DOI:
10.1039/D1BM00245G
(Paper)
Biomater. Sci., 2021,
9, 3675-3691
The effects of VEGF-centered biomimetic delivery of growth factors on bone regeneration†
Received
10th February 2021
, Accepted 1st April 2021
First published on 5th April 2021
Abstract
It is accepted that biomimetic supply of signaling molecules during bone regeneration can provide an appropriate environment for accelerated new bone formation. In this study, we developed a growth factor delivery system based on porous particles and a thermosensitive hydrogel that allowed fast, continuous, and delayed/continuous release of growth factors to mimic their biological production during bone regeneration. It was observed that the Continuous group (continuous release of growth factors) provides a better environment for the osteogenic differentiation of hPDCs than the Biomimetic group (biomimetic release of growth factors), and thus is anticipated to promote bone regeneration. However, contrary to expectation, the Biomimetic group promoted significant new bone formation compared to the Continuous group. From the systematic cell culture experiments, the initial supply of VEGF was considered to have more favorable effects on the osteoclastogenesis than osteogenesis, which may hinder bone regeneration. Our results indicated that the continuous supply of VEGF (in particular, at early stage) from VEGF-loaded biomaterial might not be conducive to new bone formation. Therefore, we suggest that a biomimetic supply of growth factors is a more pivotal parameter for sufficient tissue regeneration. Its use as a molecular delivery system may also serve as a useful tool for the investigation of biological processes and molecules during tissue regeneration processes.
Introduction
Despite advances in graft materials and surgical techniques over the past several decades, bone regeneration in large defects and/or in patients with poor wound healing microenvironments remains as a challenge for surgeons.1–3 It is known that an ideal combination of biological cues (e.g., osteogenic cells, scaffolding structures, and signaling molecules) observed in small bony defects can lead to perfect bone reconstruction. A bone autograft, which can provide these fundamental biological cues and thus allow a better environment for bone healing, is considered the gold standard to treat bony defects in clinical practice.4 However, limited amounts of donor bone and the high risk of donor site morbidity (pain, sensory impairment, hematoma formation, hemorrhage, and infection) are considered hurdles for the wide use of bone autografts.5,6 In recent years, the delivery of signaling molecules from scaffolding matrices to mimic the biological environment during bone regeneration has gained interest as an alternative to bone autografts. This approach, known as in situ tissue engineering, minimizes the psychological and physical burden on both patients and surgeons, and reduces the cost and time needed for bone harvesting.7–10
The bone healing process is divided into four stages: inflammation, fibrous tissue formation, bony callus formation, and bone remodeling.11 During each of these processes, specific signaling molecules induce normal bone reconstruction.11–14 During the inflammation stage, osteoprogenitor cells are recruited to the bony defect by chemoattractant growth factors [e.g., platelet-derived growth factor (PDGF) and bone morphogenetic proteins (BMPs)].11,15,16 Osteogenesis and angiogenesis are then simultaneously and/or sequentially stimulated by BMPs, vascular endothelial growth factor (VEGF), and basic fibroblast growth factor (bFGF).11 It is believed that the biomimetic release profile of the signaling molecules may provide an appropriate biological environment for ideal bone healing. Although the drug delivery system (DDS) for growth factors is considered a potential solution to simulate the biological environment during bone regeneration, delivery matrices that allow a simultaneous and simple sequential release profile, as opposed to a biomimetic release profile, have been developed.17–26 However, well-defined delivery systems that can precisely regulate the release profiles of signaling molecules have not yet been designed.
The main aims in this study were (i) to develop a delivery matrix that mimics the sophisticated release behavior of key signaling molecules during bone regeneration [e.g., PDGF (initial expression up to ∼3 days), BMP-2 (continuous expression over ∼20 days), and VEGF (continuous expression after ∼14 days)]11 and (ii) to confirm the superiority of a biomimetic release profile compared to a conventional (fast and continuous) profile during bone regeneration. To achieve these goals, we prepared two kinds of particles with different pore structures [particles with a micron-sized pore structure (MP) and particles with a leaf-stacked structure (LSS)] that would allow the fast and continuous release of signaling molecules, respectively.27 For the delayed/continuous release of signaling molecules, pluronic mixture-infiltrated LSS particles were prepared. The thermosensitive pluronic mixture can protect signaling molecules immobilized on pore surface of particles,28 thereby delaying their release for a specific period of time. We prepared particles with different release patterns (i.e., fast, continuous, and biomimetic) for key growth factors (PDGF-BB, BMP-2, and VEGF) involved in bone regeneration using the particles (Fig. 1). The Biomimetic group was realized by the combination of MP particles (for fast release of PDGF), LSS particles (for continuous release of BMP-2), and pluornic mixture-infiltrated LSS particles (for delayed/continuous release of VEGF). The behaviors of each growth factor from the particle systems were investigated. We compared the migration of human periosteum-derived cells (hPDCs) by PDGF, osteogenic differentiation of hPDCs by BMP-2, angiogenic differentiation of human umbilical vein endothelial cells (HUVECs) by VEGF, and bone regeneration in a rat model (calvarial defect). We also conducted in vitro cell culture experiments to explore the use of biomimetic release of growth factors during bone regeneration involving VEGF-induced osteogenesis and osteoclastogenesis.
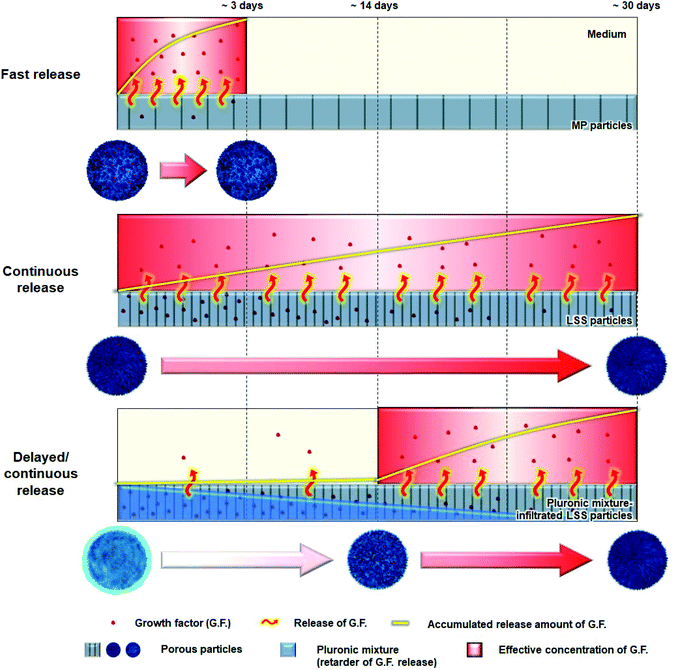 |
| Fig. 1 Schematic diagrams showing three particles with different growth factor release patterns [i.e., fast (MP particles), continuous (LSS particles), and delayed/continuous (pluronic mixture-infiltrated LSS particles)]. | |
Materials and methods
Materials
LSS and MP particles were prepared using polycaprolactone (PCL; MW 81
000 Da; Evonik, Germany) and tetraglycol (Sigma-Aldrich, USA), and NaCl (sieved to size of 25–53 μm; Daejung, Republic of Korea). For the delayed release of signaling molecules from LSS particles, pluronic F127 (Sigma-Aldrich) and pluronic P123 (Sigma-Aldrich) were used. All signaling molecules (growth factors) were purchased from R&D Systems (USA).
Fabrication and characterization of porous particles
LSS particles (size, 1.0–1.4 mm) were prepared using a spray-precipitation method with PCL and tetraglycol, and MP particles (size, 1.0–1.4 mm) were prepared using a melt-molding, particulate-leaching method with dense, sphere-shaped PCL (size, 500–710 μm) and NaCl particles (size, 25–53 μm), as described in our previous study.27 The morphology of each particle was observed by scanning electron microscopy (SEM; S-4300; Hitachi) at the Center for Bio-Medical Engineering Core Facility (Dankook University). Fourier Transform Infrared Spectroscopy (FTIR; 640-IR; Varian) was utilized to estimate any changes of chemical structures of the PCL during each procedure to prepare the particles.
Fabrication and characterization of pluronic mixture-infiltrated LSS particles
The pluronic F127/P123 mixture [1.0/2.5 (w/w)], which can form stable micelles in an aqueous state and allow prolonged water (residence) stability,28 was used for the delayed release of growth factors from LSS particles. The pluronic mixture was dissolved in water (35 wt%) at 4 °C to avoid gel formation. An autoclaved solution was used for the in vitro cell culture and in vivo animal studies. For infiltration of the solution into LSS particles, a syringe containing 20 mg of LSS particles and 1 mL of the cold solution was stored at 4 °C for 3 h under a positive pressure to allow penetration of the viscous solution into the pore spaces. The resultant mixture was stored at room temperature to induce gel formation (sol–gel transition temperature, ∼25 °C). The pluronic mixture around the particles was removed by gentle washing using excess water at room temperature. Morphology of the particles (freeze-dried) was observed using a SEM (S-4300; Hitachi). FTIR (640-IR; Varian) was also used to determine the presence of the pluronic mixture in the LSS particles. To examine the residence stability of the solution that infiltrated the porous LSS particles, the particles were incubated in water at 37 °C for 21 days. At the indicated time points, the water was carefully removed, and the particles were freeze-dried and weighed (Wdry). The solution-infiltrated LSS particles at day 0 were also freeze-dried and weighed (Wdry,initial). The residence stability of the solution in the LSS particles was determined as follows: Pluronic mixture remaining (%) = [Wdry,initial − 20 mg (weight of LSS particles) − (Wdry − 20 mg)]/(Wdry,initial − 20 mg) × 100.
