DOI:
10.1039/D0CE01363C
(Paper)
CrystEngComm, 2021,
23, 216-220
Synthesis, structure, and heterogeneous Fenton reaction of new Cu(II)-based discrete Cu2Lx coordination complexes†
Received
19th September 2020
, Accepted 30th October 2020
First published on 30th October 2020
Abstract
Discrete and polymeric coordination complexes, namely [Cu2L4(ClO4)4]·2CH2Cl2·CH3OH (1), [Cu2L2(NO3)4(CH3OH)2] (2) and {[Cu2L4(NO3)4]}n (3), were obtained using bridged pyridine ligand 4-methyl-2,6-bis(pyridinyl-3-ethynyl)aniline (L) and Cu(II) salts in CH2Cl2/MeOH. The structure of the Cu2L4 cage or Cu2L2 macrocycle was generated depending on the selection of the anion (ClO4−/NO3−). The heterogeneous Fenton reaction experiments using H2O2 showed that discrete coordination complexes are good photocatalysts in which the Cu–N bond plays a major role in the photocatalytic degradation. The degradation mechanism could be described as a synergistic process of the Fenton reaction and high-valence copper ions using t-butanol as the hydroxyl radical scavenger. The photocatalytic degradation of methylene blue (MB) experiments showed that the photodegradation efficiency of the coordination complexes is higher than that of the polymeric Cu-MOFs.
Introduction
Coordination polymers (CPs) constructed from organic ligands and metal ions have significant applications in small molecular separation,1,2 gas storage,3,4 luminescence materials,5 heterogeneous catalysts,6,7 and photocatalytic degradation.8,9 Among these, photocatalytic degradation, which is based on the presence of metal nodes or clusters, has attracted considerable attention.10 MOFs are a class of porous CPs, and they have a special application prospect in the field of catalysis, particularly in photocatalysis.10 Numerous studies have reported the degradation of various dyes such as methylene blue under UV or Xe lamp irradiation with MOFs as photocatalysts.11–15 Despite the fruitful achievements, CP or MOF materials could be decomposed as the photo-degradation reaction proceeds and release a lot of metal ions. However, metal ions dissolved in water could cause secondary pollution,16 which is a serious problem in the field of environmental science.17 This problem could be effectively solved by introducing hydrogen peroxide into the photocatalytic system. The presence of hydrogen peroxide can not only promote the photocatalytic efficiency of CPs or MOFs but also oxidize the metal ions dissolved in water and form solid metal oxides. In this way, it is possible to avoid secondary pollution to water.18 Particularly, the metal ions such as Fe(II) under the presence of H2O2 could be turned to a metal oxide precipitate and can avoid the heavy metal pollution, which was named as the Fenton reaction.19,20 By heating the MOFs as precursors, the carbon-doped hybrid materials with metal oxides could be obtained, which show excellent photocatalytic properties.6 Compared to the heating treatment, the CPs in the presence of H2O2 could act as a sustained-release aggregate to generate hydroxyl radicals and metal oxides. Based on this consideration, discrete coordination compounds have better reactivity. 4-Methyl-2,6-bis(pyridinyl-3-ethynyl)aniline (L) has a pyridyl terminated rigid structure with rich electrons that could coordinate with the transition metal ions to form the MOFs with different structures.21 The ligand can greatly affect the photocatalytic properties of CPs via the ligand-to-metal charge transfer (LMCT) and numerous cages or macrocycles were obtained using similar rigid ligands, which have been reported earlier.22–24 In our reported literature earlier,25 0-D coordinated molecules show higher efficiency than the 1-D coordinated polymer in the photocatalytic degradation process of MB. Herein, we report the coordination structures and photodegradation properties of 0-D and 1-D coordination complexes using Cu(II) salts (ClO4− or NO3− as anions) and L. Coordination cage [Cu2L4(ClO4)4]·2CH2Cl2·CH3OH (1), macrocycle [Cu2L2(NO3)4(CH3OH)2] (2) and coordination polymer{[Cu2L4(NO3)4]}n (3) were obtained with different coordinate modes and photocatalytic activities. Compared to the Cu-MOFs and Ni-MOF,11 the photodegradation efficiency of the coordination complexes is higher than that of the polymeric Cu-MOFs. In the similar discrete coordination molecules, the molecule with a longer Cu-donor bond has a higher photocatalytic effect.
