DOI:
10.1039/D0AY01996H
(Paper)
Anal. Methods, 2021,
13, 117-123
Highly sensitive electrochemical determination of methotrexate based on a N-doped hollow nanocarbon sphere modified electrode
Received
27th October 2020
, Accepted 26th November 2020
First published on 10th December 2020
Abstract
As an antineoplastic drug, methotrexate (MTX) is widely applied in cancer therapies; however it has potentially toxic activity, and thus the qualitative and quantitative determination of MTX is of great significance. In this paper, by using dopamine synchronously as a carbon and N source, N-doped hollow nanocarbon sphere (NHNC) hybrids were prepared via a simple self-polymerization method and used to construct a new electrochemical sensor for MTX. The prepared NHNC exhibits a uniform hollow nanostructure with high conductivity and electrocatalytic properties as well as large adsorption capacity and surface area, which are the key factors for improving the sensor sensitivity of MTX. For achieving highly sensitive determination of MTX, various conditions were optimized, and the final results show that the NHNC modified electrode has excellent sensing responses for MTX, and it has a wide linear range from 0.05 to 14.0 μM coupled with a low detection limit of 0.01 μM. Finally, studies on the reproducibility, stability and selectivity of the NHNC modified electrode show that the corresponding results are satisfactory.
1. Introduction
Methotrexate (MTX), named also as amethopterin, is an effective anti-metabolite drug and is used widely in treating various cancers such as leukemia, neck and head, breast, lymphoma and osteogenic sarcoma; meanwhile, MTX is applied extensively for autoimmune diseases (e.g. psoriasis, rheumatoid arthritis, and lupus) as an immunosuppressive and anti-inflammatory drug.1–4 However, the clinical use of MTX is generally limited owing to the severe side-effects since it can cause ulcerative stomatitis, liver damage, and low white blood cell count, and the application of high-dose MTX can even be life threatening.5–7 Therefore, monitoring the MTX concentration in blood is very beneficial for obtaining an optimized drug dosing which exhibits minimum toxicity and maximum treatment effects.
In the past, high performance liquid chromatography,8 UV-vis spectrophotometry,9 electrospray ionization tandem mass spectrometry,10 capillary electrophoresis11 and fluorimetry12 have been used widely for monitoring MTX. Although the requirements of sensitivity, selectivity, and accuracy could be met via the above methods, they generally require complex sample preparation and operation, a large amount of organic solvent, high cost and long time. Recently, the electrochemical method has received attention and several related proposals have been developed for detecting MTX owing to its unique advantages such as simplicity, ease of field applications, sensitivity and accuracy.13,14 For instance, Foroughi et al.15 prepared cerium-doped ZnO nanoflowers to obtain an electrochemical sensor for MTX; the results showed that the nanoflower modified electrode has a low detection of 6.3 nM; in another study, Fathi et al.16 fabricated a CuCr2O4/CuO nanofiber carbon paste electrode in the presence of IL for electrochemically sensing MTX, and a linear range of 0.1–300.0 μM and detection limit of 0.025 μM were obtained successfully. No doubt these methods are of great significance, but the electrochemical method with high sensitivity and quick response times as well as low cost is still a major challenge for MTX analysis.
In recent years, much attention has been given to nanohollow structures owing to their intriguing properties including high shell permeability and surface-to-volume ratio as well as low density and important potential applications in many areas.17,18 In the families of nanohollow structures, the hollow nanocarbon nanosphere (HNC) is particularly interesting since it can exhibit many unique advantages, such as wide sources, low capital cost, strong chemical stability, high conductivity and electrocatalytic properties,19–21 which enable HNC to be a desirable potential candidate for constructing an effective electrochemical sensor. So far, HNC has been applied largely in ion batteries,22 energy storage,23 LiS battery,24 catalysis,25,26 supercapacitors,27 oxygen reduction,28etc.,29,30 while there are only a few studies focused on electrochemical sensors.31 Especially, there is no work reported for electrochemical monitoring of antineoplastic drugs using HNC.