Immobilization and release behavior of growth factors
To immobilize growth factors on the MP (for fast release) and LSS (for continuous or delayed/continuous release) particles, syringes containing 20 mg of each particle type and 1 mL of each growth factor solution [1 μg mL−1 growth factors {PDGF-BB, BMP-2, and VEGF in phosphate-buffered saline (PBS; pH 7.4) supplemented with 1% bovine serum albumin (BSA)}] were stored at 4 °C for 3 h under a positive pressure. Then, the excess growth factor solution was removed from the syringes and freeze-dried. The amount of growth factors immobilized on each particle type was quantified using direct enzyme-linked immunosorbent assay (ELISA).27,29 For the delayed/continuous release of VEGF from LSS particles, the pluronic mixture was infiltrated into VEGF-immobilized LSS particles using the same procedure as described for the residence stability test. The amount of growth factors released from 5 mg of each particle type in 1% BSA containing 1 mL of PBS was detected using an ELISA kit (Duoset; R&D Systems).30 The specifications of each particle system for the in vitro cell culture and in vivo animal studies are summarized in Table 1.
Table 1 Experimental groups for the in vitro and in vivo experiments
Code name |
Release manner |
PDGF-BB |
BMP-2 |
VEGF |
Component ratio.
Delivery matrix for growth factors. BMP, bone morphogenetic protein; LSS, leaf-stacked structure; MP, micron-sized pore structure; PDGF, platelet-derived growth factor; VEGF, vascular endothelial growth factor.
|
PDGF |
Fast |
1a (MP)b |
|
|
BMP |
Continuous |
|
1 (LSS) |
|
VEGF |
Delayed/continuous |
|
|
1 (pluronic mixture-infiltrated LSS) |
Fast |
Fast |
1/3 (MP) |
1/3 (MP) |
1/3 (MP) |
Continuous |
Continuous |
1/3 (LSS) |
1/3 (LSS) |
1/3 (LSS) |
Biomimetic |
Fast |
1/3 (MP) |
|
|
continuous |
|
1/3 (LSS) |
|
Delayed/continuous |
|
|
1/3 (pluronic mixture-infiltrated LSS) |
In vitro cell culture
Cell migration by PDGF-BB.
The migration of hPDCs was estimated using a modified Boyden chamber assay31 for PDGF-BB released from MP particles (fast release) and combined particle systems. All experiments were performed in accordance with the Guidelines of the Ministry of Food and Drug Safety (MFDS) of Korea and the procedure for isolating the osteoprogenitor hPDCs from the human periosteum was approved by the Ethics and Committee of Gyeongsang National University Hospital (2014-05-012).32 Each particle system (15 mg) was incubated in cell growth medium [2 mL, 37 °C, 24-well plates (Corning Inc., USA)] for 7 days, which was changed daily. At designated time intervals (1 and 7 days), hPDCs (passage 3) were seeded onto plate inserts (cell density, 1 × 104 cells per insert; polycarbonate membrane; pore size, 8.0 μm; Corning Inc.) in wells containing particles and incubated for 24 h. Plate inserts were also placed in wells without any particles (no growth factors) and incubated as the Control group. Plate inserts were then removed from the 24-well plate, and any remaining cells on the top of the membrane were carefully removed using a cotton-tipped applicator. Cells that migrated through the membrane pores were fixed in 4% paraformaldehyde and stained with 1% crystal violet (Sigma-Aldrich). The stained cells were examined by optical microscopy (Eclipse Ts2R, Nikon, Japan). A 300-μL aliquot of a 30% glacial acetic acid solution (Sigma-Aldrich) was then incubated with each plate insert for 15 min at room temperature. The optical density (OD) of the resultant solution was measured using a microplate reader (Spark 10M, Tecan, Switzerland) at 575 nm.
Angiogenic differentiation by VEGF.
HUVECs (CC-2517; Lonza, USA) were used as a model to compare in vitro angiogenesis induced by VEGF released from pluronic mixture-infiltrated LSS particles (delayed/continuous release) and combined particle systems. For the tube formation assay, which was used to determine the angiogenic potential of specific signaling molecules,33,34 15 mg of each particle system was plated onto inserts in the wells and incubated in 20 mL of the endothelial cell growth medium (37 °C, 24-well plates) for 21 days.29 The medium was changed every day. At designated time intervals (1, 3, 7, 14, and 21 days), the inserts were placed in 24-well plates containing HUVECs (cell density, 1 × 104 cells per well) that were seeded onto Matrigel (Corning Inc.) and incubated for 24 h. HUVECs seeded onto Matrigel without any particles (no growth factors) were also used as the Control group. The growth pattern of HUVECs on Matrigel was observed by optical microscopy. The total tube length was measured using ImageJ (National Institutes of Health, USA). For quantitative real-time polymerase chain reaction (qRT-PCR) analysis, the HUVECs were seeded into 24-well plates (cell density, 3 × 104 cells per well) and incubated in growth medium for 24 h. Then, plate inserts containing each particle system (15 mg) were placed into each well and incubated. After 3 weeks in culture, qRT-PCR analysis was performed, as described in our previous study.29 CD31 (ThermoFisher Scientific, USA) and Von Willebrand factor (vWF; ThermoFisher Scientific) were used as the angiogenic probes. For immunocytochemical analysis, CD31 (Abcam) and vWF (Abcam) were used as primary antibodies, and NorthernLights 557 (R&D Systems; for CD31) and DyLight 488 (for vWF) were used as secondary antibodies. Cell nuclei were stained with 4′,6-diamidino-2-phenylindole (DAPI; Vector Laboratories, USA) and observed by confocal laser microscopy (LSM 700; Carl Zeiss, Germany).
Osteogenic differentiation by BMP-2.
hPDCs, which are differentiated into osteoblasts by specific signaling molecules, were used to compare osteogenic differentiation induced by BMP-2 released from LSS particles (continuous release) and combined particle systems. Cells were incubated and characterized, as described our previous study.27 Briefly, hPDCs (passage 3) were seeded into 24-well plates (cell density, 3 × 104 cells per well) and incubated with plate inserts containing each particle system (15 mg) for 4 weeks. An hPDC-seeded plate without any particles (no growth factors) was used as the Control group. After 1, 2, and 4 weeks in culture, alkaline phosphatase (ALP) activity was measured using SigmaFast BCIP/NBT staining (Sigma-Aldrich) and an ALP enzyme kit (Abcam). In addition, mineralization was assessed using alizarin red S (ARS; Sigma-Aldrich) staining. A qRT-PCR analysis using Runx2 (early marker) and osteocalcin (late marker) primers was performed, and immunocytochemical analyses using osteogenic primary antibodies to Runx2 and osteocalcin were conducted.
Animal study
To compare bone regeneration rates using particle systems with different growth factor release patterns, Sprague-Dawley rats were used as an animal model (calvarial defect, 200–250 g). A total of 24 rats were divided into four groups: (i) Blank (no particles), (ii) Fast (implantation of a particle system that allows an initial burst release of all growth factors), (iii) Continuous (implantation of a particle system that allows the continuous release of all growth factors), and (iv) Biomimetic [implantation of a particle system that allows the biomimetic release of growth factors (initial burst release of PDGF-BB; continuous release of BMP-2; and delayed/continuous release of VEGF)]. All animal procedures were performed in accordance with the Guidelines for Care and Use of Laboratory Animals of the Ministry of Food and Drug Safety (MFDS) of Korea and approved by the Animal Care Committee of Dankook University of Korea (DKU-17-013). Skull defects (8 mm in diameter) on the parietal calvaria were created using a trephine bur and filled with particles. Soft tissues (periosteum and skin) were then closed using a 5-0 nylon suture (Ethicon, USA). At 4 and 8 weeks after implantation, tissue samples were harvested and fixed in 4% paraformaldehyde. The new bone formation, bone volume, and bone density profile of the bony defect were examined using microcomputed tomography (μ-CT; SkyScan-1176, Bruker, USA). After μ-CT, the tissue specimens were stained with hematoxylin and eosin (H&E) and observed using light microscopy (Eclipse Ts2R; Nikon). More detailed procedures were described in our previous study.35
Culture of THP-1 cells and osteoclast differentiation
As the in vivo analysis suggested that new bone formation was more pronounced in defects filled with particles containing biomimetically released growth factors from PCL scaffolds, we analyzed the effects of VEGF on osteoclast activity in vitro. To examine the osteoclast activity induced by VEGF in in vitro conditions, we first established an osteoclast culture system.
Human THP-1 monocytes (derived from a patient with acute monocytic leukemia; ATCC, USA) were grown in RPMI-1640 (ThermoFisher Scientific) medium supplemented with 10% FBS and 0.05 mM 2-mercaptoethanol (ThermoFisher Scientific) in a 5% CO2 humidified atmosphere at 37 °C. The cells were incubated with 100 ng mL−1 phorbol 12-myristate 13-acetate (PMA, PeproTech, USA) for 3 days to induce adherence to the culture plate. For osteoclast differentiation, the cells were cultured in 24-well plates at a density of 5 × 104 cells per well in an osteoclast induction medium, composed of RPMI-1640 medium supplemented with 10% FBS, 0.05 mM 2-mercaptoethanol, 100 ng mL−1 PMA, 50 ng mL−1 M-CSF (R&D Systems), and 50 ng mL−1 RANKL (R&D Systems). The medium was changed every 2 days during the induction period.
To identify the differentiation of THP-1 cells into osteoclasts after 10 days in culture, the cells were fixed in 4% paraformaldehyde, and nuclei were stained with 300 nM DAPI (Invitrogen, USA). In addition, because differentiated preosteoclasts fuse to form tartrate-resistant acid phosphatase (TRAP)-positive multinucleated cells, TRAP staining was used to characterize osteoclasts, according to the manufacturer's instructions (Kamiya Biomedical Company, USA). TRAP-positive cells with more than three nuclei, as determined by light microscopy (Zeiss Axiostar Plus), were considered osteoclasts.