Experimental
Complexes 1 and 2 were obtained as green and blue crystals, respectively, via the diffusion reaction with the CH3OH solution of copper salt and the CH2Cl2 solution of ligand L. Complex 1 is a M2L4 cage, while 2 is having an M2L2 macrocyclic structure. The red crystal of complex 3 was confirmed to be a by-product of the synthesis process of complex 2. The mixed solution containing the crystals of complex 2 was filtered, and the filtrate continued to stand at room temperature. After one week, the red crystals were gradually precipitated out. The results reveal that the anionic species have a significant effect on the structure formation of the coordination complexes,26 which can induce the Cu(II) ions and L to form diverse structures ranging from M2L4 to M2L2 or {M2L4}n. All the Cu-ions are in the N and O donor coordinated environment, which could lead the Cu-units to have the ability to catalyze the generation of hydroxyl radicals and exhibit the photodegradation properties. Complexes 1 and 2 have similar Cu-units but shorter Cu–N bonds and longer Cu–O bonds (Table S2†) than the complexes we reported earlier, and the difference between them and the Cu-MOFs in photocatalytic degradation properties can reveal the factors that affect photocatalytic degradation (Scheme 1).24
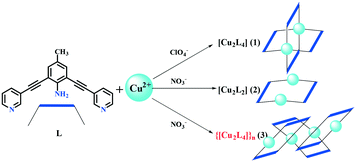 |
| Scheme 1 Synthesis of coordination complexes 1–3. | |
Results and discussion
Complexes 1 and 2 are both crystallized in the triclinic P
space group and show a discrete 0-D structure. The central Cu(II) ion in 1 is in the middle of an octahedral coordination environment of [CuN4O2] in a package of four nitrogen atoms from four pyridyl rings and two oxygen atoms from two perchlorates. All the Cu–N bonds in the unit are 0.01 nm shorter than the coordinated complex based L and cupric acetate, which means that the anions are an important factor.27 Two copper ions acting as two metal junctions are coordinated with four ligands, forming a cubic cage. Two ClO4− anions are inside the cage and fixed by H-bonds (N5–H5A⋯O8ii and N2–H2A⋯O5ii) in solid states of 1, as shown in Fig. 1(a). The central Cu(II) ion in 2 is in the middle of a deformed octahedral coordination environment of [CuN2O4], which was provided by two pyridyl rings, two nitrates, and one methanol molecule. Two copper ions regarded as two metal junction coordinated with two ligands generating a macrocyclic structure, as shown in Fig. 2. The Cu–Cu distance of complex 2 is longer than that of complex 1.
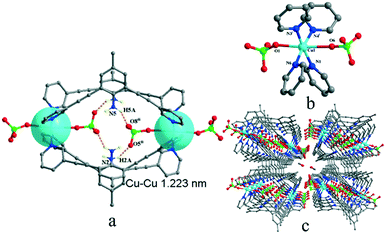 |
| Fig. 1 The structure of coordination complex 1. Symmetry code: ii1 − x, 2 − y, 2 − z. | |
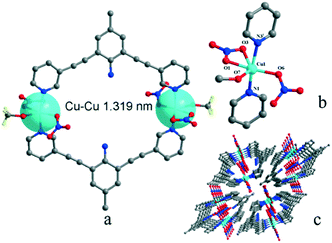 |
| Fig. 2 The structure of the coordination complex 2 symmetry code: i2 − x, 1 − y, 1 − z. | |
In solid states, complex 3 crystallizes in the monoclinic P21/c space group and shows a polymeric 1-D structure. As shown in Fig. 3, the central Cu(II) ion is in the middle of an octahedral coordination environment of [CuN4O2] in a package of four nitrogen atoms from four pyridyl rings and two oxygen atoms from two nitrates. The coordination environment was similar to that of [CuN4O2] in complex 1 but two ligands connect the copper ions to form an M2L2 macrocyclic structure and the adjacent macrocycles and then were further connected to build a 1D Z-type chain structure. The coordination environment of the Cu(II) ion in complex 3 is similar to that in complex 1, but the Cu–O distance (0.248 nm) in complex 3 is between the Cu–O1 and Cu–O6 distance (0.238 nm and 0.258 nm) in complex 1.
