be equipped with promising properties and multiple functions in the domains of gas adsorption, photoluminescence, separation, chemical sensing, catalysis, and so on.1–3 To construct these inorganic–organic hybrid materials, the hydro/solvothermal method has been widely employed and afforded numerous CPs with various frameworks.4–6 However, the fabrication process of CPs under solvothermal conditions is affected by many factors, such as metal ions, organic ligands, temperature, solvents, pH value and templates, which all make the structures diverse and even unexpected.7,8 Among the aforesaid influential elements, the utilization of organic ligands with diverse types, shapes, sizes, geometries and rigidities is essential and will continue be a trend for the generation of CPs bearing interesting topologies as well as unique physical/chemical characteristics.9,10 N-Containing organic molecules exemplified by pyridine, imidazole, triazole and their derivatives are representative in terms of various ligands. Benefiting from their abundant coordination fashions as well as the strong coordination abilities of N-donor linkers, the corresponding CP products are usually constructed diversely accompanied with a high thermostability and excellent properties.11–13 For instance, the widely-known zeolitic imidazolate framework (ZIF) series, such as ZIF-8 and ZIF-67, are fabricated from the linkage of Zn2+/Co2+ and 2-methylimidazole, showing beautiful cage-based architectures and outstanding chemical resistance, and have been extensively employed in various fields, including sorption, separation, catalysis, and so forth.14–16 In addition, metal azolate frameworks (MAFs) have aroused huge interest during the past several years owing to the coordinative contribution of aromatic N-heterocycles.17–19 Moreover, robust CP materials constructed with triazole–pyrazine, triazole–pyridine, and other ligands have also been continuously reported recently coupled with their distinct physical properties.20–24
On the other hand, photo-functional transition metal-based coordination complexes are becoming a hot area of research among crystalline materials by virtue of their potential applications as functional solids in light emitting areas, chemical sensing and biomedical imaging.25–27 It is widely acknowledged that luminescent CPs fabricated with d10 transition metals, especially Ag(I), have attracted great attention and become an important branch of optical compounds owing to the absence of d–d nonradiative transitions.28,29 Adding to its extensive availability, rich resource and non-toxicity, Ag(I) complexes could be competent to perform as promising substitutes for other luminophores. Previous literature studies indicate that a Ag(I) ion with a d10 closed-shell electronic configuration exhibits multiple coordination numbers and geometries when connecting to organic linkers, which is regarded as vital in the generation of original structural motifs.30,31 Besides that, Ag(I), serving as a “soft” metal center, has a high affinity for “soft” bases such as N donors, which provides a great possibility for the introduction of N-containing ligands to luminescent CPs.32,33 For example, pyrazine and pyrazolate-derivative ligands were employed to construct silver-based solids displaying bright blue photoluminescence at room temperature.34 Moreover, the existence of intermetallic interactions which can influence the charge transfer in Ag-cluster-based CPs may result in functional materials with multicolor emissions, and enhancement of photophysical properties and sensitivity to outside environmental conditions such as temperature. Luminescent molecular thermometers, as a novel type of thermometer, offer a promising platform in temperature detection owing to their unique advantages in comparison with traditional thermometers including quick response, high sensitivity, being noninvasive and universality in strong magnetic or electric fields. At present, numerous ratiometric luminescent CP thermometers based on lanthanide compounds have been prepared. For example, by employing a lanthanide codoping strategy, Qian and coworkers designed a Tb3+/Eu3+ mixed Ln–organic framework featuring an excellent linear response to temperature ranging from 25 to 125 K with a high sensitivity.35 Ln-phosphonate-based CPs were prepared by Ananias et al., which could serve as ratiometric luminescent thermometers operative at close to ambient temperature.36 By contrast, only a few transition metal CPs especially d10-metal CPs as intensity-based luminescent thermometers have been reported. The distinct luminescence properties of these complexes urge us to explore and develop novel luminescent d10-metal-based CP thermometers.
Considering those mentioned above, we are exploring the possibility for the self-assembly of novel Ag(I) cluster-based CPs based on N-donor ligands to investigate their interior structures as well as photoluminescence behaviors. As a result, a triazole–pyridine-containing molecule was selected and employed to connect with Ag centers affording two original CPs with different architectures, namely [Ag4(bptp)2(H2O)]·3.5H2O(1) and [Ag3(bptp)I] (2) (H2bptp = 2,6-bis[5-(2-pyridinyl)-1H-triazol-3-yl]pyridine). Their syntheses and structural characterization have been conducted. Moreover, luminescence thermochromic phenomena of compounds 1 and 2 were studied in detail.