The expression of osteoclast-specific genes [calcitonin receptor (CTR), cathepsin K, nuclear factor of activated T cells (NFATc1), and TRAP] was examined using Western blot analyses after 5, 10, and 15 days in culture. The membranes were then probed with primary antibodies against CTR, cathepsin K, NFATc1, and TRAP (all from Santa Cruz Biotechnology, USA) to analyze genes specifically expressed in osteoclasts; β-actin (Cell Signaling Technology) was used as a positive control.
Treatment of VEGF on osteoclast differentiation of THP-1 cells
To examine the effects of VEGF on the viability and differentiation of THP-1 cells into osteoclasts, the cells cultured in osteoclast induction medium were treated with 100 ng mL−1 recombinant human VEGF121 (PeproTech). The viability of THP-1 cells during differentiation was assayed using the Cell Counting Kit-8 (Dojindo, Japan) after 4, 8, 12, and 16 days in culture, as previously described. The osteoclastogenic phenotypes of the cells were assessed using TRAP staining after 4 and 12 days in culture.
In addition, to visualize osteoclast markers (αvβ3 integrin and NFATc1), immunofluorescence staining was performed in cells treated with or without VEGF after 1 week in culture as previously described.27 The cells were incubated with anti-αvβ3 (1
:
200 dilution; Abcam) and anti-NFATc1 (1
:
200 dilution; Santa Cruz Biotechnology) overnight at 4 °C. The cells were subsequently incubated with fluorescently labeled secondary antibodies (Abcam) for 1 h at room temperature. Images were examined using fluorescence microscopy.
Direct co-culture system of osteoblasts and osteoclasts
We next established a direct co-culture system for osteoblasts and osteoclasts to mimic in vivo conditions. The isolation and culture of hPDCs were performed as previously described, with the following modifications.27 In this study, hPDCs at passage 1 were used, and THP-1 cells were expanded in RPMI-1640 medium supplemented with 10% FBS and 0.05 mM 2-mercaptoethanol prior to seeding. These cells were then co-cultured in 24-well plates at a density of 5 × 104 cells per well in an equal mixture of osteogenic induction medium and osteoclast induction medium containing 100 ng mL−1 recombinant human VEGF121 (PeproTech).
We examined VEGF binding using immunofluorescence staining of cells with a 1
:
100 dilution of anti-VEGF antibodies (Cell Signaling Technology) after 1 week in co-culture. We then observed VEGF receptor (VEGFR) expression in both osteogenic differentiation medium-induced-hPDCs and osteoclastogenic medium-induced THP-1 cells.
Considering that VEGF-induced angiogenic signaling is primarily mediated via the activation of signal transduction by VEGFR2, the expression of VEGFR2 was analyzed using immunoblotting (VEGFR2 antibody, Cell Signaling Technology) in hPDCs and THP-1 cells after 5, 10, and 15 days in culture.36–38
To evaluate the effects of VEGF on the in vitro osteogenic phenotypes of co-cultured hPDCs and THP-1 cells, the following three groups were prepared: (1) hPDCs and THP-1 cells co-cultured in 24-well plates at a density of 5 × 104 cells per well in osteogenic induction medium without VEGF; (2) hPDCs and THP-1 cells co-cultured in 24-well plates at a density of 5 × 104 cells per well in an equal mixture of osteogenic induction medium and osteoclast induction medium without VEGF; and (3) hPDCs and THP-1 cells co-cultured in 24-well plates at a density of 5 × 104 cells per well in an equal mixture of osteogenic induction medium and osteoclast induction medium containing 100 ng mL−1 recombinant human VEGF121. The osteogenic phenotypes of the cells were assessed, as previously described.27 ALP staining, ALP activity, ARS staining, and calcium content were observed.
We also quantified the levels of macrophage colony-stimulating factor (M-CSF) and receptor activator of nuclear factor-kappa B (NF-κB) ligand (RANKL) secreted by hPDCs after treatment with VEGF to examine its indirect effects on osteoclastogenesis. During the differentiation of hPDCs into osteoblasts with or without 100 ng mL−1 VEGF121 for 48 h, the culture medium was collected after 3, 10, and 20 days in culture, and M-CSF and RANKL levels were measured using ELISA kits (Abcam), according to the manufacturer's instructions. In addition, the effects of exogenous VEGF on the differentiation of hPDCs into osteoblasts were also evaluated using an osteogenic medium containing 100 ng mL−1 VEGF121. ALP staining, ALP activity, ARS staining, and calcium content were observed.
Signaling pathways by VEGF in THP-1 cells
We then investigated signaling pathways induced by 100 ng mL−1 VEGF121 during the differentiation of THP-1 cells into osteoclasts cultured in osteoclastic induction medium by Western blot analysis. Primary antibodies against phospho-ERK, phospho-JNK, phospho-p38, phosphor-mTOR, phospho-PKCα/β, phospho-PKCδ, phospho-PKCθ, phospho-PKD/PKCμ, phospho-PKCζ/λ, and anti-β-actin (all from Cell Signaling Technology) were used.
Statistical analysis
The data obtained in each experiment were expressed as the mean ± standard deviation. Statistical analyses consisted of using a one-way analysis of variance and Tukey's multiple comparison test (SPSS Statistics 22 software, IBM, USA). A P value less than 0.05 was considered statistically significant.
Results and discussion
Characterization of porous particles
Morphologies of porous LSS, pluronic mixture-infiltrated LSS, and MP particles are shown in Fig. 2A and S1.† The interior of the LSS particles was primarily a leaf-stacked structure, and the peripheral regions and surfaces of the particles consisted of a column-shaped porous structure and micron-sized pores, respectively. In pluronic mixture-infiltrated LSS particles, the pluronic is uniformly distributed (coated) in the pore structure of LSS particles. The MP particles (1.0–1.4 mm) show a homogeneous, open-pore structure (pore size, 25–53 μm) throughout the whole matrix. Fig. S2† shows the FTIR spectra of PCL-based particles and raw PCL. It is observed that there are no differences in functional groups (C–H, 2944 & 2865 cm−1; C
O, 1725 cm−1) among the raw PCL, MP particles, and LSS particles, indicating no structural change of PCL during each fabrication procedure. It is also recognized that the pluronic mixture solution can be infiltrated in the LSS particles under positive pressure, and thus uniformly distributed in the particles (C–H, 2889 cm−1; C–O, 1111 cm−1). As reported previously,27 MP particles release an initial burst of BMP-2 during the first 3 days, but LSS particles allow a sustained release of BMP-2 over 36 days. From these findings, MP and LSS particles are expected to be utilized as delivery systems for initial fast and continuous release of growth factors, respectively, which are the basic release patterns needed for the biomimetic release of growth factors.
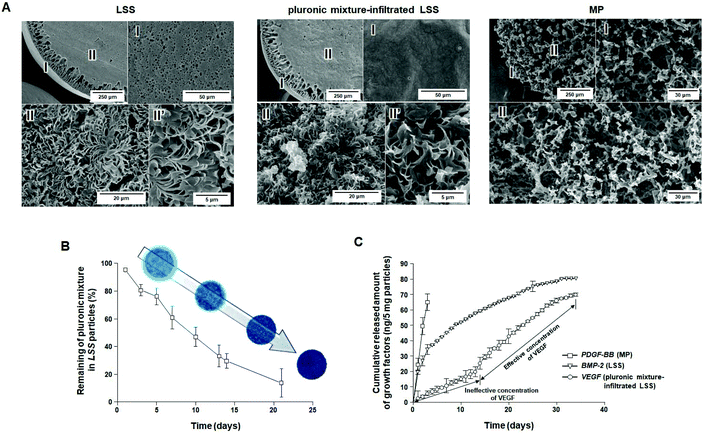 |
| Fig. 2 Characterizations of LSS, pluronic mixture-infiltrated LSS, MP, and particles. (A) SEM photographs showing the surface/cross-section morphology of LSS particle, pluronic mixture-infiltrated LSS particle, and MP particle, (B) stability profiles of pluronic mixture infiltrated in LSS particles (n = 3), and (C) cumulative release behavior of PDGF-BB, BMP-2, and VEGF from MP, LSS, and pluronic mixture-infiltrated LSS particles (n = 3). | |
To investigate the correlation between residence stability of the pluronic mixture in LSS particles and the release pattern of growth factors immobilized on LSS particles, the remaining pluronic mixture in the LSS particles was evaluated over time. The penetration of the solution into the porous particles was confirmed by analyzing the density change in the cold sol-type pluronic mixture during loading. As expected, the gel-type pluronic mixture slowly escaped (i.e., was washed away) from the LSS particles (Fig. 2B), which may allow for the gradual exposure of the growth factor-immobilized surface from the periphery to the central region, and thus steadily increase the amount of growth factor released (Fig. 1). After 21 days, approximately 80% of the pluronic mixture had been released from the LSS particles. From these observations, we anticipate that there exists a time point when effective concentrations of growth factors are released for cell differentiation/tissue regeneration, and thus, pluronic mixture-infiltrated LSS particles may be a suitable delivery system for the continuous release of growth factors after a certain time delay.
Immobilization and release behavior of growth factors
To demonstrate the possible biomimetic release of key growth factors (PDGF-BB, BMP-2, and VEGF) during bone regeneration, MP, LSS, and pluronic mixture-infiltrated LSS particles were used. The release behaviors of growth factors from each particle system are shown in Fig. 2C, S3, and S4.† As expected, in the case of MP particles, bursts of immobilized PDGF-BB were released for 3 days (∼81% PDGF-BB; initial loading amount: 101.80 ± 1.76 ng per 5 mg) at an effective concentration to induce cell migration [>10 ng mL (ref. 39)]. LSS particles allow the continuous release of BMP-2 for 34 days (∼68%; BMP-2; initial loading amount: 108.12 ± 1.69 ng per 5 mg) at an effective concentration to induce osteogenic differentiation [>0.1 ng mL (ref. 40)]. The pluronic mixture-infiltrated LSS particles were demonstrated to provide a suitable environment for the delayed/continuous release of VEGF [continuous release at an effective concentration for angiogenic differentiation after 14 days (>3 ng mL (ref. 41)]; total release amount ∼67% (initial loading amount: 104.85 ± 1.52 ng per 5 mg)]. The 13-day delay in the release of VEGF is the result of the protection of VEGF on the surfaces of LSS particles by the pluronic mixture. The disappearance of approximately 70% of the pluronic mixture from the LSS particles by day 14 allows sufficient surface exposure for the release of VEGF at an effective concentration (refer to Fig. 2B). Based on these observations, we anticipated that the combination of delivery matrices with different release patterns could provide a biomimetic environment for bone regeneration in terms of growth factor stimulation (initial production of PDGF-BB, continuous stimulation of BMP-2, and retarded production of VEGF).
