DOI:
10.1039/D0CE01458C
(Paper)
CrystEngComm, 2021,
23, 40-46
Unusual porous crystals via catenation of 1D ladders: unprecedented mixture effects on the adsorption of xylene isomers in a SCSC mode†
Received
5th October 2020
, Accepted 20th October 2020
First published on 21st October 2020
Introduction
Reversible adsorption/desorption of guest molecules into stable porous hosts is a key process in determining recognition, separation, extraction, energy transfer, and hydrophobicity-modification.1–13 Such task-specific porous framework materials offer intriguing possibilities for modulation of nestled guest-conformations, guest-organization, and unusual transition states in the single-crystal-to-single-crystal (SCSC) mode.14–23 Procedural research on effective separation of practical isomers is one of the hottest topics in the field of porous crystalline materials.24–28 To this end, various porous metal–organic frameworks have been designed and constructed for molecular sieving functions as well as specific adsorption affinity.29–34 The pore nature and dynamics of metal–organic-framework crystalline materials are factors essential to selective adsorption/desorption of useful guests. Separation of p-xylene (p-X; diameter = 0.58 nm) via natural zeolites and their analogues is well known,15,18–20,35–38 but the similar kinetic diameters of m-xylene (m-X = 0.64 nm) and o-xylene (o-X = 0.65 nm) isomers39 make their separation particularly tough. Thus, preferential adsorption of m-X over o-X (or vice versa) by means of porous metal–organic frameworks is important for its great practical utility. Separation of isomers via such efficient host–guest recognition can be strongly dependent on momentous factors such as temperature and porous nature.40,41 However, systematic research on mixture effects pertinent to separation of o-X, m-X, and p-X isomers remains both lacking and coveted. This paper provides both (1) construction of unique porous coordination network crystals formed via catenation of one-dimensional (1D) zigzag ladder-type coordination polymers and (2) unprecedented mixture and temperature effects for the adsorption and separation of o-X, m-X, and p-X via SCSC guest exchanges without any destruction of a porous, reproducible and tolerant skeleton.
Experimental
Materials and measurement
AgClO4 and 1,3,5-tris(bromomethyl)benzene were purchased from Aldrich, and 4-hydroxybenzaldehyde and isonicotinoyl chloride hydrochloride were obtained from Alfa. (((Benzene-1,3,5-triyltris(methylene))tris(oxy))tris(benzene-1,4-diyl))tris(methylene)triisonicotinate (L) was prepared according to the literature.40 The 1H spectra were recorded on a Varian Mercury Plus at 400 MHz; and the chemical shifts were measured relative to tetramethylsilane (TMS) as the internal standard in δ values (ppm). The infrared spectra were obtained on a Nicolet 380 FT-IR spectrophotometer with samples prepared as KBr pellets. Thermal analyses were carried out under a dinitrogen atmosphere at a scan rate of 10 °C min−1 using a Labsys TGA-DSC 1600.
[(2dioxane)@Ag(ClO4)L]
A methanol solution (5 mL) of AgClO4 (2.59 mg, 0.0125 mmol) was slowly diffused into a 1,4-dioxane solution (5 mL) of L (10.02 mg, 0.0125 mmol). Colourless crystals formed at the interface after 5 days. Anal. calcd. for C56H55AgClN3O17: C, 62.42; H, 5.14; N, 3.90%. Found: C, 61.95; H, 5.10; N, 3.83%. IR (KBr, cm−1): 1726 (s), 1610 (s), 1587 (w), 1562 (w), 1514 (s), 1454 (m), 1419 (m), 1378 (m), 1330 (m), 1282 (s), 1240 (s), 1219 (s), 1178 (m), 1120 (s), 1081 (w), 1058 (m), 1014 (w), 1001 (w), 922 (w), 838 (s), 764 (m), 704 (m), 685 (w), 613 (w), 582 (w), 557 (s), 507 (w), 461 (w), 405 (w). 1H NMR (dissociated in Me2SO-d6, δ): 8.79 (d, J = 5.87 Hz, 6H), 7.83 (d, J = 5.87 Hz, 6H), 7.50 (s, 3H), 7.42 (d, J = 8.61 Hz, 6H), 7.04 (d, J = 8.61 Hz, 6H), 5.31 (s, 6H), 5.16 (s, 6H), 3.57 (s, 16H).