In addition, the doping strategy has sparked fresh interest and offered new prospects in electrochemistry,32–34 and heteroatom-doped nanocarbon materials have made significant progress in improving their performance (e.g. increasing edge-plane defect and catalytically active sites as well as the electron transfer rate) and showing broad prospects for application.35,36 Hence, herein, N-doped HNC (NHNC) hybrids were prepared firstly via a simple and green method, and then NHNC was used to modify a glassy carbon electrode for constructing an electrochemical sensor (NHNC/GCE) for antineoplastic drug MTX (Scheme 1). The results show that NHNC/GCE exhibits prominent sensing performance for MTX: rapid and highly sensitive electrochemical determination of MTX was successfully achieved owing to the unique properties from NHNC (high conductivity and electrocatalytic properties as well as large surface area) and strong adsorption capacity towards MTX molecules, thus revealing that the developed method based on NHNC has important applications for MTX determination.
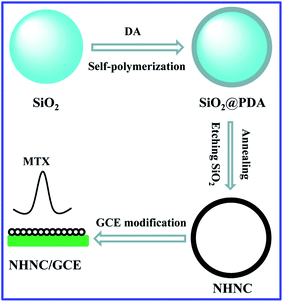 |
| Scheme 1 The procedures for preparing NHNC/GCE and electrochemically sensing MTX. | |
2. Experimental section
2.1. Preparation of NHNC hybrids
The NHNC hybrids were prepared through a typical self-aggregating process of dopamine (DA) with minor modification.37 In brief, the purchased SiO2 nanoparticles were added to Tris buffer containing DA and the DA molecules were allowed to aggregate on the SiO2 surface with stirring for one day; thus SiO2/polydopamine (SiO2/PDA) composites were formed. After being centrifuged/washed/dried, the obtained SiO2/PDA was annealed in nitrogen flow for two hours at high temperature (800 °C), and then through etching the template SiO2 in HF solution, NHNC was obtained successfully.
2.2. Constructing the electrochemical sensor for MTX
The GCE surface was polished carefully with 0.5 and 0.05 μm alumina, and cleaned with ultrapure water, followed by cleaned ultrasonically in acetone and ultra-pure water, respectively. 3.0 mg NHNC was dispersed in 6.0 mL ultrapure water and sonicated to form a homogeneous suspension. NHNC/GCE was constructed via dropping different amounts of NHNC suspension on the GCE surface. The electrochemical measurement of MTX by using NHNC/GCE was carried out following 2 consecutive steps: (1) pre-concentration of MTX on the NHNC/GCE surface; and (2) measurement through the corresponding electrochemical technology, which was performed on a CHI 660E electrochemical workstation (Chenhua Instrument Company, Shanghai, China), and a common three-electrode system was introduced with a modified electrode as a working electrode, a Ag/AgCl electrode as a reference electrode, and platinum wire as a counter electrode.
3. Results and discussion
3.1. Characterization of NHNC hybrids
The nanostructures and morphologies of NHNC were investigated firstly by scanning-/transmission-electron microscopies (SEM and TEM). As shown in Fig. 1A, SiO2 nanoparticles have a uniform diameter of about 350 nm and their surfaces are considerably smooth. When PDA was coated on the SiO2 surface via the self-aggregation (Scheme 2) of DA to produce SiO2/PDA (Fig. 1B), its diameter increased to about 370 nm, revealing that the PDA shell thickness is ∼20 nm. After annealing SiO2/PDA at high temperature and removing template SiO2 with HF solution, the SEM image of NHNC exhibits a typical hollow structure (Fig. 1C). TEM was further used to investigate the morphologies of NHNC, and as depicted Fig. 1D, uniform and hollow nanospheres were present, which is consistent with the SEM image, indicating the successful preparation of the NHNC nanohybrids.