In vitro cell culture
Cell migration by PDGF-BB.
The migration of hPDCs induced by PDGF-BB released from each particle system was compared. One day after cell seeding, all particle groups that can be stimulated by PDGF-BB induced much faster cell migration than the Control group, as expected (Fig. 3A). However, the migration of hPDCs in the PDGF and Fast groups after 7 days was similar to the Control group, suggesting that the supply of chemoattractant growth factors was below the critical concentration. Migration of hPDCs in the Continuous and Biomimetic groups after 7 days was the result of the continuous supply of bioactive PDGF-BB and/or BMP-2 from LSS particles. BMP-2 is also known as a chemoattractant that can stimulate cell migration during the initial stage of bone regeneration.42,43
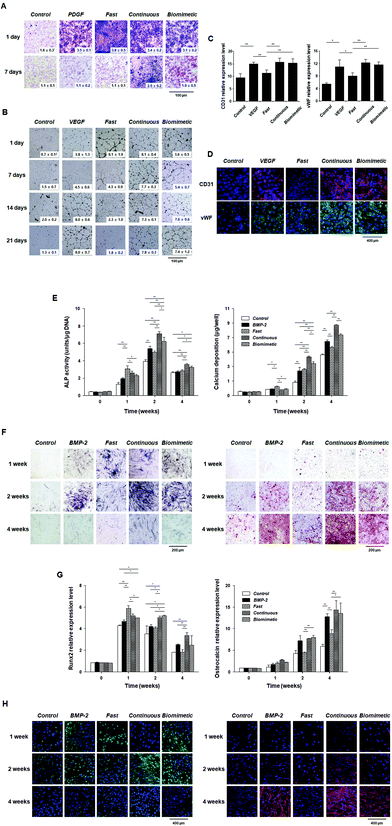 |
| Fig. 3
In vitro analyses of cell migration, angiogenic differentiation, and osteogenic differentiation. (A) Cell migration assays performed in the indicated hPDC groups after 1 and 7 days in culture (†, OD). (B) Tube formation assay performed in the indicated HUVEC groups after 1, 7, 14, and 21 days in culture (‡, total tube length). (C) qRT-PCR analysis of the expression of angiogenic markers (CD31 and vWF) in the indicated HUVEC groups after 3 weeks in culture, and (D) immunocytochemistry of CD31 (red) and vWF (green) expression (blue, cell nuclei) in the indicated hPDC groups after 3 weeks in culture. (E) Quantitative analysis of ALP activity and calcium levels, (F) ALP and ARS staining, (G) qRT-PCR analysis of the expression of osteogenic markers (Runx2 and osteocalcin), and (H) immunocytochemistry of Runx2 (green) and osteocalcin (red) expression (blue, cell nuclei) in the indicated hPDC groups after 1, 2, and 4 weeks in culture (n = 3; *P < 0.05, **P < 0.01). | |
Angiogenic differentiation by VEGF.
In vitro angiogenesis induced by the release of VEGF from each particle system was investigated using HUVECs (Fig. 3B). Tubular structures were formed in groups in which VEGF was supplied at effective concentrations, indicating that the released VEGF had sufficient bioactivity and could thus lead to the angiogenesis of HUVECs. Partial tube formation in the Biomimetic group after 1 and 7 days (despite the low concentration of VEGF) may be the result of PDGF-BB (after 1 day) and/or BMP-2 (after 1 and 7 days) stimulation, which could also induce the angiogenic differentiation of HUVECs.44 To further analyze the angiogenic differentiation of HUVECs, qRT-PCR was used to assess the expression levels of biomarkers CD31 and vWF (Fig. 3C). After 21 days, the VEGF, Continuous, and Biomimetic groups exhibited notably higher expression levels of both markers than the Control and Fast groups. Although the differences among these groups were not significant, the Continuous group showed slightly greater expression levels than the VEGF and Biomimetic groups. The immunocytochemistry results for CD31 and vWF were also consistent with the qRT-PCR results (Fig. 3D).
Osteogenic differentiation by BMP-2.
Fig. 3E–H shows the osteogenic differentiation of hPDCs in response to the release of BMP-2. As expected, the BMP, Continuous, and Biomimetic groups were able to induce effective osteogenic differentiation of hPDCs. Although not all of these differences were significant, the induction potential of osteogenic differentiation among the three groups were as follows: Continuous > Biomimetic > BMP. From this observation, it was recognized that the stimulation of multiple growth factors is more effective for the osteogenic differentiation of stem cells than the stimulation of a single growth factor. These findings are supported by previous studies in which dual growth factor delivery systems (e.g., BMP-2/PDGF-BB and BMP-2/VEGF systems45–47) synergistically accelerated osteogenesis. However, the Fast group was able to induce osteogenic differentiation for only 1 week, after which the induction potential for osteogenic differentiation was similarly weak as in the Control group.
Based on these results, we briefly conclude that (i) each particle system permits the release of target growth factors that are active for a designated period of time in an in vitro environment and (ii) the Continuous group provided a better in vitro environment for the induction of target cell behaviors, including osteogenic differentiation, than the other groups, which is likely the result of the continuous stimulation of all growth factors at effective concentrations.
Animal study
Fig. 4 shows new bone formation after implantation of each particle system. In the Fast group, new bone formation (sea blue in A, blue-magenta-green in C, and magenta in D) and reconstructed bone volumes were similar to those in the Blank group. The Continuous and Biomimetic groups showed notably accelerated bone regeneration compared to the Blank group at 8 weeks, suggesting that continuously supplied bioactive BMP-2 stimulates osteogenic cells for bone reconstruction.27,35 Interestingly, the Biomimetic group showed notably enhanced bone regeneration compared to the Continuous group, unlike the in vitro cell culture experiments in which the Continuous group provided a better environment for osteogenic differentiation compared to the Biomimetic group and was thus expected to promote new bone formation. This observation may be the result of differences between the in vitro and in vivo environments and the inherent limitations of in vitro studies, which do not fully correspond to the physiological environment of a living organism. To demonstrate which biological processes are involved in this process, we focused on the effects of VEGF, which are delayed during early bone healing, on the differentiation of osteoprogenitor cells into osteoblasts and osteoclasts.
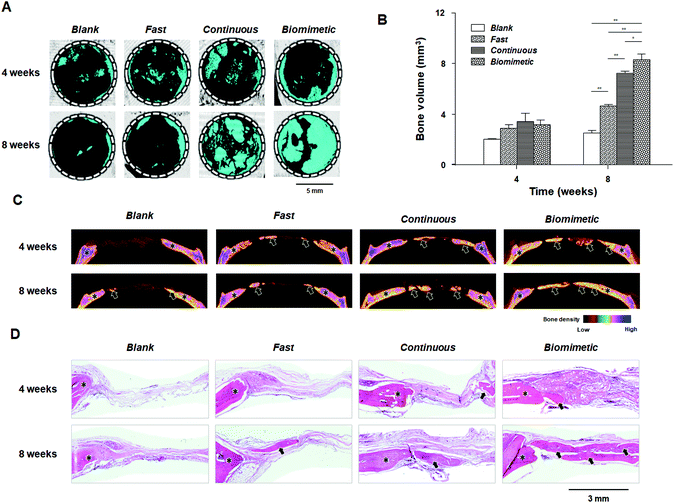 |
| Fig. 4
In vivo bone regeneration in the calvaria after particle implantation. The (A) μ-CT, (B) bone volume, (C) bone density profile, and (D) H&E staining [dot circle, initial bony defect; asterisk, host bone; arrow, new bone; sea blue in (A), blue-magenta-green in (C), and magenta in (D), regenerated bone; n = 3; *P < 0.05, **P < 0.01] results of the indicated systems after 4 and 8 weeks. Biomimetic group shows notably enhanced bone regeneration compared to the Continuous group, unlike the in vitro cell culture experiments in which the Continuous group provided a better environment for osteogenic differentiation compared to the Biomimetic group and is thus expected to promote new bone formation. | |
Characterization of THP-1 cells
Osteoclasts are multinucleated, bone-resorbing cells that are formed via the fusion of monocyte-macrophage precursor cells. When observing the cellular morphology of differentiated THP-1 cells, large, multinuclear cells were clearly observed: the cells had a round appearance (Fig. 5A), the nuclei were stained with DAPI (Fig. 5B), and the cells stained positively for TRAP (Fig. 5C).