[(1.5p-X)@Ag(ClO4)L] via adsorption of p-xylene in a SCSC mode
Single crystals of [(2dioxane)@Ag(ClO4)L] were left in p-X media at room temperature for 22 h, resulting in the transformation of single crystals of [(2dioxane)@Ag(ClO4)L] into [(1.5p-X)@Ag(ClO4)L] crystals. Anal. calcd. for C60H54AgClN3O13: C, 61.68; H, 4.66; N, 3.60%. Found: C, 62.24; H, 4.56; N, 3.51%. IR (KBr, cm−1): 1726 (s), 1610 (s), 1587 (w), 1562 (w), 1514 (s), 1454 (m), 1419 (m), 1378 (m), 1330 (m), 1282 (s), 1240 (s), 1219 (s), 1178 (m), 1120 (s), 1081 (w), 1058 (m), 1014 (w), 1001 (w), 922 (w), 838 (s), 764 (m), 704 (m), 685 (w), 613 (w), 582 (w), 557 (s), 507 (w), 461 (w), 405 (w). 1H NMR (dissociated in Me2SO-d6, δ): 8.79 (d, J = 5.28 Hz, 6H), 7.83 (d, J = 5.87 Hz, 6H), 7.51 (s, 3H), 7.43 (d, J = 8.8 Hz, 6H), 7.04 (d, J = 8.61 Hz, 10H), 5.32 (s, 6H), 5.16 (s, 6H), 2.25 (s, 6H).
[(1.5m-X)@Ag(ClO4)L] via adsorption of m-xylene in a SCSC mode
Single crystals of [(2dioxane)@Ag(ClO4)L] were left in m-X media at room temperature for 2 days, resulting in the transformation of single crystals of [(2dioxane)@Ag(ClO4)L] into [(1.5m-X)@Ag(ClO4)L]. Anal. calcd. for C60H54AgClN3O13: C, 61.68; H, 4.66; N, 3.60%. Found: C, 62.03; H, 4.69; N, 3.59%. IR (KBr, cm−1): 1726 (s), 1610 (s), 1587 (w), 1562 (w), 1514 (s), 1454 (m), 1419 (m), 1378 (m), 1330 (m), 1282 (s), 1240 (s), 1219 (s), 1178 (m), 1120 (s), 1081 (w), 1058 (m), 1014 (w), 1001 (w), 922 (w), 838 (s), 764 (m), 704 (m), 685 (w), 613 (w), 582 (w), 557 (s), 507 (w), 461 (w), 405 (w). 1H NMR (dissociated in Me2SO-d6, δ): 8.79 (d, J = 5.28 Hz, 6H), 7.83 (d, J = 5.28 Hz, 6H), 7.51 (s, 3H), 7.43 (d, J = 8.22 Hz, 6H), 7.13 (m, J = 15.26 Hz, 2H), 7.04 (d, J = 8.22 Hz, 6H), 6.97 (d, J = 12.91 Hz, 2H), 5.32 (s, 6H), 5.16 (s, 6H), 2.26 (s, 6H).
[(1.5o-X)@Ag(ClO4)L] via adsorption of o-xylene in a SCSC mode
Single crystals of [(2dioxane)@Ag(ClO4)L] were left in o-xylene media at room temperature for 6 days, resulting in the transformation of single crystals of [(2dioxane)@Ag(ClO4)L] into [(1.5o-X)@Ag(ClO4)L] suitable for single crystal X-ray crystallography. Anal. calcd. for C60H54AgClN3O13: C, 61.68; H, 4.66; N, 3.60%. Found: C, 62.08; H, 4.60; N, 3.54%. IR (KBr, cm−1): 1726 (s), 1610 (s), 1587 (w), 1562 (w), 1514 (s), 1454 (m), 1419 (m), 1378 (m), 1330 (m), 1282 (s), 1240 (s), 1219 (s), 1178 (m), 1120 (s), 1081 (w), 1058 (m), 1014 (w), 1001 (w), 922 (w), 838 (s), 764 (m), 704 (m), 685 (w), 613 (w), 582 (w), 557 (s), 507 (w), 461 (w), 405 (w). 1H NMR (dissociated in Me2SO-d6, δ): 8.79 (d, J = 5.28 Hz, 6H), 7.83 (d, J = 5.28 Hz, 6H), 7.51 (s, 3H), 7.43 (d, J = 8.22 Hz, 6H), 7.13 (m, J = 15.26 Hz, 2H), 7.04 (d, J = 8.22 Hz, 6H), 6.96 (d, J = 12.91 Hz, 2H), 5.32 (s, 6H), 5.16 (s, 6H), 2.21 (s, 6H).
Adsorption of mixture xylene isomers into the channels was also attempted in the same manner.
Crystal-structure determination
X-ray data on [(2dioxane)@Ag(ClO4)L] and [(1.5o-X)@Ag(ClO4)L] were measured at 120 K with synchrotron radiation (λ = 0.7000 Å) on a Rayonix MX225HS detector at 2D SMC with a silicon (111) double-crystal monochromator (DCM) at the Pohang Accelerator Laboratory, Korea. The PAL BL2D-SMDC program42 was used for data collection (detector distance was 66 mm, omega scan; Δω = 1°, exposure time was 1 s per frame), and HKL3000sm (ver. 703r)43 was used for cell refinement, reduction, and absorption correction. The structures were solved using direct methods (SHELXS) and refined by full-matrix least squares techniques (SHELXL 2018/3).44 The non-hydrogen atoms were refined anisotropically, and the hydrogen atoms were placed in calculated positions and refined only for the isotropic thermal factors. Their crystallographic data are listed in Table 1.