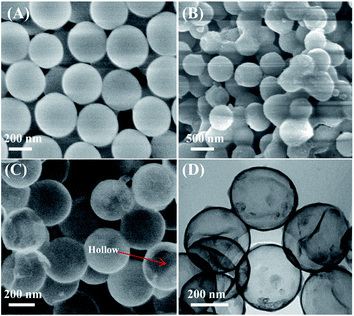 |
| Fig. 1 SEM images of SiO2 (A), SiO2/PDA (B) and NHNC (C). TEM image of NHNC (D). | |
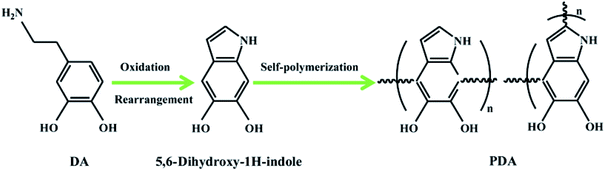 |
| Scheme 2 The self-aggregation processes of DA to form PDA. | |
Next, the prepared NHNC was used to modify a GCE to obtain NHNC/GCE, and its electron transfer behavior was evaluated by electrochemical impedance analysis (EIS, Fig. 2), which was measured in an aqueous solutions containing Fe(CN)63−/4− (5.0 mM) and KCl (0.1 M). It's noted from the figure that the bare GCE shows a large fitted charge transfer resistance (Rct) value (∼220.0 Ω); as for NHNC/GCE, the corresponding Rct value is much lower than that of the bare GCE and it can even almost be neglected, suggesting that the NHNC modified electrode (NHNC/GCE) has higher electrical conductivity than the GCE, which is very beneficial for improving the sensitivity of the electrochemical sensor. Furthermore, the surface areas of the modified electrodes were calculated with cyclic voltammetry (CV) in [Fe(CN)6]3− solution according to the Randles–Sevcik equation: Ip = 2.69 × 105An3/2D1/2Cv1/2, where Ip refers to the peak current and A is the surface area (cm2). For [Fe(CN)6]3−, n = 1, D = 7.6 × 10−6 cm2 s−1, C is the concentration of [Fe(CN)6]3−, and v is the scan rate. The results show that the surface area of NHNC/GCE (∼0.86 cm2) is ∼12.3 times higher than that of the bare GCE (∼0.070 cm2), respectively, suggesting that the NHNC modified GCE can remarkably increase the surface area. As for the capacitance of NHNC/GCE, it was obtained by studying the galvanostatic charge–discharge curves of NHNC/GCE at varying current densities and calculated according to C = It/mΔV, where C (F g−1), I (A), t (s), m (g) and ΔV (V) are the capacitance, discharge current, discharge time, the mass of NHNC, and potential window, respectively. The results show that the capacitances of the modified electrode at current densities of 1, 2, 3, 4 and 5 A g−1 were calculated to be 142.5, 137.3, 132.4, 129.5 and 126.7 F g−1, respectively. It's noted that 88.9% capacitance was retained when the current density increased from 1 to 5 A g−1, demonstrating that the NHNC nanohybrids possess good charge–discharge performance.
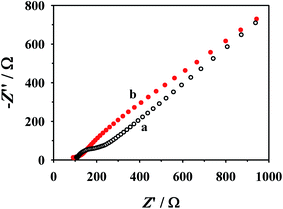 |
| Fig. 2 EIS plots of the bare GCE (a) and NHNC/GCE (b) in Fe(CN)63−/4− and KCl aqueous solutions. | |
3.2. Electrochemical behavior of MTX at NHNC/GCE
MTX is an electroactive substance which could be oxidized electrochemically on the electrode surface and the related oxidation mechanism is shown in Fig. 3. The electrochemical behaviors of 50.0 μM MTX at different electrodes including the NHNC/GCE and bare GCE were investigated using CV and differential pulse voltammetry (DPV) techniques in 0.1 M PBS (pH 6.0). As can be seen from Fig. 4A, the bare GCE merely shows a very broad and weak anodic peak of MTX at ∼0.74 V since the electron transfer and sensing performance of the bare GCE are sluggish, indicating that the pure GCE is not desirable for the highly sensitive determination of MTX. However, as for the electrochemical response of MTX at NHNC/GCE, the oxidation peak current dramatically increases accompanied by an obvious negative shift of the peak potential owing to the high conductivity and electrocatalytic activity as well as large adsorption capacity and surface area. Specifically, the current value of MTX at NHNC/GCE is ∼25.3 times higher than that at the GCE, and the oxidation peak potential was shifted to ∼0.69 V. Meanwhile, as for the successive CV scans, it's found that the oxidation current of MTX would decrease from the first scan to the second scan due to the adsorption species formed on the NHNC/GCE surface owing to the strong adsorption ability of NHNC toward MTX. In addition, the DPV results of MTX with low concentration (4.0 μM) at the NHNC/GCE and bare GCE were also studied (Fig. 4B); it can be seen that the DPV current of MTX at NHNC/GCE is much higher than that at the GCE, and these results are similar to those obtained via CV, suggesting that NHNC/GCE has prominent sensing capabilities toward the determination of MTX.