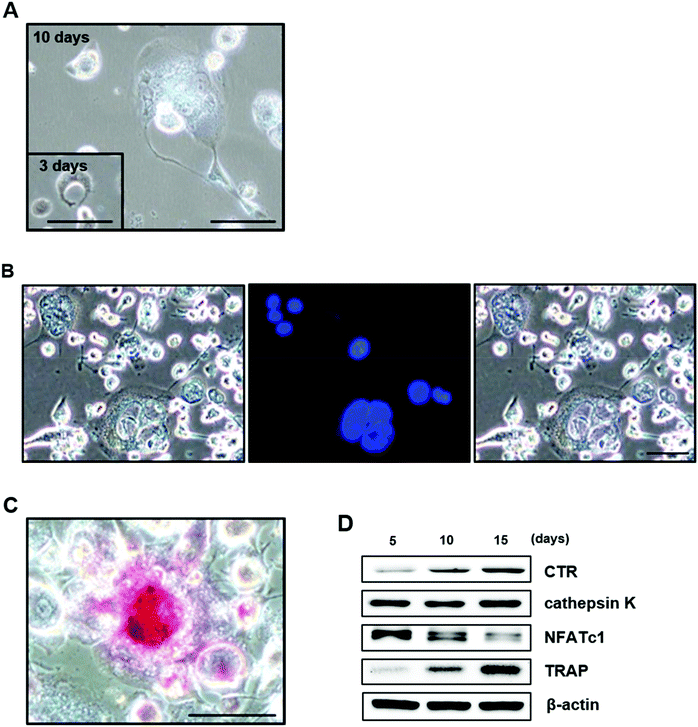 |
| Fig. 5 Characterization of THP-1 cells during their differentiation into osteoclasts. (A) Large, multinucleated cells were clearly observed during the differentiation of THP-1 cells. Cells were stained with DAPI (B) and for TRAP (C). (D) Western blot analysis of osteoclast-specific genes in cells after 5, 10, and 15 days in culture. Scale bar = 100 μm. | |
We also examined the expression of osteoclast-specific genes by Western blotting. NFATc1 was highly expressed after 5 days in culture and decreased over time. CTR and TRAP were weakly expressed after 5 days in culture and increased in a time-dependent manner. The expression of cathepsin K was also clearly observed after 5 days in culture and remained constant throughout the 15-day observation period (Fig. 5D). These results suggest that THP-1 cells differentiate into active osteoclasts, as shown in other studies.48–51
Effects of VEGF on osteoclast differentiation of THP-1 cells
To examine the effects of VEGF on the mechanisms underlying the biomimetic release-mediated promotion of the in vivo bone formation potential of PCL particles treated with growth factors under in vitro conditions, we first examined the effects of VEGF on the viability of THP-1 cells and their differentiation into osteoclasts.
Although exogenous VEGF had no direct effects on cell viability during the differentiation of THP-1 cells into osteoclasts after 4 and 8 days in culture, VEGF clearly increased the viability of THP-1 cells after 12 and 16 days in culture (Fig. 6A). The number of TRAP-positive THP-1 cells was also significantly higher after VEGF treatment (Fig. 6B). The expression of osteoclast-related markers (αvβ3 and NFATc1) was also analyzed using immunocytochemistry. The expression of αvβ3 (green) and NFATc1 (green) was higher in cells treated with VEGF than in those without VEGF (Fig. 6C). In the present study, our results suggest that VEGF, known to stimulate the differentiation of mesenchymal stem cells (MSCs) into osteoblasts by various intracellular mechanisms, directly enhances the viability and differentiation of THP-1 cells into osteoclasts.52–55
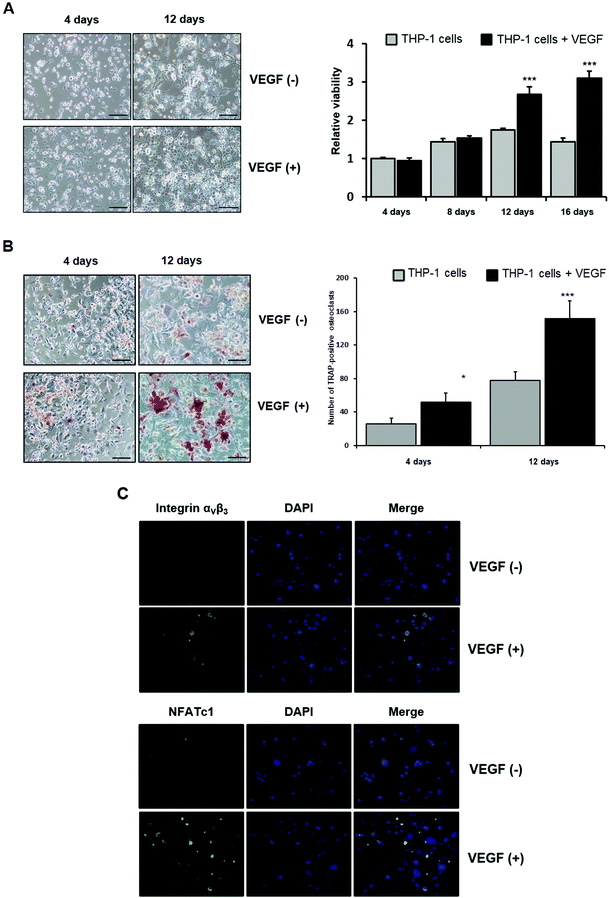 |
| Fig. 6 Effects of VEGF on the differentiation of THP-1 cells into osteoclasts. (A) Viability of THP-1 cells treated with or without VEGF after 12 and 16 days in culture. (B) THP-1 cells were treated with or without VEGF and the number of TRAP-positive osteoclasts was determined after 4 and 12 days in culture. (C) The immunocytochemical expression of αvβ3 and NFATc1. Scale bar = 100 μm. ***P < 0.001. | |
Effects of VEGF on osteogenic phenotypes in co-culture of hPDCs and THP-1 cells
To investigate the effects of VEGF on the in vivo bone formation potential of PCL particles treated (continuous or biomimetic) with growth factors under in vitro conditions, we established a direct co-culture system of osteoblasts and osteoclasts to mimic in vivo conditions. Co-culture systems are useful for the evaluation of in vitro bone formation, as they mimic normal physiological conditions. Although limited evidence exists regarding the optimized osteoblast/osteoclast ratio to use in the direct co-culture system, we chose the ratio of one osteoclast to one osteoblast, using a previously published method.48 In the present study, hPDCs and THP-1 cells in co-culture grew well in an equal mixture of osteogenic induction medium and osteoclast induction medium. The hPDCs exhibited a fibroblastic appearance in multiple layers, and large, multinucleated THP-1 cells were also observed (Fig. 7A).
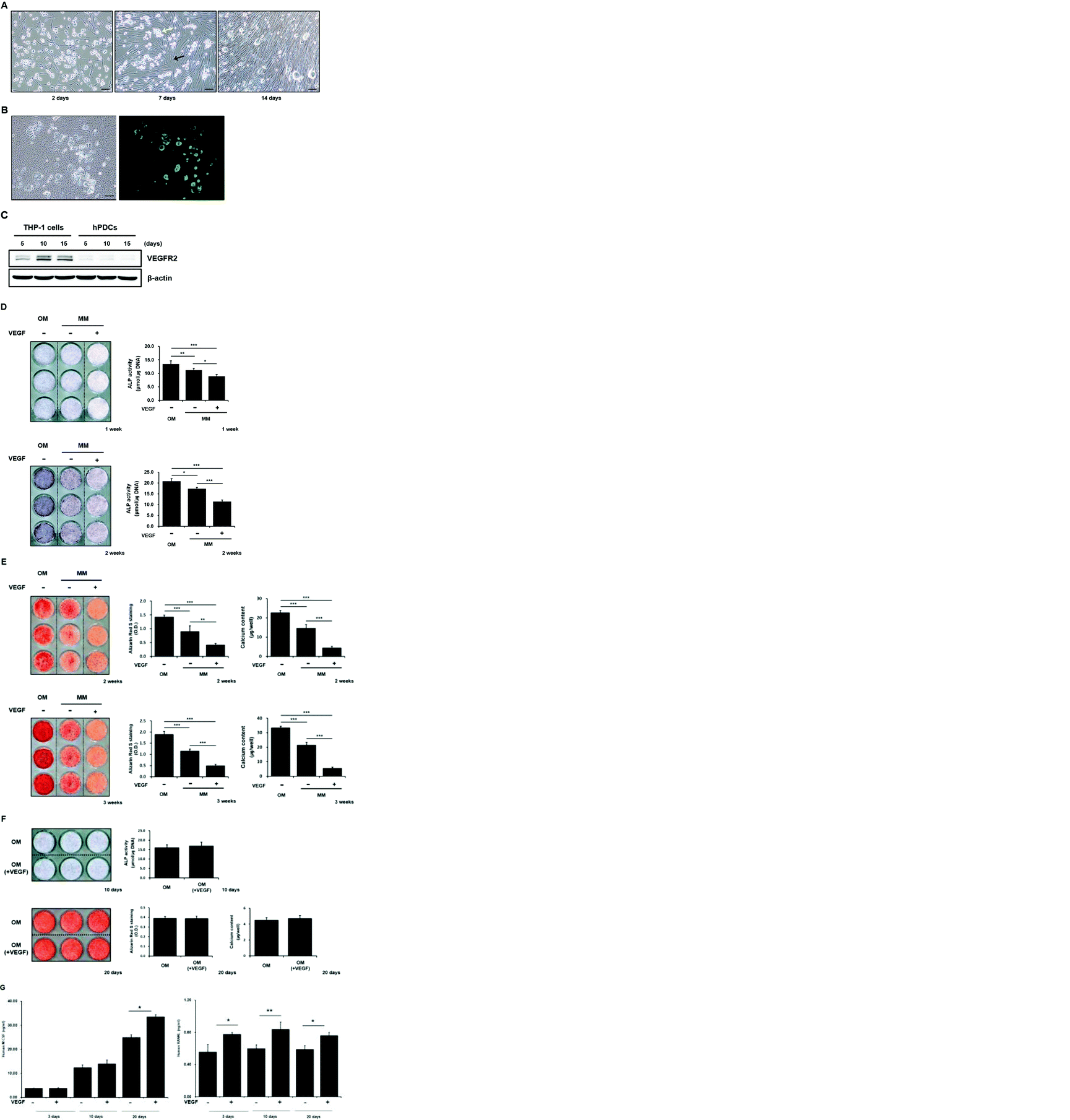 |
| Fig. 7 Effects of VEGF on osteogenic phenotypes in co-cultured hPDCs and THP-1 cells. (A) The direct co-culture of hPDCs and THP-1 cells after 2, 7, and 14 days in culture. (B) Immunofluorescence staining for VEGF binding in co-cultured hPDCs and THP-1 cells. (C) Quantification of VEGFR2 in THP-1 cells and hPDCs. (D and E) Effects of VEGF on the detection and bioactivity of ALP (D), mineralization, and calcium levels (E) in co-cultured hPDCs and THP-1 cells. (F) Effects of VEGF treatment on the detection and bioactivity of ALP, mineralization, and calcium levels during the differentiation of hPDCs into osteoblasts. (G) Protein levels of M-CSF and RANKL in hPDCs treated with and without VEGF after 3, 10, and 20 days in culture. Black arrow, osteoblasts; yellow arrow, multinucleated osteoclasts. *P < 0.05. **P < 0.01. ***P < 0.001. | |
We next examined which cells in the co-culture system had a higher binding affinity for VEGF using immunofluorescence staining. Exogenously added VEGF-A can stimulate the differentiation of osteoblast-like cells, and VEGF secreted from osteoblast-like cells expressing the VEGFR also plays an important role in maintaining bone homeostasis by stimulating the differentiation of MSCs into osteoblasts; however, in the present study, VEGF had a higher affinity for THP-1 cells than hPDCs in the co-culture system (Fig. 7B).52,53,55
We also observed the expression of VEGFR2 in hPDCs cultured in osteogenic induction medium and in THP-1 cells cultured in osteoclast induction medium. Similar to the VEGF affinity data, the expression of VEGFR2 was also clearly observed in THP-1 cells, whereas its expression was nearly undetectable in hPDCs (Fig. 7C). These results indicate that VEGF may play a larger role in osteoclasts than in osteoblasts and have unfavorable effects on bone formation under osteoblast/osteoclast co-culture.