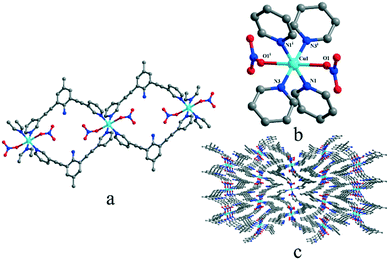 |
| Fig. 3 The structure of coordination complex 3. Symmetry code: i2 − x, 2 − y, 1 − z. | |
There are only simple H-bonds in complex 1 and 3, which could be described as between anion (acceptor) and amino in the ligand (donor). The H-bond system in complex 2 is more complicated than those in 1 and 3 (Tables S3 and S4 in ESI†) because the coordinated molecule methanol was involved in H-bonds, as shown in Fig. 4 and S2(c).†
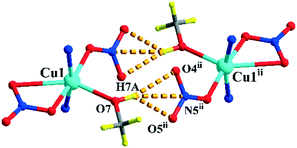 |
| Fig. 4 The H-bonds based on methanol in the complex 2. Symmetry code: ii2 − x, 1 − y, 1 − z. | |
Since numerous copper coordination complexes had the characteristics of the photocatalytic degradation of organic pollutants,8 we verified the photocatalytic effect of complexes 1 and 2 on MB dyes. The experimental results showed that complexes 1 and 2 have good photodegradation properties towards MB. Due to the poor yield of complex 3, the catalytic degradation experiment was not carried out. As shown in Fig. 5, the degradation rate of MB in the H2O2 solution in the presence of 1, 2, and without other additions were 89.96%, 92.31%, and 48.10%, respectively, after 120 min, and further rose to 95.38%, 96.33% and 61.22% after another 120 min under ultraviolet lamp irradiation. Without the irradiation of the Xe-lamp, MB can also be degraded, which indicates that the photodegradation effect of complexes 1 and 2 is favorable. UV-vis spectroscopy shows that complexes 1 and 2 have intense absorption in the visible light region (Fig. S6†), which is responsible for the good performance of the photo-degradation reaction carried out without Xe-lamp irradiation.
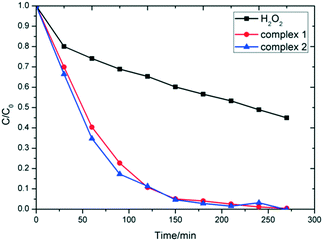 |
| Fig. 5 MB photodegradation diagram of complexes 1, 2 and hydrogen peroxide. | |
According to the literature,28 four active substances exist in the photocatalytic degradation process of MB: hydroxyl radicals, superoxide radicals, photogenerated electron–hole pairs, and high-valence copper ions (for example Cu(III)). Isopropanol and tert-butanol are usually used as hydroxyl radical scavengers, which make the captured free radicals lose their activity.29 The quenching experiments were carried out to verify the mechanism of the reaction process of macrocycle complexes by using t-butanol as the scavenger for ˙OH. The experimental results are shown in Fig. 6.
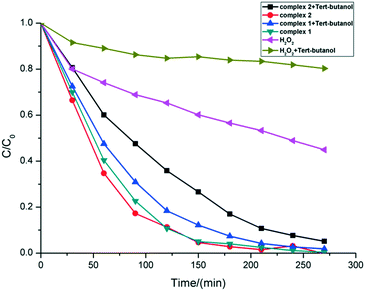 |
| Fig. 6 Photocatalytic degradation of MB in the presence of free radical scavengers. | |
In the presence of tert-butanol, the degradation rate of complexes 1 and 2 on the MB solution reduced from the original 59.65% and 65.38% to 53.48% and 39.91%, respectively, after 60 min of reaction. Although the presence of tert-butanol reduced the degradation rate of the reaction system, it could not completely inhibit the progress of the catalytic reaction. The result showed that ˙OH radicals are not the only active factor in the photodegradation reaction. Since the free radical scavenger could not completely prevent the reaction, there must be some active species to degrade MB molecules. Cheng et al. had mentioned that in a neutral (pH ≈ 7) solution environment when hydrogen peroxide existed, Cu(II) could stimulate H2O2 to generate ˙OH radicals and disproportionate itself to Cu(I) and Cu(III).28 The high valence copper oxide Cu(III) is a very active species to degrade MB and could be turned to Cu(OH)2 that can be decomposed into CuO precipitates.
| Cu(II) + H2O2 → Cu(I) + O2˙− + 2H+ | (1) |
| Cu(I) + H2O2 → Cu(II) + ˙OH + OH− | (2) |
|  | (3) |
The photodegradation mechanism could be proved to be reasonable if the presence of copper oxide is detected in the residue. The X-ray powder diffraction of the residues 1 and 2 was measured after the photodegradation reaction. Three diffraction peaks were found in the PXRD spectrum at 24.540, 32.20, and 38.480, which were consistent with the PXRD data of CuO (PDF #44-0706). These results further indicated that the Cu-units in complexes 1 and 2 could react with H2O2 to generate the ˙OH radical and the high-valence copper ions, and both of them synergistically catalyzed the degradation of MB and finally were turned to CuO solid.