Table 1 Crystallographic data
|
[(2dioxane)@Ag(ClO4)L] |
[(1.5o-X)@Ag(ClO4)L] |
R
1 = ∑||Fo| − |Fc||/∑|Fo|.
wR2 = (∑[w(Fo2 − Fc2)2]/∑[w(Fo2)2])1/2.
|
Formula |
C112H108Ag2Cl2N6O34 |
C60H54AgClN3O13 |
M
w
|
2368.68 |
1168.38 |
Cryst. sys. |
Triclinic |
Triclinic |
Space group |
P![[1 with combining macron]](https://www.rsc.org/images/entities/char_0031_0304.gif) |
P![[1 with combining macron]](https://www.rsc.org/images/entities/char_0031_0304.gif) |
a (Å) |
12.519(3) |
12.716(3) |
b (Å) |
13.668(3) |
13.798(3) |
c (Å) |
16.827(3) |
16.840(3) |
α (°) |
99.56(3) |
97.80(3) |
β (°) |
108.37(3) |
110.15(3) |
γ (°) |
95.92(3) |
98.57(3) |
V (Å3) |
2657(1) |
2687(1) |
Z
|
1 |
2 |
ρ (g cm−3) |
1.480 |
1.444 |
μ (mm−1) |
0.480 |
0.668 |
R
int
|
0.0291 |
0.0266 |
GoF on F2 |
1.070 |
1.018 |
R
1 [I > 2σ(I)]a |
0.0724 |
0.0765 |
wR2 (all data)b |
0.2117 |
0.2271 |
Results and discussion
Synthesis
Self-assembly reaction of AgClO4 with a C3-symmetric tridentate N-donor (((benzene-1,3,5-triyltris(methylene))tris(oxy))tris(benzene-1,4-diyl))tris(methylene)triisonicotinate (L) in the mixture of methanol and 1,4-dioxane produced single crystals of [(2dioxane)@Ag(ClO4)L] in high yield, as shown in Scheme 1. The reaction was initially conducted in the 1
:
1 mole ratio of Ag(I) ion
:
L, but the species formation was not significantly influenced by the reactant mole ratios or concentration, indicating that the crystalline product is thermodynamically stable. Its composition and structure were confirmed by elemental analyses, IR (Fig. S1†), thermal analysis (Fig. S2†), and single crystal X-ray diffraction. The colourless crystal was determined to be reasonably stable and insoluble in water and common organic solvents, but was dissociated in dimethyl sulfoxide. Adsorption and separation of xylene isomers were reversibly achieved, as will be explained in detail.
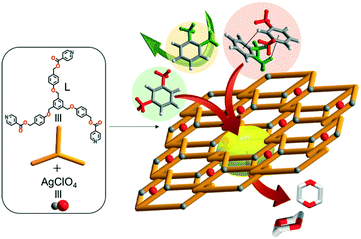 |
| Scheme 1 Adsorption procedure of xylene isomers into pseudo-2D porous networks. | |
Crystal structures
The structures are depicted in Fig. 1, and their relevant bond lengths, bond angles, and guest volumes are listed in Table 2. For [(2dioxane)@Ag(ClO4)L], the silver(I) ion approximates to a trigonal-bipyramidal arrangement with three equatorial N-donors from three Ls (Ag–N = 2.217(4)–2.351(4) Å; N–Ag–N = 105.3(1)–143.2(1)°) and two axial O-donors from the perchlorate anion (Ag⋯OClO3 = 2.765(7); 2.95(1) or 3.051(6) Å). The Ag(I) ions and Ls are defined as a 3-connected node, which produces a zigzag ladder-type 1D coordination polymer consisting of two kinds of 60-membered metallacycles. Each zigzag ladder skeleton is packed in an interpenetration mode, resulting in a pseudo-2D network with open channels of 10.2 × 8.4 Å2. The 1,4-dioxane solvent molecules are nestled within the channels, and the perchlorate anion is positioned in the middle of each channel. The skeletal structure of the SCSC solvent-exchanged species, [(1.5o-X)@Ag(ClO4)L], is basically the same, but the channel dimension is slightly dependent on the exchanged guest molecules. The perchlorate anion was slightly dislocated to accept the o-X, as depicted in Fig. 1(d). The adsorbed o-X was safely nestled in the channel.