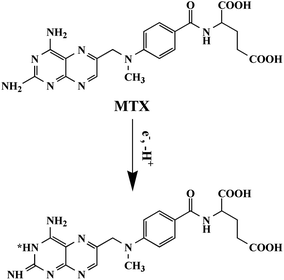 |
| Fig. 3 The electrochemical oxidation mechanism of MTX. | |
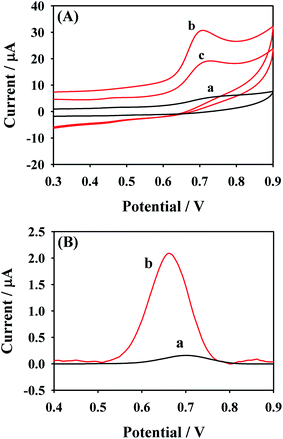 |
| Fig. 4 (A) CV responses of MTX at the bare GCE (a) and NHNC/GCE with the first (b) and second scans (c) in 0.1 M PBS. (B) The DPV responses of MTX with low concentration (4.0 μM) at the bare GCE (a) and NHNC/GCE (b). | |
3.3. Optimization of the conditions
As a powerful electrochemical technology, DPV has higher sensitivity and resolution compared to CV technology. For achieving the maximum sensitivity of MTX determination, some related test conditions were optimized by DPV. The influence from the accumulation time toward the peak current response of MTX was firstly investigated. Under stirring, NHNC/GCE was soaked into 0.1 M PBS containing 7.0 μM MTX for a certain period of time to allow the full accumulation of MTX molecules on the NHNC/GCE surface. As exhibited clearly in Fig. 5A, taking a simple accumulation process even with a very short time shows a significant effect on the electrochemical response of MTX. It can be noted that the peak current dramatically increases with increasing accumulation time from 10 to 80 s, while further increasing the time to 140 s has no obvious effect on the MTX peak current. Therefore, 80 s is set as the optimum accumulation time.
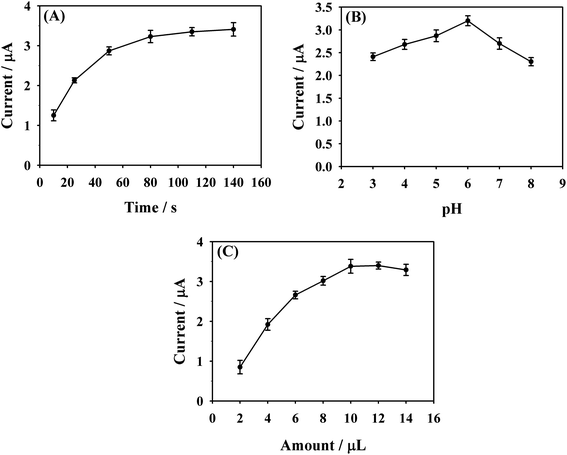 |
| Fig. 5 The effect of accumulation time (A), pH value of PBS (B) and amount of NHNC (C) on the DPV currents of 7.0 μM MTX at NHNC/GCE in 0.1 M PBS. | |
The pH values of PBS have an obvious effect on the peak current of MTX. To study the influence of PBS with different pH on the electrochemical response of MTX at NHNC/GCE, the DPV current of 7.0 μM MTX was recorded in the pH range of 3.0–8.0 (Fig. 5B). Upon increasing pH from 3.0 to 6.0, the oxidation current of MTX increases gradually and achieves its maximum value at pH of 6.0. A further rise of pH to 8.0 makes the peak current decrease. Thus, PBS with a pH of 6.0 was chosen and used for all further experiments. It's no doubt that the sensing sensitivity is affected by the NHNC amount on the electrode surface; thus the influence of NHNC amount was also investigated. As shown in Fig. 5C, it's noted that the oxidation peak current of MTX increases with the increase of NHNC amount from 2.0 to 14.0 μL and the maximum current was obtained at 10.0 μL NHNC suspension; hence this amount (10.0 μL) was applied throughout the work unless otherwise stated.