The degradation efficiency depends on the structure and metal nodes of MOFs. We have obtained other Cu-MOFs and coordination complexes and explored the efficiency of photocatalytic degradation in the MB solution. The catalytic activity of the two Cu(II) coordination complexes, namely HKUST-1 and {[Cu2(nic)4]·H2O}n, was based on the carboxylic acid terminated ligand (terephthalic acid and nicotinic acid),30,31 which were determined under the same photocatalytic conditions as complexes 1 and 2. The experimental results reveal that the photocatalytic degradation efficiency of complexes 1 and 2 in the [CuNxOy] coordination environment is 96%, which is 20% higher than that of HKUST-1 having [CuO5]2 coordination environment or {[Cu2(nic)4]·H2O}n owing to [CuN2O4] (Fig. S8†). Under the action of coordination complexes of niacin with copper and nickel ions, the catalytic degradation rate of the coordination complex with {CuN2O4} coordination environment was also significantly higher than that in the coordination environment [NiN2O4] (Fig. S9†).32 The coordination environment plays an important role in catalyzing the decomposition of H2O2 to form hydroxyl radicals in the photocatalytic activity of the coordination complex. The longer Cu-donor distances (0.258 nm in complex 1vs. 0.268 nm in complex 2) the higher photocatalytic degradation rate (Fig. 5) because the metal nodes can generate a large number of hydroxyl radicals and high-valence copper ions.
Conclusions
Two coordination complexes with similar Cu-units were successfully synthesized using the solvent diffusion method. Complex 1 is an M2L4 cage, while complex 2 is an M2L2 macrocycle, and both of them could show high photodegradation efficiency under only ultraviolet lamp irradiation. The existence of the Cu–N bonds was an important factor for photocatalytic degradation, and the mechanism of the photocatalytic degradation of the MB solution could be described as the hydroxyl free radical and high-valence copper ions (Cu(III)) generated in the slow release process of Cu(II) ions from the bulk crystal of the coordination complexes.
Conflicts of interest
There are no conflicts to declare.
Acknowledgements
We are grateful for the financial support from Natural Science Foundation of Shandong Province (Grant No. ZR2019BB041), National Key R&D Program of China (2018YFC0807900) and Scientific Research Foundation of Shandong University of Science and Technology for Recruited Talents.
References
- H. C. Zhou, J. R. Long and O. M. Yaghi, Chem. Rev., 2012, 112, 673–674 CrossRef CAS.
- Y. Okamoto and T. Ikai, Chem. Soc. Rev., 2008, 37, 2593–2608 RSC.
- D. Bradshaw, A. Garai and J. Huo, Chem. Soc. Rev., 2012, 41, 2344–2381 RSC.
- M. Bosch, S. Yuan, W. Rutledge and H. C. Zhou, Acc. Chem. Res., 2017, 50, 857–865 CrossRef CAS.
- L. Y. Yao, F. K. Hau and V. W. Yam, J. Am. Chem. Soc., 2014, 136, 10801–10806 CrossRef CAS.
- A. Bavykina, N. Kolobov, I. S. Khan, J. A. Bau, A. Ramirez and J. Gascon, Chem. Rev., 2020, 120, 8468–8535 CrossRef CAS.
- W. Gong, X. Chen, H. Jiang, D. Chu, Y. Cui and Y. Liu, J. Am. Chem. Soc., 2019, 141, 7498–7508 CrossRef CAS.
- T. Zhang and W. Lin, Chem. Soc. Rev., 2014, 43, 5982–5993 RSC.
- Y. Xie, Z. Fang, L. Li, H. Yang and T. F. Liu, ACS Appl. Mater. Interfaces, 2019, 11, 27017–27023 CrossRef CAS.
- M. B. Chambers, X. Wang, L. Ellezam, O. Ersen, M. Fontecave, C. Sanchez, L. Rozes and C. Mellot-Draznieks, J. Am. Chem. Soc., 2017, 139, 8222–8228 CrossRef CAS.
- L.-S. Cui, X.-M. Meng, Y.-G. Li, K.-R. Huang, Y.-C. Li, J.-Q. Long and P.-F. Yao, CrystEngComm, 2019, 21, 3798–3809 RSC.