Experimental
Chemicals and characterization techniques
All solids and solvents were bought directly without further purification. H2bptp was purchased from Jinan Henghua Sci. & Tec. Co. Ltd. A Philips X'Pert-MPD diffractometer was employed to conduct powder X-ray diffraction (PXRD). To perform elemental analyses for the C, H and N content, an Elemental Vario EL III instrument was used. Thermogravimetric (TG) tests were performed by using a NETZSCH STA 449 F5 instrument. With a FLS920 fluorescence spectrophotometer, we have investigated the luminescence spectra.
Synthesis of [Ag4(bptp)2(H2O)]·3.5H2O (1)
16.9 mg of AgNO3 (0.1 mmol) and 5 mg of H2bptp (0.014 mmol) were treated in 3 mL CH3CN–NH3·H2O (v
:
v = 2
:
1) mixed solvent. After being sonicated for 15 min, the contents were transferred into a 20 mL glass vial. The mixture was kept at 70 °C for 24 h, giving colorless hexagonal prism-like crystals (51% yield based on H2bptp). Elemental analysis calcd (%) for C38H31Ag4N18O4.5: C 36.71, H 2.51, N 20.28; found: C 36.23, H 2.33, N 20.66.
Synthesis of [Ag3(bptp)I] (2)
16.9 mg of AgNO3 (0.1 mmol) and 18 mg of H2bptp (0.05 mmol) were mixed in 4 mL CH3CN, 1 mL NH3·H2O, and 0.1 mL of hydroiodic acid (45 wt%). The mixture was sealed in a Teflon-lined autoclave and heated at 145 °C for 6 days. Colorless strip-shaped crystals could be obtained after temperature cooling (43% yield). Elemental analysis calcd (%) for C19H11Ag3IN9: C 27.97, H 1.36, N 15.45; found: C 28.11, H 1.32, N 15.09.
Comparison of the preparation for 1 and 2
In the reaction process, the molar ratio of the metal salt and organic ligand was different for the construction of 1 and 2. However, an increasing amount of H2bptp didn't give rise to a new structure. Thus, the reactant ratio may not be responsible for the structural difference of the two compounds. For preparing compound 2, hydroiodic acid was essential and played a vital role in the fabrication of the corresponding products. No crystals were formed without the addition of HI. Besides that, reaction temperature was a key factor that affected the final architectures of 1 and 2. If the temperature was altered, X-ray quality crystals could not be harvested.
X-ray crystallographic study
Crystal data were collected on a SuperNova, Dual, Cu at home/near, Atlas diffractometer and a Rigaku XtaLAB mini CCD diffractometer (Mo Kα) for 1 and 2, respectively. The structures have been resolved and refined by using SHELX-2014. All atoms but hydrogen have been anisotropically refined. Hydrogen atoms linking with carbon were geometrically generated. Crystallographic data for compounds 1 and 2 are listed in Table 1. Some bond distances and angles are provided in Table S1.†1977163 and 1977164 are the CCDC numbers for 1 and 2, respectively.
Table 1 Crystal and refinement data for compounds 1 and 2
Compounds |
1
|
2
|
R
1 = ∑||Fo| − |Fc||/∑|Fo|.
wR2 = {∑[w(Fo2 − Fc2)2]/∑w(Fo2)2}1/2.
|
Formula |
C38H31Ag4N18O4.5 |
C19H11Ag3IN9 |
M
r (g mol−1) |
1243.29 |
815.88 |
Space group |
Ibca
|
P21/c |
Crystal system |
Orthorhombic |
Monoclinic |
a (Å) |
21.8025(3) |
14.8663(11) |
b (Å) |
26.2621(4) |
7.3955(8) |
c (Å) |
29.1639(4) |
20.839(2) |
α (°) |
90 |
90 |
β (°) |
90 |
119.363(6) |
γ (°) |
90 |
90 |
V (Å3) |
16 698.6(4) |
1996.8(3) |
Z
|
16 |
4 |
F(000) |
9744 |
1528 |
D
c (g cm−3) |
1.978 |
2.714 |
μ (mm−1) |
15.399 |
4.490 |
R
int
|
0.0278 |
0.0550 |
GOF on F2 |
1.027 |
0.992 |
R
1,bwR2[I > 2σ(I)] |
0.0301, 0.0769 |
0.0513, 0.1049 |
Results and discussion
Crystal structure of [Ag4(bptp)2(H2O)]·3.5H2O (1)