3.4. Analytical performance
Under the above optimal experimental parameters, the analytical performance of NHNC/GCE toward MTX was evaluated through plotting the related calibration curve containing the changes in DPV currents with the increase of MTX concentration; it's found that the current value of MTX linearly increases with MTX concentrations ranging from 0.05 to 14.0 μM (Fig. 6). The related linear regression equation is defined as Ip (μA) = 0.2543 + 0.4473C (μM) coupled with the correlation coefficient of R2 = 0.9984, and the limit of detection (LOD) is calculated to be 0.01 μM (S/N = 3), which are better than those reported in many previous studies (Table 1), revealing that NHNC/GCE could be used in the highly sensitive determination of MTX. Meanwhile, the preparation method for the NHNC sensor in this work is very low cost and simple as well as eco-friendly.
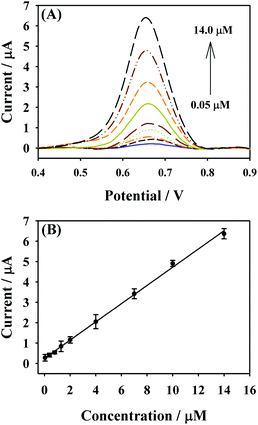 |
| Fig. 6 (A) DPV responses of MTX with different concentrations at NHNC/GCE in 0.1 M PBS. (B) The calibration curve for MTX detection. | |
Table 1 Comparison of different modified electrodes for the electrochemical determination of MTX
Materials used |
Linear range [μM] |
LOD [μM] |
Reference |
Ce–ZnO/GCE |
0.01–500.0 |
0.0063 |
15
|
Biopolymers blend films/indium tin oxide |
1.5–50.0 |
0.595 |
38
|
CoFe2O4/reduced graphene oxide/ionic liquid/GCE |
0.1–7.5 |
0.02 |
39
|
Carbon black/copper nanoparticles/Nafion/GCE |
2.2–25.0 |
0.09 |
40
|
Porous graphene–carbon nanotube/GCE |
0.7–10.0 |
0.07 |
41
|
Surfactant modified carbon nanotube paste electrode |
0.2–7.0 |
0.035 |
42
|
NHNC/GCE |
0.05–14.0 |
0.01 |
This work |
3.5. Reproducibility, stability and selectivity
For studying the reproducibility of NHNC/GCE, which is expressed via the relative standard deviation (RSD), eight parallelly made NHNC/GCEs were used to measure 10.0 μM MTX by DPV. The RSD value of its peak current response was found to be 4.59% (Fig. 7A). On the other hand, the long-term stability of NHNC/GCE was studied via monitoring the current response of MTX for four weeks with intervals of four days; the results show that little decrease of the peak current is seen and the decrease in value is below 3.0% (Fig. 7B). These results demonstrated that NHNC/GCE has an acceptable reproducibility and storage stability. The selectivity of NHNC/GCE for MTX was evaluated through comparing current responses in the presence of potential interfering organic molecules. The results revealed that no evident interference was presented for the following molecules including ascorbic acid (AA), uric acid (UA), folinic acid (FA), tetrahydrofolic acid (TA), pyridoxine (Py), 5-methyltetrahydrofolate (MH) and dopamine (DA) (10.0 times the concentration of MTX, Fig. 7C), suggesting that NHNC/GCE possesses a satisfactory selectivity in MTX determination.