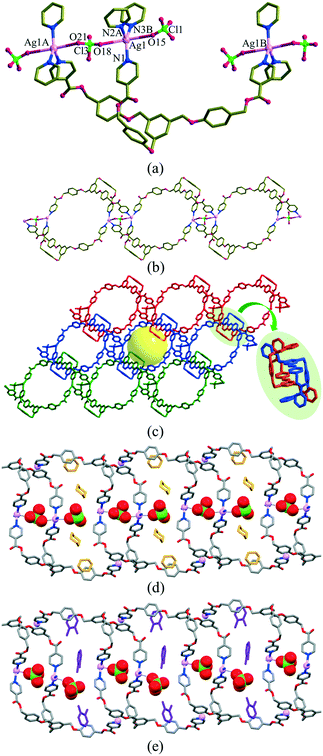 |
| Fig. 1 Single crystal structures of [(2dioxane)@Ag(ClO4)L]. Ball and stick model of a view showing the coordination environment of Ag1. Symmetry operations for A: 2 − x, 2 − y, 1 − z; B: 2 − x, 1 − y, 2 − z; C: x, 1 + y, −1 + z (a). 1D skeletal structure including the coordination environment around Ag(I) (b). A pseudo-2D network via interpenetration of 1D ladders showing the pores in yellow sphere (c). Solvate dioxane molecules nestled in the pores of [(2dioxane)@Ag(ClO4)L] (10.17 × 8.45 Å void dimension) (d) and exchanged o-X molecules in the pores of [(1.5o-X)@Ag(ClO4)L] (10.17 × 9.49 Å2 void volume) (e). The H atoms are omitted for clarity. | |
Table 2 Selected bond lengths (Å), angles (°), and guest volumes
[(2dioxane)@Ag(ClO4)L] |
[(1.5o-X)@Ag(ClO4)L] |
Ag(1)–N(1) |
2.217(4) |
Ag(1)–N(2) |
2.223(4) |
Ag(1)–N(3)#1 |
2.230(4) |
Ag(1)–N(3)#1 |
2.219(4) |
Ag(1)–N(2)#2 |
2.351(4) |
Ag(1)–N(1)#2 |
2.46(4) |
N(1)–Ag(1)–N(3)#1 |
143.22(13) |
N(3)#1–Ag(1)–N(2) |
151.67(14) |
N(1)–Ag(1)–N(2)#2 |
110.57(13) |
N(3)#1–Ag(1)–N(1)#2 |
105.6(14) |
N(3)#1–Ag(1)–N(2)#2 |
105.33(13) |
N(2)–Ag(1)–N(1)#2 |
102.0(14) |
Ag(1)⋯O(18) |
2.765(7) |
Ag(1)⋯O(3E)#2 |
2.595(9) |
Ag(1)⋯O(21)#2 |
2.950(13) |
Ag(1)⋯O(1D) |
2.997(9) |
Ag(1)⋯O(15) |
3.051(6) |
|
|
Guest volume (%) |
21.9 |
Guest volume (%) |
17.9 |
Void dimension (Å) |
10.17 × 8.45 |
Void dimension (Å) |
10.17 × 9.49 |
#1−x + 2, −y + 1, −z + 2 |
|
#1−x, −y + 1, −z |
|
#2−x + 2, −y + 2, −z + 1 |
#2−x, −y, −z + 1 |
Adsorption of xylene isomers in SCSC
The volumes of solvent molecules in [(2dioxane)@Ag(ClO4)L] and [(1.5o-X)@Ag(ClO4)L] were 21.9% (581.3 Å3/2657.2 Å3) and 17.9% (480.7 Å3/2686.6 Å3), respectively, as determined on the basis of PLATON/SOLV.45 For [(2dioxane)@Ag(ClO4)L], both the thermal stability of the skeleton and the evaporation temperature of the solvent dioxane molecules were checked by thermogravimetric analysis (TGA) and differential scanning calorimetry (DSC). The TGA and DSC overlays showed drastic decomposition at 126 °C, corresponding to the collapse of the skeleton (Fig. S2†) along with evaporation of dioxane molecules in the wide temperature range of 10–93 °C. The guest dioxane molecules were also confirmed by the 1H NMR spectra in Me2SO-d6 (Fig. S3†), even though the skeleton was dissociated in the solution.
In this context, the single crystals of [(2dioxane)@Ag(ClO4)L] were employed for the adsorption of o-X, m-X, and p-X isomers along with their mixtures in the SCSC mode.25,30 Of course, their crystallinity was safely maintained before and after adsorption of the xylene isomers. Their IR spectra remained virtually unchanged (Fig. S1†), suggesting that the skeletal structure was not significantly changed after the guest exchange. The adsorption rate in the SCSC manner was monitored with reference to the 1H NMR spectra in Me2SO-d6. While the crystals were simply immersed in the respective xylene isomers, the dioxane solvent molecules were slowly exchanged in the order p-X > m-X > o-X at room temperature, as shown in Fig. S4.† An interesting feature is that [(2dioxane)@Ag(ClO4)L] adsorbs o-X into the channel at room temperature, in contrast to [(2dioxane)@Ag(PF6)L], indicating that the counteranion plays an important role in adsorption. The size difference between PF6− (56.2 cm3 mol−1) and ClO4− (52.1 cm3 mol−1) anions46 might have resulted in the different adsorption properties of o-X. The attempted SCSC adsorption of o-X at 5 °C showed a significant temperature effect, as depicted in Fig. 2(a). The difference in pore size between 5 °C and room temperature may be due to the drastic change of adsorption. That is, o-X was not adsorbed into the channel at 5 °C, which was the first temperature effect of the adsorption of xylene isomers; this indicated that the present channel is somewhat flexible.