Compound 1 belongs to an orthorhombic crystal system with the centrosymmetric Ibca space group. There are four silver ions, two deprotonated H2bptp molecules, and one coordinated and three and a half solvent water molecules in its asymmetric unit. As shown in Fig. 1a, the four Ag(I) ions exhibit two coordination numbers, giving rise to different coordination manners, i.e., tri-coordination and tetra-coordination. Both Ag(1) and Ag(4) adopt distorted tetrahedral geometries connecting to four coordinated bptp2− molecules with Ag–N bond distances ranging from 2.130 to 2.631 Å. Meanwhile, for the Ag(2) and Ag(3) centers, they are three-coordinated and display distorted T-shaped geometries. As observed in this structure, the bptp2−linkers are somewhat twisted with dihedral angles between the central pyridine ring and the nearest triazole ring in the same ligand being 13.4–27.0° and 11.9–27.4° (Scheme 1). For the completely deprotonated H2bptp ligand, three pyridine rings and triazole groups take part in the coordination in mode I and mode II to connect the Ag(I) centers. Both the bptp2− ligands present a V-shaped conformation when bridging the Ag centers in an end to end fashion, furnishing a neutral [Ag4(bptp)2(H2O)] unit. As depicted in Fig. 1b, these subunits are further interconnected into a butterfly-shaped [Ag8(bptp)4(H2O)2] cluster by sharing the center Ag(I) ions and Ag–N coordination bonds. The short distances of Ag1⋯Ag2 (2.911 Å), Ag2⋯Ag2 (3.004 Å) and Ag2⋯Ag4 (2.894 Å) indicate the existence of relatively strong argentophilic interactions. As a result of the coordination effect as well as the rigidity of bptp2−, strong π⋯π interactions between the nearest pyridyl rings of different isolated octanuclear clusters (centroid–centroid distance of 3.517 Å) could be concluded (Fig. S1†), leading to a 3D supramolecular network wherein a neutral octanuclear structure is surrounded by eight neighboring crystallographic equivalent ones along the [001] direction (Fig. 1c and d). The lattice water molecules are located in the voids of the framework to stabilize the whole architecture.
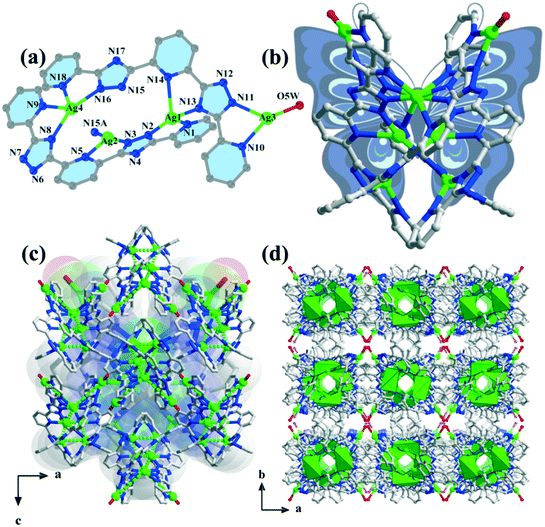 |
| Fig. 1 (a) Coordination environments of the silver ions in compound 1; (b) view of the butterfly-shaped [Ag8(bptp)4(H2O)2] cluster; ((c) and (d)) 3D supramolecule along the b axis and c axis. | |
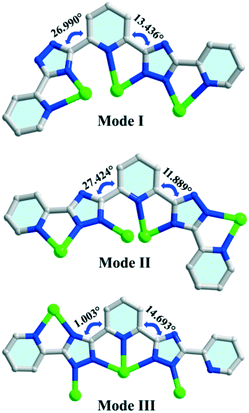 |
| Scheme 1 Diverse coordination fashions of bptp2− in 1 and 2. | |
Crystal structure of [Ag3(bptp)I] (2)
Compound 2 belongs to the monoclinic P21/c space group according to the X-ray crystallographic study. Its asymmetric unit contains three isolated Ag(I) centers, one bptp2− ligand and a coordinated I− ion (Fig. 2a). The Ag(I) ions in compound 2 have three coordination numbers (2, 3 and 5), resulting in diverse coordination geometries. Ag(1) is coordinated linearly by two N atoms with a N2–Ag1–N7A angle of 170.1°. Ag(2) ion adopts a five-coordinated mode with a slightly distorted trigonal bipyramidal environment, and is completed by three nitrogen atoms of one bptp2− ligand (Ag2–N3 = 2.475 Å, Ag2–N5 = 2.465 Å, and Ag2–N6 = 2.411 Å) and two I− ions, with the angles around it falling in the range of 66.4° to 132.8°. The Ag(3) cation is three-connected and displays an irregular Y-shaped geometry with the vertexes being occupied by two nitrogen centers from the bptp2− ligand (N8 and N9) and one I− ion. The H2bptp ligand in 2 is fully deprotonated and adopts coordination fashion III to link with four silver ions via seven nitrogen atoms from two pyridine groups and triazole rings. Meanwhile, the neighboring Ag(I) ions are bridged/chelated by three bptp2− molecules and one μ3-I− ion, generating a tetranuclear building unit [Ag4(bptp)3I] (Fig. S2†). In order to increase the steric stabilization, two triazole groups are twisted by 1.0° and 14.7° with respect to the central pyridine ring. In this architecture, the adjacent tetranuclear units are connected by bptp2− ligands, forming a 2D inorganic–organic hybrid layer as displayed in Fig. 2b. To simplify the complicated network, topological analysis is employed on it. Both Ag(1) and Ag(3) can be simplified into linkers, while the Ag(2) and I− ions can be considered as 3-connected nodes. In addition, the bptp2− ligand which connects four metal ions can be viewed as a 4-connected node. Therefore, the structure can be rationalized to a 3,4-connected 3-nodal topology with a Schläfli symbol of {5.6.7}{52·6.7.82}{52·8} (Fig. 2c). Moreover, π⋯π stacking interactions are found between terminal pyridine rings of neighboring layers with centroid to centroid distances of 3.667 Å–3.792 Å (Fig. S3†), giving rise to a final 3D supramolecular network (Fig. 2d).