After 1 and 2 weeks in culture, the histochemical detection and bioactivity of ALP were significantly increased in co-cultured cells grown in osteogenic induction medium without VEGF than in cells cultured in an equal mixture of osteogenic induction medium and osteoclast induction medium with or without VEGF. Among the co-culture groups of hPDCs and THP-1 cells cultured in an equal mixture of osteogenic induction medium and osteoclast induction medium, the histochemical detection and activity of ALP was clearly lower in VEGF-treated co-cultured cells than in those without VEGF (Fig. 7D). Similarly, mineralization and calcium levels were also markedly increased in cells co-cultured in osteogenic induction medium without VEGF than in those cultured in an equal mixture of osteogenic induction medium and osteoclastic induction medium with or without VEGF. In addition, VEGF also obviously decreased the quantification of alizarin red-positive mineralization and calcium contents in co-cultured cells cultured an equal mixture of osteogenic induction medium and osteoclastic induction medium (Fig. 7E). Our results suggest that VEGF plays a detrimental role in osteogenic phenotypes in hPDCs and THP-1 cells co-cultured in an equal mixture of osteogenic induction medium and osteoclastic induction medium. In addition, VEGF has a greater influence on THP-1 cells than on hPDCs.
Osteoclastogenesis is a multi-step process that requires the secretion of two cytokines for bone resorption—M-CSF and RANKL—by neighboring stromal/osteoblast-like cells. M-CSF acts via its receptor on osteoclast precursors, leading to the expression of receptor activator of NF-κB (RANK) and the activation of the RANKL/RANK signaling pathway. M-CSF participates in the osteoclastogenic process by promoting the proliferation and survival of osteoclast precursors. RANKL is a type II transmembrane protein that, when released, binds to its receptor RANK, which is expressed on the surfaces of osteoclast precursors and functions as a key regulator for osteoclast differentiation and activation. Therefore, osteoclastogenesis requires osteoclast progenitor commitment, M-CSF-induced osteoclast precursor proliferation, and RANKL-mediated osteoclast differentiation.56–58 Recently, several studies reported that VEGF is an important regulator of osteoclastic bone resorption, including acting as a substitute for M-CSF or RANKL.59–62 However, it is unclear whether the cellular target of VEGF during osteoclastogenesis is within the osteoclast lineage or a stromal/osteoblast-like cell.
In the present study, we examined the role of VEGF as a stimulator of M-CSF and RANKL secretion from hPDCs. In addition, we also evaluated the effects of VEGF on the in vitro differentiation of hPDCs into osteoblasts. As shown in Fig. 3F, VEGF treatment did not have significant effects on ALP, mineralization, or calcium levels in hPDCs (Fig. 7F). Although the main effects of VEGF are on endothelial cells, VEGF can also enhance osteoblast differentiation and mineralization of osteoprogenitor cells expressing VEGFRs. However, there is no direct evidence of the mechanism by which VEGF affects osteoprogenitor cell differentiation. Some studies showed that VEGF alone did not affect the differentiation of MSCs into osteoblasts.63–66 Although further studies will be needed to elucidate the mechanism by which VEGF controls the differentiation of MSCs into osteoblasts, our results suggest that VEGF has no direct effects on the osteoblast activities of hPDCs under in vitro cell culture conditions.
Although VEGF treatment did not affect the protein levels of M-CSF in hPDCs after 3 and 10 days in culture, it significantly increased the secretion of M-CSF from hPDCs after 20 days in culture. However, the protein levels of RANKL were clearly increased in the media collected from hPDCs treated with VEGF during osteoblastic differentiation, regardless of the days in culture (Fig. 7G). These results indicate that the activity of osteoclastogenesis-related genes may be enhanced during the maturation of hPDCs into osteoblasts. In addition, combined with the effects of VEGF on the in vitro differentiation of the THP-1 cells into osteoclasts, our results suggest that VEGF can indirectly enhance osteoclastogenesis in vivo and in co-cultured osteoblasts and osteoclasts in vitro. Moreover, VEGF appears to be a more important factor in osteoclastogenesis than in osteoblastogenesis.
Considering that the osteoblast-osteoclast co-culture system reflects part of the dynamic process of bone formation and resorption that occurs in vivo, these findings show that the biomimetic release of growth factors, including the late release of VEGF from PCL particles treated with three growth factors, are more advantageous to in vivo bone formation than is the sustained release of those growth factors accompanied by the early release of VEGF.
Several signaling pathways by VEGF on THP-1 cells
Several signaling pathways regulate key transcriptional events that mediate osteoclast differentiation. Three mitogen-activated protein kinases (MAPKs), such as extracellular signaling-related kinase (ERK), c-Jun N-terminal kinase (JNK), and p38 MAPK, have been shown to influence osteoblast differentiation and stimulate osteoblast-related gene expression in osteoprecursor cells.67–70 Recently, MAPK pathways have attracted considerable interest due to their important roles in a range of osteoclast activities.70–72 However, evidence regarding the influence of VEGF-mediated stimulation of osteoclast differentiation has not been well characterized. Therefore, in the present study, we examined whether MAPKs were involved in stimulation of osteoclast potential of THP-1 cells mediated by VEGF. As shown in Fig. 4, VEGF treatment of THP-1 cells clearly stimulated the phosphorylation of ERK, JNK, and p38 MAPK. The phosphorylation of JNK and p38 MAPK was distinctly observed in cells treated with VEGF, whereas ERK phosphorylation in VEGF-treated THP-1 cells was less evident in comparison (Fig. 8).
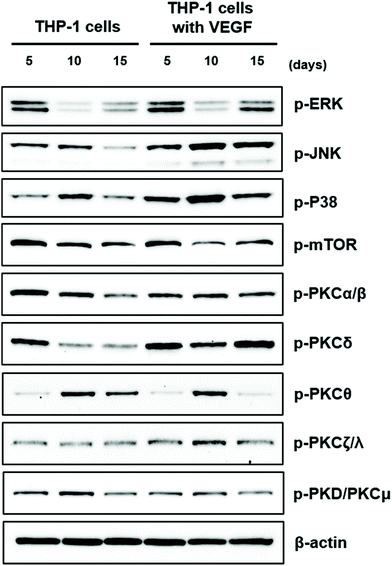 |
| Fig. 8 Effects of VEGF on the activation of signaling pathways in THP-1 cells. The effects of VEGF on the phosphorylation of ERK, JNK, p38 MAPK, mTOR, and the indicated PKC isoforms in THP-1 cells. β-Actin was used as a positive control. | |
The phosphoinositide 3-kinase/protein kinase B/mammalian target of rapamycin (PI3K/AKT/mTOR) signaling pathway plays a role in osteoclast differentiation and survival, including as a determinant of osteoclast size during mTOR signaling. Considering that the expression of VEGFR2 was clearly observed in THP-1 cells in the present study and that VEGF/VEGFR2 exerts its function through the PI3K/AKT/mTOR signaling pathway in several cell types, we examined whether the PI3K/AKT/mTOR pathway is involved in the effects on the differentiation of THP-1 cells into osteoclasts mediated by VEGF.73–75 However, VEGF had no effect on mTOR phosphorylation in THP-1 cells, indicating that PI3K/AKT/mTOR signaling does not affect the activation of differentiation of THP-1 cells into osteoclasts by VEGF (Fig. 8).
Protein kinase C (PKC) represents a family of serine/threonine kinases that can be divided into three subfamilies based on tertiary protein structure and requirements for additional co-factors and calcium: classical (PKCα, PKCβ, and PKCγ), novel (PKCδ, PKCε, PKCη, and PKCθ), and atypical (PKCζ and PKCι/λ). Protein kinase D (PKD, also known as PKCμ) is closely related to the PKC family. PKC isoforms are activated by phosphorylation and phosphorylate protein substrates. PKC-dependent pathways are involved in the expression of osteoclast-specific genes, osteoclast differentiation, and osteoclast function, including the activation of RANKL-mediated phosphorylation of individual PKC isoforms.76–78 However, the involvement of individual PKC isoforms in the regulation of osteoclast function by VEGF is largely unknown. In the present study, the treatment of THP-1 cells with VEGF clearly stimulated the phosphorylation of PKCδ, and the phosphorylation of PKCζ/λ was also enhanced in VEGF-treated THP-1 cells. On the other hand, the phosphorylation of other PKC isoforms, as well as PKD/PKCμ, was not stimulated in cells treated with VEGF.