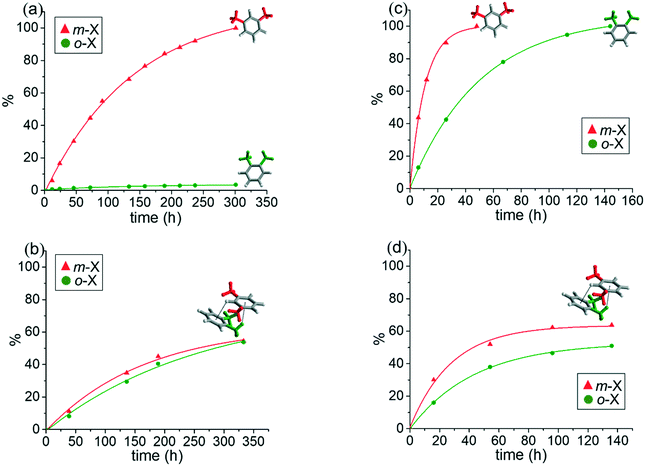 |
| Fig. 2 Exchange rates of m-X and o-X at 5 °C (a) and at room temperature (c); exchange rates of the m-X and o-X (1 : 1) mixture at 5 °C (b) and at room temperature (d). The quantities of absorbed xylene isomers depending on time were determined by 1H NMR spectra in Me2SO-d6. | |
The most important feature identified was the trend of the adsorption of the o-X and m-X, o-X and p-X, and m-X and p-X mixtures. For instance, the 1
:
1 mixture of o-X
:
m-X at 5 °C was adsorbed into the channel in an almost 50
:
50 mole ratio (Fig. 2(b)) after 330 h, in contrast to the non-adsorption of o-X at the same temperature (Fig. 2(a)). Also, the 1
:
1 mixture of o-X and m-X was adsorbed into the channel in a 40
:
60 mole ratio at room temperature after 140 h (Fig. 2(d)), and the 1
:
1 mixture of o-X and p-X was adsorbed in a 22
:
68 mole ratio at 5 °C, and in a 22
:
75 mole ratio at room temperature (Fig. S5†). Such facts suggest that the unique, weak interactions between the xylene isomers are different, and that such weak interaction may be a significant factor in adsorption, as will be explained in detail. The adsorptions at room temperature predictably resulted in increased adsorption, but the adsorption of o-X from the isomer mixture decreased, due to the weakening of the intermolecular interaction. Further experiments in the 1
:
1
:
1 mixture of p-X
:
m-X
:
o-X showed the exchange ratio of 39
:
23
:
15% at 5 °C, and 48
:
28
:
17% at room temperature, as depicted in Fig. S6.† For the 1
:
1
:
1 mixture containing p-X, p-X was predominantly adsorbed into the channel along with the weak mixture effect.
In order to confirm the existence of weak intermolecular interactions between the mixtures including the o-X isomer as the main driving force of the significant mixture effects, computational simulations were performed with the Gaussian 09 development program.47–49 The optimization and energy calculations were done without consideration of the solvent effect. The optimized aggregates depicted in Fig. 3 show that the excimeric-type dimer of o-X and m-X is more stable than each isomer by −8.05 kcal mol−1via the shortest intermolecular CH–π distance (3.78 Å). The dimeric structure of o-X and p-X, meanwhile, is more stable than that of each isomer by −7.49 kcal mol−1 with its short π–π interactions (3.41 Å, 3.56 Å). These energy calculation results were coincident with the adsorption rates on the mixture isomers including o-X. That is, the adsorption rate of the mixture after 300 h was 50
:
50 for o-X and m-X (1
:
1) and 22
:
68 for o-X and p-X.
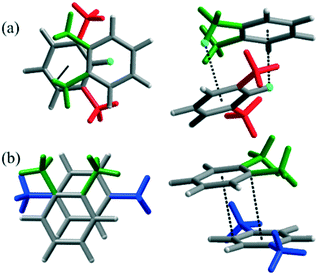 |
| Fig. 3 Computational simulation of the o-X and m-X (1 : 1) mixture showing a stable dimeric structure via CH–π interaction (3.78 Å) (ΔE = −8.05 kcal mol−1) ((a); top view (left), side view (right)) and o-the X and p-X (1 : 1) mixture showing a stable dimeric structure via π–π interaction (3.408 Å, 3.555 Å) (ΔE = −7.49 kcal mol−1) ((b); top view (left), side view (right)). The ground states of xylene isomers had been calculated by optimization with the 6-311++G (d,p) basis set using the B3LYP functional. The ground states of the energy of molecules were computed according to density functional theory (DFT). | |
Conclusions
The present pseudo 2D porous crystals were determined to be sensitive to both mixture and temperature effects on the reversible adsorption and separation of xylene isomers in the SCSC guest-exchange mode. This study discloses that the order of adsorption affinity is p-X > m-X > o-X at room temperature. Furthermore, o-X is adsorbed at room temperature and is not adsorbed into the pores at 5 °C. The most important conclusion reached in this study is the perfect adsorption for o-X isomer mixtures, even at 5 °C, via weak excimeric interactions. Furthermore, the porous crystalline materials are a practical template for structural determination of small liquid hydrocarbons in the SCSC state.50 Further research and wide applications of such porous crystals are in progress, the results of which will help to open up key future SCSC technology in the fields of isomeric separation.