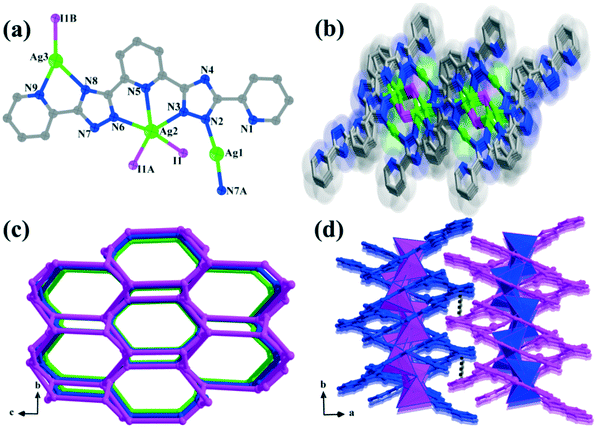 |
| Fig. 2 (a) Coordination environments of the silver ions in compound 2; (b) 2D layer of 2 along the [010] structure; (c) view of the 2D topological framework; (d) 3D supramolecule of 2 with π⋯π interactions indicated by the dashed lines. | |
Comparison of the structures of 1 and 2
In compounds 1 and 2, H2bptp with three pyridine rings and two triazole groups was employed to assist with the assembly of Ag(I)–CPs bearing variable architectures. The crystallographic results clearly show the diverse architectures of 1 and 2, from an isolated cluster to a 2D network, in which the metal centers are two-, three-, four-, and five-coordinated and the pyridine–triazole ligands display various coordination modes. As shown in Scheme 1, the N-donor ligand presents three types of coordination fashions in a V-shaped geometry bonding with neighboring silver clusters, which plays a significant role in the assembly process. Mode I could be observed in compound 1, in which the bptp2− ligand coordinates to three silver(I) cations in tridentate mode. Differently, the another bptp2− ligand in compound 1 exhibits mode II, where three pyridyl N atoms and four triazole N atoms coordinate to four Ag(I) centers. Coordination mode III is formed in compound 2, in which the organic linker coordinates to four distinct Ag(I) centers with two pyridyl N atoms and five bridging triazole N atoms, resulting in an infinite 2D layered motif. In brief, the diverse coordination modes of the N-donor linker are the main reason for the network difference for these two compounds. Compared with other reported Ag-cluster based CPs which are mainly assembled from pyridine and its derivatives,37–39 compounds 1 and 2 in this work are fabricated from the linkage of Ag(I) centers and a polydentate triazole–pyridine-containing molecule. The abundant coordination sites together with the diverse linking fashions of the ligand have resulted in two original CPs with unique structural characteristics.
PXRD and TG analyses
To check the phase purities of solids 1 and 2, PXRD patterns were collected. As depicted in Fig. S4 and S5,† the curves for the as-synthesized samples match well with the simulated ones from the data of compounds 1 and 2, indicating their purity after the synthesis process.40,41 TG tests have been carried out from 30 to 800 °C under an inert gas atmosphere. As displayed on the TG curve of 1, a 5.8% weight loss before 100 °C could be observed, which corresponds to the removal of lattice H2O molecules (calcd 5.1%). Further temperature increase is accompanied with the release of coordinated water molecules. The desolvated framework can maintain its host stability up to 460 °C, followed by the decomposition of the skeleton.42–44 There is no guest molecule in compound 2, so no obvious weight loss is observed before 440 °C (Fig. S6†).