Several studies have reported the influence of PKCδ on osteoclastogenesis, without considering VEGF in this process.79–81 By inactivating PKCδ specifically in osteoclasts using the Cre/loxP system, Li et al.77 reported that PKCδ deficiency in osteoclasts favors bone mass accumulation in a sex-dependent manner in male mice. The atypical PKC isoforms critically mediate cell survival by activating NF-κB, leading to the activation of osteoclast differentiation-associated genes and the survival of osteoclasts. However, the role of the atypical PKC isoforms in this process is largely unknown, especially with regard to VEGF.
To our knowledge, limited evidence exists regarding the effects of VEGF on either the activation of the differentiation of THP-1 cells into osteoclasts or the co-culture of hPDCs and THP-1 cells. Although the physiological role of MAPK, PKCδ, and PKCζ/λ activation by VEGF during the differentiation of THP-1 cells into osteoclasts has not yet been elucidated, combined with the unfavorable effects of VEGF on the in vitro osteogenesis of co-cultured cells with hPDCs and THP-1 cells, our results suggest that the MAPK, PKCδ, and PKCζ/λ pathways could be involved in the VEGF-mediated reduction of the osteogenesis of co-cultured hPDCs and THP-1 cells.
Conclusions
We developed a growth factor delivery system based on porous particles and a thermosensitive hydrogel that allowed fast (PDGF-BB from MP particles), continuous (BMP-2 from LSS particles), and delayed/continuous (VEGF from pluronic-infiltrated LSS particles) release of growth factors to mimic their biological production during bone regeneration. It was observed that the Continuous group (continuous release of growth factors) provide a better environment for the osteogenic differentiation of hPDCs than the Biomimetic group (biomimetic release of growth factors), and thus anticipate to promote bone regeneration. However, contrary to expectation, the Biomimetic group promoted significant new bone formation compared to the Continuous group. From the additional cell culture experiments, the initial supply of VEGF was considered to have more favorable effects on the osteoclastogenesis than osteogenesis, thus hindering bone regeneration. In addition, our results indicated that the sustained release of VEGF from VEGF-loaded biomaterial might not be conducive to new bone formation. Therefore, we suggest that a biomimetic supply of growth factors is more pivotal parameter for sufficient tissue regeneration. Its use as a molecular delivery system may also serve as a useful tool for the investigation of biological processes and molecules during tissue regeneration processes.
Author contributions
H.Y.K. and J.H.P. conceptualized the project, designed and conducted the experiments, analyzed the data, and wrote the manuscript. M.J.K. helped in performing the experiments and data analysis. J.H.L provided key protocols and intellectual input and helped in designing the experiments. S.H.O. and J.H.B. conceptualized the project, acquired funding, supervised the overall study, and wrote/edited the manuscript.
Conflicts of interest
The authors declare no conflicts of interest.
Acknowledgements
This research was supported by grants from the Basic Science Research Program through the National Research Foundation (NRF) of Korea funded by the Ministry of Education (2015R1A5A2008833, 2018R1D1A1A02085564, and 2020R1A6A1A03043283) and the Bio & Medical Technology Development Program of the National Research Foundation (NRF) funded by the Ministry of Science & ICT (2019M3A9E2066347) and a grant of the Korean Health Technology R&D Project, Ministry of Health & Welfare, Republic of Korea (HI14C1277). This work was also supported by the biomedical research institute fund (GNUHBRIF-2018-0011) from the Gyeongsang National University Hospital. The authors gratefully acknowledge the Center for Bio-Medical Engineering Core Facility at Dankook University for providing equipment.
References
- E. Gibon, L. Lu and S. B. Goodman, Stem Cell Res. Ther., 2016, 7, 44 CrossRef PubMed.
- Y. Li, S. K. Chen, L. Li, L. Qin, X. L. Wang and Y. X. Lai, J. Orthop. Translat., 2015, 3, 95–104 CrossRef PubMed.
- B. Ning, Y. Zhao, J. A. Buza III, W. Li, W. Wang and T. Jia, Mol. Med. Rep., 2017, 15, 1017–1023 CrossRef CAS PubMed.
- W. Wang and K. W. K. Yeung, Bioact. Mater., 2017, 2, 224–247 CrossRef PubMed.
- C. J. Damien and J. R. Parsons, J. Appl. Biomater., 1991, 2, 187–208 CrossRef CAS PubMed.
-
E. R. Carlisle and J. S. Fischgrund, Bone graft and fusion enhancement, in Surgical Management of Spinal Deformities, ed. T. J. Errico, B. S. Lonner and A. W. Moulton, W.B. Saunders, Philadelphia, 2009, pp. 433–448 Search PubMed.
- A. I. P. M. Smits, V. Bonito and M. Stoddart, Tissue Eng., Part A, 2016, 22, 1061–1062 CrossRef PubMed.
- I. K. Ko, S. J. Lee, A. Atala and J. J. Yoo, Exp. Mol. Med., 2013, 45, e57 CrossRef PubMed.
- T. Nakahara, T. Nakamura, E. Kobayashi, M. Inoue, K. Shigeno, Y. Tabata, K. Eto and Y. Shimizu, Tissue Eng., 2003, 9, 153–162 CrossRef CAS PubMed.
- D. E. Discher, D. J. Mooney and P. W. Zandstra, Science, 2009, 324, 1673–1677 CrossRef CAS PubMed.
- Y. H. Kim and Y. Tabata, Adv. Drug Delivery Rev., 2015, 94, 28–40 CrossRef CAS PubMed.
- L. C. Gerstenfeld, D. M. Cullinane, G. L. Barnes, D. T. Graves and T. A. Einhorn, J. Cell. Biochem., 2003, 88, 873–884 CrossRef CAS PubMed.
- P. M. Mountziaris and A. G. Mikos, Tissue Eng., Part B, 2008, 14, 179–186 CrossRef CAS PubMed.
- Z. S. Ai-Aql, A. S. Alagl, D. T. Graves, L. C. Gerstenfeld and T. A. Einhorn, J. Dent. Res., 2008, 87, 107–118 CrossRef CAS PubMed.
- Y. Kimura, N. Miyazaki, N. Hayashi, S. Otsuru, K. Tamai, Y. Kaneda and Y. Tabata, Tissue Eng., Part A, 2010, 16, 1263–1270 CrossRef CAS PubMed.
- J. Fiedler, G. Röderer, K. P. Günther and R. E. Brenner, J. Cell. Biochem., 2002, 87, 305–312 CrossRef CAS PubMed.
- F. B. Basmanav, G. T. Kose and V. Hasirci, Biomaterials, 2008, 29, 4195–4204 CrossRef PubMed.
- D. H. Kempen, L. Lu, A. Heijink, T. E. Hefferan, L. B. Creemers, A. Maran, M. J. Yaszemski and W. J. Dhert, Biomaterials, 2009, 30, 2816–2825 CrossRef CAS PubMed.
- N. J. Shah, M. N. Hyder, M. A. Quadir, N. M. D. Courchesne, H. J. Seeherman, M. Nevins, M. Spector and P. T. Hammond, Proc. Natl. Acad. Sci. U. S. A., 2014, 111, 12847–11252 CrossRef CAS PubMed.
- X. Shen, Y. Zhang, Y. Gu, Y. Xu, Y. Liu, B. Li and L. Chen, Biomaterials, 2016, 106, 205–216 CrossRef CAS PubMed.
- R. Reyes, B. De la Riva, A. Delgado, A. Hernández, E. Sánchez and C. Évora, Injury, 2012, 43, 334–342 CrossRef PubMed.
- M. Dang, L. Saunders, X. Niu, Y. Fan and P. X. Ma, Bone Res., 2018, 6, 25 CrossRef PubMed.
- F. M. Chen, M. Zhang and Z. F. Wu, Biomaterials, 2010, 31, 6279–6308 CrossRef CAS PubMed.
- S. S. Lee, J. H. Kim, J. Jeong, S. H. L. Kim, R. H. Koh, I. Kim, S. Bae, H. Lee and N. S. Hwang, Biomaterials, 2020, 257, 120223 CrossRef CAS PubMed.
- K. J. Kim, M. S. Choi, J. H. Shim and J. W. Rhie, Tissue Eng. Regener. Med., 2019, 16, 395–403 CrossRef CAS PubMed.
- T. W. Kim, W. B. Ahn, J. M. Kim, J. H. Kim, T. H. Kim, R. A. Perez and H. S. Jang, Tissue Eng. Regener. Med., 2020, 17, 607–624 CrossRef CAS PubMed.
- H. Y. Kim, J. H. Lee, H. A. R. Lee, J. S. Park, D. K. Woo, H. C. Lee, G. J. Rho, J. H. Byun and S. H. Oh, ACS Appl. Mater. Interfaces, 2018, 10, 21091–21102 CrossRef CAS PubMed.
- S. H. Oh, J. G. Kang and J. H. Lee, J. Biomed. Mater. Res., Part B, 2018, 106B, 172–182 CrossRef PubMed.
- H. Y. Kim, B. S. An, M. J. Kim, Y. J. Jeoung, J. H. Byun, J. H. Lee and S. H. Oh, ACS Biomater. Sci. Eng., 2020, 6, 2231–2239 CrossRef CAS PubMed.
- Y. H. Shen, M. S. Shoichet and M. Radisic, Acta Biomater., 2008, 4, 477–489 CrossRef CAS PubMed.
- S. V. Boyden, J. Exp. Med., 1962, 115, 453–466 CrossRef CAS PubMed.
- B. W. Park, Y. S. Hah, D. R. Kim, J. R. Kim and J. H. Byun, Arch. Oral Biol., 2007, 52, 983–989 CrossRef CAS PubMed.
- Y. Kubota, H. K. Kleinman, G. R. Martin and T. J. Lawley, J. Cell Biol., 1988, 107, 1589–1598 CrossRef CAS PubMed.