Conflicts of interest
There are no conflicts to declare.
Acknowledgements
This work was supported by the National Research Foundation of Korea (NRF) grants funded by the Korean Government [MEST] (2016R1A2B3009532 and 2016R1A5A1009405).
Notes and references
- S. L. James, Metal-organic frameworks, Chem. Soc. Rev., 2003, 32, 276–288 RSC.
- O. M. Yaghi, H. Li, C. Davis, D. Richardson and T. L. Groy, Synthetic strategies, structure patterns, and emerging properties in the chemistry of modular porous solids, Acc. Chem. Res., 1998, 31, 474–484 CrossRef CAS.
- W. A. Maza, R. Padilla and A. J. Morris, Concentration dependent dimensionality of resonance energy transfer in a postsynthetically doped morphologically homologous analogue of UiO-67 MOF with a ruthenium(II) polypyridyl complex, J. Am. Chem. Soc., 2015, 137, 8161–8168 CrossRef CAS.
- P. Samanta, A. V. Desai, S. Sharma, P. Chandra and S. K. Ghosh, Selective recognition of Hg2+ ion in water by a functionalized metal−organic framework (MOF) Based Chemodosimeter, Inorg. Chem., 2018, 57, 2360–2364 CrossRef CAS.
- X. Lian and B. Yan, A postsynthetically modified MOF hybrid as a ratiometric fluorescent sensor for anion recognition and detection, Dalton Trans., 2016, 45, 18668–18675 RSC.
- K. J. Hartlieb, J. M. Holcroft, P. Z. Moghadam, N. A. Vermeulen, M. M. Algaradah, M. S. Nassar, Y. Y. Botros, R. Q. Snurr and J. F. Stoddart, CD-MOF: A versatile separation medium, J. Am. Chem. Soc., 2016, 138, 2292–2301 CrossRef CAS.
- S. Horike, S. Shimomura and S. Kitagawa, Soft porous crystals, Nat. Chem., 2009, 1, 695–704 CrossRef CAS.
- T. H. Noh, H. R. Lee, J. Jang and O.-S. Jung, Organization and energy transfer of fused aromatic hydrocarbon guests within anion-confining nano-channel MOFs, Angew. Chem., Int. Ed., 2015, 54, 9284–9288 CrossRef CAS.
- T. R. Cook, Y.-R. Zheng and P. J. Stang, Metal−organic frameworks and self-assembled supramolecular coordination complexes: comparing and contrasting the design, synthesis, and functionality of metal−organic materials, Chem. Rev., 2013, 113, 734–777 CrossRef CAS.
- X. Chena, N. Dinga, H. Zanga, H. Yeungb, R.-S. Zhaoa, C. Chenga, J. Liua and T.-W. D. Chan, Fe3O4@MOF core–shell magnetic microspheres for magnetic solid-phase extraction of polychlorinated biphenyls from environmental water samples, J. Chromatogr. A., 2013, 1304, 241–245 CrossRef.
- L.-H. Xie, M.-M. Xu, X.-M. Liu, M.-J. Zhao and J.-R. Li, Hydrophobic metal–organic frameworks: assessment, construction, and diverse applications, Adv. Sci., 2020, 7, 1901758–1901801 CrossRef CAS.
- T. H. Noh and O.-S. Jung, Recent advances in various metal-organic channels for photochemistry beyond confined spaces, Acc. Chem. Res., 2016, 49, 1835–1843 CrossRef CAS.
- S. Horike, D. Tanaka, K. Nakagawa and S. Kitagawa, Selective guest sorption in an interdigitated porous framework with hydrophobic pore surfaces, Chem. Commun., 2007, 3395–3397 RSC.
- I.-H. Park, E. Lee, S. S. Lee and J. J. Vittal, Chemical patterning in single crystals of metal–organic frameworks by [2+2] cycloaddition reaction, Angew. Chem., Int. Ed., 2019, 58, 14860–14864 CrossRef CAS.
- A. Song, J. Ma, D. Xu and R. Li, Adsorption and diffusion of xylene isomers on mesoporous beta zeolite, Catalysts, 2015, 5, 2098–2114 CrossRef CAS.
- D. Kim, S. Park and O.-S. Jung, In situ two-steps crystallization: transformation of kinetic into thermodynamic crystals, Cryst. Growth Des., 2019, 19, 2019–2023 CrossRef CAS.
- C. Janiak, Engineering coordination polymers towards applications, Dalton Trans., 2003, 2781–2804 RSC.
- L. Chen, D.-D. Zhu, G.-J. Ji, S. Yuan, J.-F. Qian, M.-Y. He, Q. Chen and Z.-H. Zhang, Efficient adsorption separation of xylene isomers using a facilely fabricated cyclodextrin-based metal–organic framework, J. Chem. Technol. Biotechnol., 2018, 93, 2898–2905 CrossRef CAS.