Luminescence properties
Luminescent CPs have attracted much research interest recently owing to their potential functions as light-emitting diodes.45–47 Generally speaking, some silver(I)-based compounds display abundant structural diversity combined with intense luminescence even at ambient temperature. Moreover, their emissive behaviours could be readily modulated by the change of structure and environment.48,49 Encouraged by these considerations, we are urged to investigate the photoluminescence properties of compounds 1 and 2 in their solid state. Additionally, to track their thermal response, temperature-dependent emission spectra have been collected between 298 K and 77 K by dipping these solids into liquid nitrogen. Upon excitation at 365 nm, compound 1 displays yellow emission at room temperature, and emits a wide luminescence band with the maximum peak being located at about 577 nm. The CIE chromaticity coordinates are calculated to be (0.42, 0.45) as shown in Fig. 3a. When the environment temperature decreases from 298 K to the temperature of liquid nitrogen, a slightly red-shifted emission band accompanied with a gradually increased intensity can be observed for solid 1. Meanwhile, for compound 2, it can be seen from Fig. 3c that a blue-shift phenomenon occurs with a stepwise decrease of temperature. The emission peak at 580 nm at room temperature shifts gradually to a shorter wavelength with temperature cooling and finally reaches 550 nm at 77 K. The corresponding changes of the luminescence color are found to be yellow to green-yellow, as depicted in the CIE chromaticity diagram at variable temperatures. The different luminescence responses to temperature of 1 and 2 may be ascribed to their detailed structural differences, like the configuration of the organic ligand, Ag–Ag interactions and packing modes. Moreover, an excellent linear relationship between emission intensity and temperature in the ranges of 77–300 K and 77–200 K for 1 and 2 could be fitted as equations: y =2.50 × 105 − 827x (R2 = 0.9905) and y = 3.25 × 105 − 1327x (R2 = 0.9903). The temperature sensing ranges (region of linear relationship) of the compounds, especially for 1, are wider than those of the majority of reported luminescent thermometers (Table S2†), indicating that these materials have potential applications in intensity-dependent luminescent thermometers (Fig. 3b and d).50–52
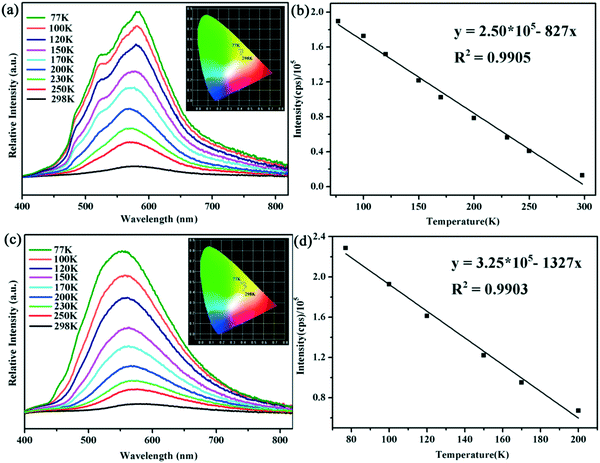 |
| Fig. 3 Luminescence spectra of (a) 1 and (c) 2 from 298 K to 77 K; emission intensity and fitted curves of (b) 1 from 77 to 300 K and (d) 2 from 77 to 200 K. | |
To further investigate and analyze the emission nature of compounds 1 and 2, photoluminescence spectroscopy for the free H2bptp ligand has been carried out. Upon irradiation at 365 nm and 298 K, the organic molecule shows a blue emission curve with the maximum wavelength residing at 450 nm corresponding to the π*–π transitions (Fig. S7†). According to previous reports on Ag(I) compounds, the emission behaviors of 1 and 2 at different temperatures could be tentatively assigned to a triplet metal-to-ligand charge-transfer (MLCT) excited state mixed with the Ag⋯Ag [4d → 5s] triplet-cluster-centered (3CC) excited state.53–56 Furthermore, research for time-resolved luminescence has also been conducted by monitoring the most intense emission for the corresponding samples at 298 K and 77 K. The decay curves could be well fitted as shown in Fig. 4 with detailed data being listed in Table 2. At 298 K, the luminescence lifetimes of solids 1 and 2 are determined to be 2.56 and 3.34 ms, respectively. Upon cooling to 77 K with liquid nitrogen, the emission lifetimes become longer (τ = 19.88 and 15.49 ms for 1 and 2), suggesting a spin-forbidden triplet parentage. From the results above, the lifetimes of 1 and 2 at 298 K and 77 K are in the range of milliseconds, indicating a phosphorescence behavior.57,58
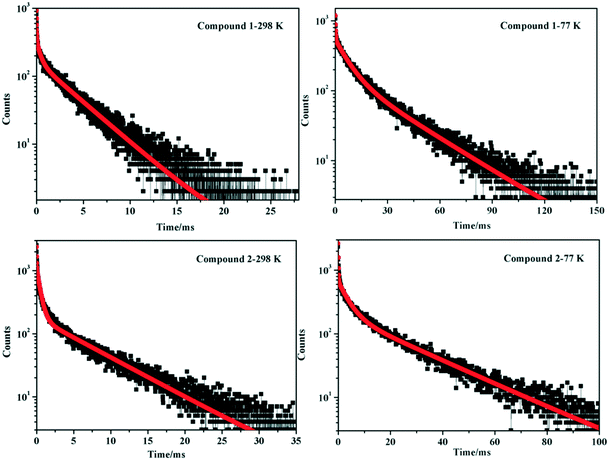 |
| Fig. 4 Luminescence decay curves of solids 1 and 2. | |
Table 2 Photoluminescence data of 1 and 2 at 298 K and 77 K
|
T (K) |
λ
ex (nm) |
λ
em (nm) |
τ (ms) |
Triexponential decays.