- F. Li, D. Wang, Q.-J. Xing, G. Zhou, S.-S. Liu, Y. Li, L.-L. Zheng, P. Ye and J.-P. Zou, Appl. Catal., B, 2019, 243, 621–628 CrossRef CAS.
- M. Zhang, L. Wang, T. Zeng, Q. Shang, H. Zhou, Z. Pan and Q. Cheng, Dalton Trans., 2018, 47, 4251–4258 RSC.
- X. Sun, J. Zhang, X. Yuan and Z. Fu, CrystEngComm, 2019, 21, 5563–5567 RSC.
- C. Ritchie, V. Baslon, E. G. Moore, C. Reber and C. Boskovic, Inorg. Chem., 2012, 51, 1142–1151 CrossRef CAS.
- M. Feng, P. Zhang, H. C. Zhou and V. K. Sharma, Chemosphere, 2018, 209, 783–800 CrossRef CAS.
- D. Jiang, A. Urakawa, M. Yulikov, T. Mallat, G. Jeschke and A. Baiker, Chem. – Eur. J., 2009, 15, 12255–12262 CrossRef CAS.
- Q. K. Liu, J. P. Ma and Y. B. Dong, J. Am. Chem. Soc., 2010, 132, 7005–7017 CrossRef CAS.
- M. Cheng, C. Lai, Y. Liu, G. Zeng, D. Huang, C. Zhang, L. Qin, L. Hu, C. Zhou and W. Xiong, Coord. Chem. Rev., 2018, 368, 80–92 CrossRef CAS.
- J. K. Kim, F. Martinez and I. S. Metcalfe, Catal. Today, 2007, 124, 224–231 CrossRef CAS.
- P. Wang, J. Zhao, Z. Wang, D. Cao and J. Xu, Chin. J. Org. Chem., 2011, 31, 757–761 CAS.
- J. Lee, S. Park, D. Kim, Y.-A. Lee and O.-S. Jung, Inorg. Chem. Front., 2020, 7, 1546–1552 RSC.
- S. Freye, J. Hey, A. Torras-Galan, D. Stalke, R. Herbst-Irmer, M. John and G. H. Clever, Angew. Chem., Int. Ed., 2012, 51, 2191–2194 CrossRef CAS.
- Q. B. Wang, X. Li, H. Y. Wang, W. X. Lu and P. Wang, Chin. J. Inorg. Chem., 2020, 36, 233–240 Search PubMed.
- X. Li, H. A. Zhao, J. Su, J. Y. Cheng and P. Wang, Chin. J. Inorg. Chem., 2019, 35, 109–115 CAS.
- H. Wei, Y. Liu, T. Y. Gopalakrishna, H. Phan, X. Huang, L. Bao, J. Guo, J. Zhou, S. Luo, J. Wu and Z. Zeng, J. Am. Chem. Soc., 2017, 139, 15760–15767 CrossRef CAS.
- F. Baig, K. Rangan, S. M. Eappen, S. K. Mandal and M. Sarkar, CrystEngComm, 2020, 22, 751–766 RSC.
- L. Cheng, M. Wei, L. Huang, F. Pan, D. Xia, X. Li and A. Xu, Ind. Eng. Chem. Res., 2014, 53, 3478–3485 CrossRef CAS.
- A. Kochem, M. Orio, C. Philouze, H. Jamet, A. du Moulinet d'Hardemare and F. Thomas, Eur. J. Inorg. Chem., 2011, 2011, 45–48 CrossRef.
- Q. Qian, P. A. Asinger, M. J. Lee, G. Han, K. Mizrahi Rodriguez, S. Lin, F. M. Benedetti, A. X. Wu, W. S. Chi and Z. P. Smith, Chem. Rev., 2020, 120, 8161–8266 CrossRef CAS.
- L. Guang-Xiang, Chin. J. Inorg. Chem., 2013, 29, 1914–1920 Search PubMed.
- C. Gupta, Synth. React. Inorg., Met.-Org., Nano-Met. Chem., 2010, 40, 5–11 CrossRef CAS.
Footnote |
† Electronic supplementary information (ESI) available. CCDC 1986794 for 1, 1986795 for 2, 1986796 for 3. For ESI and crystallographic data in CIF or other electronic format see DOI: 10.1039/d0ce01363c |
|
This journal is © The Royal Society of Chemistry 2021 |