- Y. Yang, P. Bai and X. Guo, Separation of xylene isomers: a review of recent advances in materials, Ind. Eng. Chem. Res., 2017, 56, 14725–14753 CrossRef CAS.
- J.-M. Lin, C.-T. He, P.-Q. Liao, R.-B. Lin and J.-P. Zhang, Structural, energetic, and dynamic insights into the abnormal xylene separation behavior of hierarchical porous crystal, Sci. Rep., 2015, 5, 11537–11546 CrossRef CAS.
- M. I. Gonzalez, M. T. Kapelewski, E. D. Bloch, P. J. Milner, D. A. Reed, M. R. Hudson, J. A. Mason, G. Barin, C. M. Brown and R. Jeffrey, Long separation of xylene isomers through multiple metal site interactions in metal−organic frameworks, J. Am. Chem. Soc., 2018, 140, 3412–3422 CrossRef CAS.
- H. Ju, I.-H. Park, E. Lee, S. Kim, J. H. Jung, M. Ikeda, Y. Habata and S. S. Lee, Guest-triggered assembly of zinc(II) supramolecular isomers with accompanying dimensional change and reversible single-crystal-to-single-crystal transformation, CrystEngComm, 2016, 18, 1600–1608 RSC.
- K. Biradha, Y. Hongo and M. Fujita, Crystal-to-crystal sliding of 2D coordination layers triggered by guest exchange, Angew. Chem., Int. Ed., 2002, 41, 3395–3398 CrossRef CAS.
- D. Choi, H. Lee, J. J. Lee and O.-S. Jung, Practical porous matrix for molecular structure determination of general liquid chemicals, Cryst. Growth Des., 2017, 17, 6677–6683 CrossRef CAS.
- Y. Wang, W. Fan, X. Wang, Y. Han, L. Zhang, D. Liu, F. Dai and D. Su, Solvent-induced framework-interpenetration isomers of Cu MOFs for efficient light hydrocarbon separation, Inorg. Chem. Front., 2018, 5, 2408–2412 RSC.
- Z. Qiao, A. K. Cheetham and J. Jiang, Identifying the best metal–organic frameworks and unravelling different mechanisms for the separation of pentane isomers, Mol. Syst. Des. Eng., 2019, 4, 609–615 RSC.
- H. Wang, X. Dong, J. Lin, S. J. Teat, S. Jensen, J. Cure, E. V. Alexandrov, Q. Xia, K. Tan, Q. Wang, D. H. Olson, D. M. Proserpio, Y. J. Chaba, T. Thonhauser, J. Sun, Y. Han and J. Li, Topologically guided tuning of Zr-MOF pore structures for highly selective separation of C6 alkane isomers, Nat. Commun., 2018, 9, 1745–1755 CrossRef.
- L. Alaerts, C. E. A. Kirschhock, M. Maes, M. A. van der Veen, V. Finsy, A. Depla, J. A. Martens, G. V. Baron, P. A. Jacobs, J. F. M. Denayer and D. E. De Vos, Selective adsorption and separation of xylene isomers and ethylbenzene with the microporous vanadium(IV) terephthalate MIL-47, Angew. Chem., Int. Ed., 2007, 46, 4293–4297 CrossRef CAS.
- S. Hyun, L. Yang, D. Kim and O.-S. Jung, Stable suprachannels via a columnar cyclodimeric ensemble: exchange and matrix of various liquid guests in SCSC fashion, Dalton Trans., 2019, 48, 10927–10932 RSC.
- H. Li, M. Eddaoudi, M. O'Keeffe and O. M. Yaghi, Design and synthesis of an exceptionally stable and highly porous metal-organic framework, Nature, 1999, 402, 276–279 CrossRef CAS.
- S. Ma and H.-C. Zhou, A metal−organic framework with entatic metal centers exhibiting high gas adsorption affinity, J. Am. Chem. Soc., 2006, 128, 11734–11735 CrossRef CAS.
- H. T. Kwon and H.-K. Jeong, In situ synthesis of thin zeolitic-imidazolate framework ZIF-8 membranes exhibiting exceptionally high propylene/propane separation, J. Am. Chem. Soc., 2013, 135, 10763–10768 CrossRef CAS.
- H. C. Jeong and T. H. Noh, Solvent-ratio dependency of polymorphism of silver(I) complexes containing 1,2,3-tris(isoniconitoyloxy)benzene, Bull. Korean Chem. Soc., 2019, 40, 1068–1075 CrossRef CAS.
- A. Knebel, B. Geppert, K. Volgmann, D. I. Kolokolov, A. G. Stepanov, J. Twiefel, P. Heitjans, D. Volkmer and J. Caro, Defibrillation of soft porous metal-organic frameworks with electric fields, Science, 2017, 358, 347–351 CrossRef CAS.
- B. Barton, E. C. Hosten and P. L. Pohl, Discrimination between o-xylene, m-xylene, p-xylene and ethylbenzene by host compound (R,R)-(-)-2,3-dimethoxy-1,1,4,4-tetraphenylbutane-1,4-diol, Tetrahedron, 2016, 72, 8099–8105 CrossRef CAS.