|
Compound 1 |
298 |
365 |
577 |
0.02257 (21.44%a) |
0.4124 (9.39%a) |
3.632 (69.17%a) |
77 |
365 |
582 |
0.1619 (4.48%a) |
7.041 (32.71%a) |
27.97 (62.81%a) |
Compound 2 |
298 |
365 |
580 |
0.03258 (31.92%a) |
0.4743 (21.27%a) |
6.886 (46.81%a) |
77 |
365 |
550 |
0.1306 (14.90%a) |
3.700 (23.06%a) |
23.56 (62.03%a) |
Conclusions
In summary, the combination of Ag(I) with a polydentate N-containing organic linker affords two original coordination polymers featuring strong argentophilic interactions in this work. The structural difference between the two compounds can be ascribed to the N-donor ligand which adopts three different kinds of coordination fashions. An excellent linear relationship between temperature and emission intensity in a wide temperature range for the two compounds could be observed through conducting temperature-dependent luminescence experiments. Apart from lanthanide-based complexes, the title d10 transition metal compounds could also be competent as promising candidates for temperature identification, which provides a new strategy for the development of novel luminescent thermometers.
Conflicts of interest
There are no conflicts to declare.
Acknowledgements
We are thankful for the funding support from the National Natural Science Foundation of China (22071126, 22075156), and the Key Research and Development Project of Shandong Province (2019GGX102006).
Notes and references
- Z. Chang, D. H. Yang, J. Xu, T. L. Hu and X. H. Bu, Adv. Mater., 2015, 27, 5432–5441 CrossRef.
- M. J. Kalmutzki, C. S. Diercks and O. M. Yaghi, Adv. Mater., 2018, 30, 1704304 CrossRef.
- Y. J. Ma, J. X. Hu, S. D. Han, J. Pan, J. H. Li and G. M. Wang, J. Am. Chem. Soc., 2020, 142, 2682–2689 CrossRef.
- J. H. Qin, Y. D. Huang, M. Y. Shi, H. R. Wang, M. L. Han, X. G. Yang, F. F. Li and L. F. Ma, RSC Adv., 2020, 10, 1439–1446 RSC.
- X. Gao, Y. Du, S. Li, J. Zhou, X. Feng, X. Jin and B. Wang, ACS Appl. Mater. Interfaces, 2020, 12, 844–850 CrossRef.
- J. Kobayashi, T. Misawa, C. Umeda, T. Isono, S. Ono, H. Naruke, Y. Okamura, S. Koguchi, M. Higuchi, Y. Nagase and T. Ito, CrystEngComm, 2019, 21, 629–636 RSC.
- S. K. Elsaidi, M. H. Mohamed, D. Banerjee and P. K. Thallapally, Coord. Chem. Rev., 2018, 358, 125–152 CrossRef.
- J. G. Jia and L. M. Zheng, Coord. Chem. Rev., 2020, 403, 213083 CrossRef.
- N. Li, R. Feng, J. Zhu, Z. Chang and X. H. Bu, Coord. Chem. Rev., 2018, 375, 558–586 CrossRef.
- A. R. Geisse, C. M. Ngule and D. T. Genna, Chem. Commun., 2020, 56, 237–240 RSC.
- A. V. Desai, S. Sharma, A. Roy and S. K. Ghosh, Cryst. Growth Des., 2019, 19, 7046–7054 CrossRef CAS.
- Y. J. Ma, J. X. Hu, S. D. Han, J. Pan, J. H. Li and G. M. Wang, Chem. Commun., 2019, 55, 5631–5634 RSC.
- C. Pettinari, A. Tăbăcaru and S. Galli, Coord. Chem. Rev., 2016, 307, 1–31 CrossRef CAS.