- K. L. DeCicco-Skinner, G. H. Henry, C. Cataisson, T. Tabib, J. C. Gwilliam, N. J. Watson, E. M. Bullwinkle, L. Falkenburg, R. C. O'Neill, A. Morin and J. S. Wiest, J. Visualized Exp., 2014, 91, 51312 Search PubMed.
- H. Y. Kim, J. H. Park, J. H. Byun, J. H. Lee and S. H. Oh, ACS Appl. Mater. Interfaces, 2018, 10, 30115–30124 CrossRef CAS PubMed.
- V. Manickam, A. Tiwari, J. J. Jung, R. Bhattacharya, A. Goel, D. Mukhopadhyay and A. Choudhury, Blood, 2011, 117, 1425–1435 CrossRef CAS PubMed.
- M. Simons, E. Gordon and L. Claesson-Welsh, Nat. Rev. Mol. Cell Biol., 2016, 17, 611–625 CrossRef CAS PubMed.
- I. C. Chuang, C. M. Yang, T. Y. Song, N. C. Yang and M. L. Hu, Life Sci., 2015, 139, 52–61 CrossRef CAS PubMed.
- L. Zheng, Q. Shi, J. Na, N. Liu, Y. Guo and Y. Fan, Cell. Mol. Bioeng., 2019, 12, 85–97 CrossRef PubMed.
- H. Lysdahl, A. Baatrup, C. B. Foldager and C. Bünger, BioRes. Open Access, 2014, 3, 278–285 CrossRef CAS PubMed.
- C. R. Ozawa, A. Banfi, N. L. Glazer, G. Thurston, M. L. Springer, P. E. Kraft, D. M. McDonald and H. M. Blau, J. Clin. Invest., 2004, 113, 516–527 CrossRef CAS PubMed.
- J. Fiedler, G. Roderer, K. P. Gunther and R. E. Brenner, J. Cell. Biochem., 2002, 87, 305–312 CrossRef CAS PubMed.
- W. Zhang, C. Zhu, Y. Wu, E. Ye, S. Wang, D. Zou, X. Zhang, D. L. Kaplan and X. Jiang, Eur. Cells Mater., 2014, 27, 1–11 CrossRef CAS PubMed.
- E. A. Bayer, M. V. Fedorchak and S. R. Little, Tissue Eng., Part A, 2016, 22, 1296–1304 CrossRef CAS PubMed.
- X. Wang, B. G. Matthews, J. Yu, S. Novak, D. Grcevic, A. Sanjay and I. Kalajzic, JBMR Plus, 2019, 3, e10127 CrossRef PubMed.
- T. Wang, S. Guo and H. Zhang, BioMed. Res. Int., 2018, 2018, 3516463 Search PubMed.
- Q. Wang, Y. Zhang, B. Li and L. Chen, J. Mater. Chem. B, 2017, 5, 6963–6972 RSC.
- R. S. Hayden, K. P. Quinn, C. A. Alonzo, I. Georgakoudi and D. L. Kaplan, Biomaterials, 2014, 12, 3794–3802 CrossRef PubMed.
- Z. H. Li, Y. Si, G. Xu, X. M. Chen, H. Xiong, L. Lai, Y. Q. Zheng and Z. G. Zhang, Mol. Med. Rep., 2017, 16, 8380–8384 CrossRef CAS PubMed.
- Y. Zhang, C. Ma, C. Liu and W. Wu, Life Sci., 2020, 258, 118093 CrossRef CAS PubMed.
- R. Scian, P. Barrionuevo, A. M. Rodriguez, P. C. Arriola Benitez, C. García Samartino, C. A. Fossati, G. H. Giambartolomei and M. V. Delpino, Infect. Immun., 2013, 81, 1940–1951 CrossRef CAS PubMed.
- Y. Liu, A. D. Berendsen, S. Jia, S. Lotinun, R. Baron, N. Ferrara and B. R. Olsen, J. Clin. Invest., 2012, 122, 3101–3113 CrossRef CAS PubMed.
- K. Hu and B. R. Olsen, Bone, 2016, 91, 30–38 CrossRef CAS PubMed.
- A. Grosso, M. G. Burger, A. Lunger, D. J. Schaefer, A. Banfi and N. Di Maggio, Front. Bioeng. Biotechnol., 2017, 5, 68 CrossRef PubMed.
- K. Hu and B. R. Olsen, J. Clin. Invest., 2016, 126, 509–526 CrossRef PubMed.
- X. Feng and S. L. Teitelbaum, Bone Res., 2013, 1, 11–26 CrossRef PubMed.
- G. Luo, F. Li, X. Li, Z. G. Wang and B. Zhang, Mol. Med. Rep., 2018, 17, 6605–6611 CAS.
- D. Abdallah, M. L. Jourdain, J. Braux, C. Guillaume, S. C. Gangloff, J. Jacquot and F. Velard, Front. Immunol., 2018, 9, 632 CrossRef PubMed.
- R. M. Taylor, T. G. Kashima, H. J. Knowles and N. A. Athanasou, Lab. Invest., 2012, 92, 1398–1406 CrossRef CAS PubMed.
- H. R. Kim, K. W. Kim, B. M. Kim, M. L. Cho and S. H. Lee, PLoS One, 2015, 10, e0124909 CrossRef PubMed.
- Q. Yang, K. P. McHugh, S. Patntirapong, X. Gu, L. Wunderlich and P. V. Hauschka, Matrix Biol., 2008, 27, 589–599 CrossRef CAS PubMed.
- H. Kitaura, A. Marahleh, F. Ohori, T. Noguchi, W. R. Shen, J. Qi, Y. Nara, A. Pramusita, R. Kinjo and I. Mizoguchi, Int. J. Mol. Sci., 2020, 21, 5169 CrossRef CAS PubMed.
- W. Lu, W. Xu, J. Li, Y. Chen, Y. Pan and B. Wu, Mol. Med. Rep., 2019, 20, 3924–3932 CAS.
- Y. Liu and B. R. Olsen, Arch. Immunol. Ther. Exp., 2014, 62, 363–368 CrossRef CAS PubMed.
- C. J. Pi, K. L. Liang, Z. Y. Ke, F. Chen, Y. Cheng, L. J. Yin, Z. L. Deng, B. C. He and L. Chen, Biol. Chem., 2016, 397, 765–775 CAS.
- M. K. Kim, S. G. Kim and S. K. Lee, Maxillofac. Plast. Reconstr. Surg., 2020, 42, 23 CrossRef PubMed.
- E. Rodríguez-Carballo, B. Gámez and F. Ventura, Front. Cell Dev. Biol., 2016, 4, 40 Search PubMed.
- M. B. Greenblatt, J. H. Shim and L. H. Glimcher, Annu. Rev. Cell Dev. Biol., 2013, 29, 63–79 CrossRef CAS PubMed.
- B. S. Kim, H. J. Kang, J. Y. Park and J. Lee, Exp. Mol. Med., 2015, 47, e128 CrossRef CAS PubMed.
- K. Lee, I. Seo, M. H. Choi and D. Jeong, Int. J. Mol. Sci., 2018, 19, 3004 CrossRef PubMed.
- K. Lee, Y. H. Chung, H. Ahn, H. Kim, J. Rho and D. Jeong, Int. J. Biol. Sci., 2016, 12, 235–245 CrossRef CAS PubMed.
- Y. Fu, J. Gu, Y. Wang, Y. Yuan, X. Liu, J. Bian and Z. Liu, Mol. Med. Rep., 2015, 12, 6939–6945 CrossRef CAS PubMed.
- J. B. Moon, J. H. Kim, K. Kim, B. U. Youn, A. Ko, S. Y. Lee and N. Kim, J. Immunol., 2012, 188, 163–169 CrossRef CAS PubMed.
- A. Irelli, M. M. Sirufo, T. Scipioni, F. De Pietro, A. Pancotti, L. Ginaldi and M. De Martinis, Int. J. Mol. Sci., 2019, 20, 5841 CrossRef CAS PubMed.
- X. B. Trinh, W. A. Tjalma, P. B. Vermeulen, G. Van den Eynden, I. Van der Auwera, S. J. Van Laere, J. Helleman, E. M. Berns, L. Y. Dirix and P. A. van Dam, Br. J. Cancer, 2009, 100, 971–978 CrossRef CAS PubMed.
- J. Shin, H. Jang, J. Lin and S. Y. Lee, Mol. Cells, 2014, 37, 747–752 CrossRef PubMed.
- S. Li, T. He, D. Wu, L. Zhang, R. Chen, B. Liu, J. Yuan, J. Tickner, A. Qin, J. Xu and L. Rong, Front. Cell Dev. Biol., 2020, 8, 450 CrossRef PubMed.
- A. C. Leightner, C. Mello Guimaraes Meyers, M. D. Evans, K. C. Mansky, R. Gopalakrishnan and E. D. Jensen, Int. J. Mol. Sci., 2020, 21, 1056 CrossRef CAS PubMed.
- M. Y. Kim, K. Lee, H. I. Shin and D. Jeong, Sci. Rep., 2019, 9, 7044 CrossRef PubMed.
- V. Cremasco, C. E. Decker, D. Stumpo, P. J. Blackshear, K. I. Nakayama, K. Nakayama, T. S. Lupu, D. B. Graham, D. V. Novack and R. Faccio, J. Bone Miner. Res., 2012, 27, 2452–2463 CrossRef CAS PubMed.
- T. Oikawa, Y. Kuroda and K. Matsuo, Cell. Mol. Life Sci., 2013, 70, 3341–3353 CrossRef CAS PubMed.
Footnotes |
† Electronic supplementary information (ESI) available: SEM photographs; FTIR spectra; release behaviors of growth factors (PDF). See DOI: 10.1039/d1bm00245g |
‡ These authors contributed equally to this work. |
|
This journal is © The Royal Society of Chemistry 2021 |