- J. Zhang, X. Zhu, G. Wang, P. Wang, Z. Meng and C. Li, The origin of the activity and selectivity of silicalite-1 zeolite for toluene methylation to para-xylene, Chem. Eng. J., 2017, 327, 278–285 CrossRef CAS.
- X. Wu, W. Wei, J. Jiang, J. Caro and A. Huang, High-flux high-selectivity metal–organic framework MIL-160 membrane for xylene isomer separation by pervaporation, Angew. Chem., Int. Ed., 2018, 57, 15354–15358 CrossRef CAS.
- A. E. Khudozhitkov, S. S. Arzumanov, D. I. Kolokolov and A. G. Stepanov, Dynamics of xylene isomers in MIL-53 (Al) MOF probed by solid state 2H NMR, Microporous Mesoporous Mater., 2020, 300, 110115–110124 CrossRef.
- S. Mukherjee, B. Joarder, B. Manna, A. V. Desai, A. K. Chaudhari and S. K. Ghosh, Framework-flexibility driven selective sorption of p-xylene over other isomers by a dynamic metal-organic framework, Sci. Rep., 2014, 4, 5761–5767 CrossRef CAS.
- S. Lee, D. Kim and O.-S. Jung, Pseudo-2D porous networks via interpenetration of 1D zigzag ladder-type coordination polymers: adsorption and separation of xylene isomers, Cryst. Growth Des., 2020, 20, 3601–3604 CrossRef CAS.
- Q. Tan, H. Huang, Y. Peng, Y. Chang, Z. Zhang, D. Liu and C. Zhong, A temperature-responsive smart molecular gate in a metal-organic framework for task-specific gas separation, J. Mater. Chem. A, 2019, 7, 2657–26579 Search PubMed.
- J. W. Shin, K. Eom and D. J. Moon, BL2D-SMC, the supramolecular crystallography beamline at the Pohang Light Source II, Korea, J. Synchrotron Radiat., 2016, 23, 369–373 CrossRef.
-
Z. Otwinowski and W. Minor, Processing of X-ray diffraction data collected in oscillation mode, Methods in Enzymology, ed. C. W. Carter Jr. and R. M. Sweet, Academic Press, New York, 1997, vol. 276, p. 307 Search PubMed.
- G. M. Sheldrick, Crystal structure refinement with SHELXL, Acta Crystallogr., Sect. C: Struct. Chem., 2015, 71, 3–8 Search PubMed.
-
G. M. Sheldrick, SADABS, A Program for empirical absorption correction of area detector data, University of Göttingen, Göttingen, Germany, 1996 Search PubMed.
- S. Kaabel, J. Adamson, F. T. Anniina, K. E. Kalenius, M. Oeren, M. Reimund, E. Prigorchenko, A. Lookene, H. J. Reich, K. Rissanen and R. Aav, Chiral hemicucurbit[8]uril as an anion receptor: selectivity to size, shape and charge distribution, Chem. Sci., 2017, 8, 2184–2190 RSC.
- A. Ghysels, M. Vandichel, T. Verstraelen, M. A. van der Veen, D. E. De Vos, M. Waroquier and V. Van Speybroeck, Host–guest and guest–guest interactions between xylene isomers confined in the MIL-47(V) pore system, Theor. Chem. Acc., 2012, 131, 1234–1246 Search PubMed.
- D. Vasudevan, R. L. Fimmen and A. B. Francisco, Tracer-grade rhodamine WT: structure of constituent isomers and their sorption behaviour, Environ. Sci. Technol., 2001, 35, 4089–4096 CrossRef CAS.
- M. F. S. Khan, J. Wu, B. Liu, C. Cheng, M. Akbar, Y. Chai and A. Memon, Fluorescence and photophysical properties of xylene isomers in water: with experimental and theoretical approaches, R. Soc. Open Sci., 2018, 5, 171719–171730 CrossRef.
- Y. Inokuma, S. Yoshioka, J. Ariyoshi, T. Arai, Y. Hitora, K. Takada, S. Matsunaga, K. Rissanen and M. Fujita, X-ray analysis on the nanogram to microgram scale using porous complexes, Nature, 2013, 495, 461–466 CrossRef CAS.
Footnote |
† Electronic supplementary information (ESI) available: Experimental details and crystal structure determination. TGA curves of [(2dioxane)@Ag(ClO4)L] and [(1.5o-X)@Ag(ClO4)L]; 1H NMR spectra and IR spectra of the ligand, [(2dioxane)@Ag(ClO4)L], [(1.5p-X)@Ag(ClO4)L], [(1.5m-X)@Ag(ClO4)L] and [(1.5o-X)@Ag(ClO4)L]. CCDC reference numbers 2017538 and 2017539. For ESI and crystallographic data in CIF or other electronic format see DOI: 10.1039/d0ce01458c |
|
This journal is © The Royal Society of Chemistry 2021 |