- Y. Pan, K. Sun, S. Liu, X. Cao, K. Wu, W. C. Cheong, Z. Chen, Y. Wang, Y. Li, Y. Liu, D. Wang, Q. Peng, C. Chen and Y. Li, J. Am. Chem. Soc., 2018, 140, 2610–2618 CrossRef CAS.
- Z. Zhang, Y. Huang, X. Liu, C. Chen, Z. Xu and P. Liu, Carbon, 2020, 157, 244–254 CrossRef CAS.
- Y. Yang, Z. Guo, W. Huang, S. Zhang, J. Huang, H. Yang, Y. Zhou, W. Xu and S. Gu, Appl. Surf. Sci., 2020, 503, 144079 CrossRef.
- S. Y. Liu, J. P. Zhang and X. M. Chen, Cryst. Growth Des., 2017, 17, 1441–1449 CrossRef CAS.
- B. N. Bhadra, N. A. Khan and S. H. Jhung, J. Mater. Chem. A, 2019, 7, 17823–17833 RSC.
- S. Wang, T. Wang, P. Liu, Y. Shi, G. Liu and J. Li, Mater. Res. Bull., 2017, 88, 62–68 CrossRef CAS.
- H. Benaissa, M. Wolff, K. Robeyns, G. Knör, K. Van Hecke, N. Campagnol, J. Fransaer and Y. Garcia, Cryst. Growth Des., 2019, 19, 5292–5307 CrossRef CAS.
- R. G. Miller and S. Brooker, Chem. Sci., 2016, 7, 2501–2505 RSC.
- R. Das, S. S. Dhankhar and C. M. Nagaraja, Inorg. Chem. Front., 2020, 7, 72–81 RSC.
- L. Shao, Y. Sang, J. Huang and Y. N. Liu, Chem. Eng. J., 2018, 353, 1–14 CrossRef CAS.
- G. N. Newton, T. Onuki, T. Shiga, M. Noguchi, T. Matsumoto, J. S. Mathieson, M. Nihei, M. Nakano, L. Cronin and H. Oshio, Angew. Chem., Int. Ed., 2011, 50, 4844–4848 CrossRef CAS.
- J. H. Qin, Y. D. Huang, Y. Zhao, X. G. Yang, F. F. Li, C. Wang and L. F. Ma, Inorg. Chem., 2019, 58, 15013–15016 CrossRef CAS.
- Y. Zhang, S. Yuan, G. Day, X. Wang, X. Yang and H. C. Zhou, Coord. Chem. Rev., 2018, 354, 28–45 CrossRef CAS.
- T. Rasheed and F. Nabeel, Coord. Chem. Rev., 2019, 401, 213065 CrossRef CAS.
- W. J. Ji, G. F. Liu, B. Q. Wang, W. B. Lu and Q. G. Zhai, CrystEngComm, 2020, 22, 4710–4715 RSC.
- Y. Yuan, Y. W. Xiao, X. S. Yan, S. X. Wu, H. Luo, J. B. Lin, Z. Li and Y. B. Jiang, Chem. Commun., 2019, 55, 12849–12852 RSC.
- R. W. Huang, Y. S. Wei, X. Y. Dong, X. H. Wu, C. X. Du, S. Q. Zang and T. C. W. Mak, Nat. Chem., 2017, 9, 689–697 CrossRef CAS.
- Z. Wang, J. W. Liu, H. F. Su, Q. Q. Zhao, M. Kurmoo, X. P. Wang, C. H. Tung, D. Sun and L. S. Zheng, J. Am. Chem. Soc., 2019, 141, 17884–17890 CrossRef CAS.
- M. Y. Dou, X. Q. Huang and G. Y. Yang, CrystEngComm, 2020, 22, 2642–2648 RSC.
- Y. Domoto, M. Abe, T. Kikuchi and M. Fujita, Angew. Chem., 2020, 59, 3450–3454 CrossRef CAS.
- R. M. Almotawa, G. Aljomaih, D. V. Trujillo, V. N. Nesterov and M. A. Rawashdeh-Omary, Inorg. Chem., 2018, 57, 9962–9976 CrossRef CAS.
- D. Zhao, D. Yue, L. Zhang, K. Jiang and G. Qian, Inorg. Chem., 2018, 57, 12596–12602 CrossRef CAS.
- D. Ananias, A. D. G. Firmino, R. F. Mendes, F. A. Almeida Paz, M. Nolasco, L. D. Carlos and J. Rocha, Chem. Mater., 2017, 29, 9547–9554 CrossRef CAS.
- G. Li, H. Ye, F. Zhu, Y. Geng, J. Fan, J. Ma, C. Adachi and Y. B. Dong, Chin. Chem. Lett., 2019, 30, 1931–1934 CrossRef CAS.
- J. Wang, Y. L. Li, Z. Y. Wang and S. Q. Zang, CrystEngComm, 2019, 21, 2264–2267 RSC.
- X. Y. Dong, Y. Si, J. S. Yang, C. Zhang, Z. Han, P. Luo, Z. Y. Wang, S. Q. Zang and T. C. W. Mak, Nat. Commun., 2020, 11, 3678 CrossRef CAS.
- K. Yorseng, S. Siengchin, B. Ashok and A. Varada Rajulu, J. Bioresour. Bioprod., 2020, 5, 101–107 CrossRef CAS.
- B. Ashok, N. Hariram, S. Siengchin and A. Varada Rajulu, J. Bioresour. Bioprod., 2020, 5, 180–185 CrossRef.
- S. Jiang, J. Y. Cheong, J. S. Nam, I.-D. Kim, S. Agarwal and A. Greiner, ACS Appl. Mater. Interfaces, 2020, 12, 19006–19014 CrossRef CAS.
- D. Wang, J. Yu, G. Duan, K. Liu and H. Hou, J. Mater. Sci., 2020, 55, 5667–5679 CrossRef CAS.
- Q. Tang, L. Fang and W. Guo, J. Bioresour. Bioprod., 2019, 4, 51–59 CAS.
- X. Zhao, X. Song, Y. Li, Z. Chang and L. Chen, ACS Appl. Mater. Interfaces, 2018, 10, 5633–5640 CrossRef CAS.
- H. Wang, W. P. Lustig and J. Li, Chem. Soc. Rev., 2018, 47, 4729–4756 RSC.
- X. T. Liu, K. Wang, Z. Chang, Y. H. Zhang, J. Xu, Y. S. Zhao and X. H. Bu, Angew. Chem., Int. Ed., 2019, 58, 13890–13896 CrossRef CAS.
- Y. H. Feng, X. L. Gao, J. F. Shi, K. Zhou, J. Y. Ji and Y. F. Bi, Chem. – Asian J., 2019, 14, 3279–3282 CrossRef CAS.
- L. Soria, C. Cuerva, M. Cano, J. A. Campo and C. Lodeiro, Dyes Pigm., 2018, 150, 323–334 CrossRef CAS.
- S. Q. Deng, X. J. Mo, S. L. Cai, W. G. Zhang and S. R. Zheng, Inorg. Chem., 2019, 58, 14660–14666 CrossRef CAS.
- C. Y. Yue, F. L. Liu, W. T. Deng, J. Tao and M. C. Hong, Cryst. Growth Des., 2017, 18, 22–26 CrossRef.
- R. C. Zhang, J. J. Wang, J. C. Zhang, M. Q. Wang, M. Sun, F. Ding, D. J. Zhang and Y. L. An, Inorg. Chem., 2016, 55, 7556–7563 CrossRef CAS.
- Z. H. Yan, X. Y. Li, L. W. Liu, S. Q. Yu, X. P. Wang and D. Sun, Inorg. Chem., 2016, 55, 1096–1101 CrossRef CAS.
- Y. Tian, Z. Y. Wang, S. Q. Zang, D. Li and T. C. W. Mak, Dalton Trans., 2019, 48, 2275–2279 RSC.
- R. W. Huang, X. Y. Dong, B. J. Yan, X. S. Du, D. H. Wei, S. Q. Zang and T. C. W. Mak, Angew. Chem., Int. Ed., 2018, 57, 8560–8566 CrossRef CAS.
- S. Z. Zhan, F. Ding, X. W. Liu, G. H. Zhang, J. Zheng and D. Li, Inorg. Chem., 2019, 58, 12516–12520 CrossRef CAS.
- L. Yang, J. Zhou and L. Fu, Dyes Pigm., 2020, 174, 108039 CrossRef CAS.
- H. Xiao, J. Zhou, X. Liu, H. Xiao, R. A. S. Ferreira, L. D. Carlos and L. Fu, Dalton Trans., 2018, 47, 3253–3257 RSC.
Footnote |
† Electronic supplementary information (ESI) available: Selected bond distances and angles for compounds 1 and 2, additional figures for the structures of 1 and 2, PXRD and TGA curves for compounds 1 and 2, and emission spectra of the H2bptp ligand. CCDC numbers are 1977163 and 1977164 for compounds 1 and 2, respectively. For ESI and crystallographic data in CIF or other electronic format see DOI: 10.1039/d0ce01507e |
|
This journal is © The Royal Society of Chemistry 2021 |
Click here to see how this site uses Cookies. View our privacy policy here.