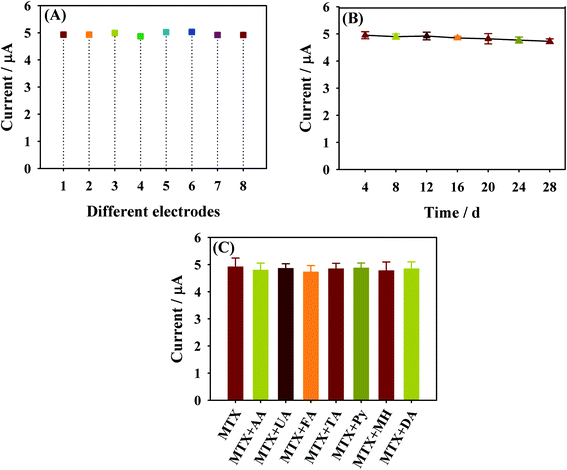 |
| Fig. 7 The DPV peak current value of MXT at independently fabricated NHNC/GCE (A), after different storage times (B) and containing different interferents (C). | |
3.6. Determination of MTX in human blood serum
For verifying the reliability of the developed method in the determination of MTX in real samples, the NHNC/GCE performance was studied in human blood serum. Specifically, the sample was centrifuged, filtered and diluted 10 times with 0.1 M PBS without any further treatment. The diluted serum sample was spiked with different amounts of MTX and the corresponding current responses were recorded using NHNC/GCE (Table 2). From Table 2, it is noted that recoveries of 94.5–96.3% can be obtained, revealing that this method is satisfactory.
Table 2 The determination of MTX based on NHNC/GCE in blood serum samples
Samples |
Added [μM] |
Found [μM] |
Recovery [%] |
a |
2.0 |
1.89 |
94.5 |
b |
5.0 |
4.82 |
96.4 |
c |
10.0 |
9.63 |
96.3 |
4. Conclusion
In summary, NHNC nanohybrids with high conductivity and electrocatalysis as well as strong adsorption capability toward MTX were prepared via a simple method and used to construct a new electrochemical sensor for MTX. By optimizing various experimental conditions, the fabricated NHNC/GCE exhibits prominent electrochemical sensing performances toward MTX analyte with a low LOD (0.01 μM) and wide linear range (0.05–14.0 μM). Meanwhile, NHNC/GCE shows high reproducibility and stability as well as good selectivity. It's confirmed that the proposed method for MTX detection on the basis of NHNC hybrids has important potential applications for MTX analysis.
Conflicts of interest
There are no conflicts to declare.
Acknowledgements
This work was supported by the Project of Innovation Platform for Fuzhou Health and Family Planning Commission (No. 2017-S-wp1 and 2018-S-wp3), the Key Clinical Specialty Discipline Construction Program of Fujian Province, the Major Science and Technology Projects of Fuzhou (2017), and the Funds for Young and Middle-aged Experts with Outstanding Contributions to Health and Family Planning in Fujian Province (2017–2018).
References
- M. Sajith, A. Pawar, N. Vaidya, K. Subramanian, V. Bafna and S. Bartakke, Anal. Chem. Lett., 2018, 8, 503–509 CrossRef CAS.
- N. Salandarijolge, A. A. Ensafi and B. Rezaei, Anal. Bioanal. Chem., 2020, 1–11 Search PubMed.
- S. Kummari, V. S. Kumar, M. Satyanarayana and K. V. Gobi, Microchem. J., 2019, 148, 626–633 CrossRef CAS.
- P. Wang, Q. Wu, C. Wang, Y. Pu, M. Zhou and M. Zhang, J. Electrochem. Soc., 2020, 167, 107505 CrossRef CAS.
- S. Phal, B. Lindholm-Sethson, P. Geladi, A. Shchukarev and S. Tesfalidet, Anal. Chim. Acta, 2017, 987, 15–24 CrossRef CAS PubMed.
- W. A. El-Said, M. A. Abdel-Rahman, E. M. Sayed and A.-M. A. Abdel-Wahab, Electroanalysis, 2019, 31, 829–837 CrossRef CAS.
- L. Janikovabandžuchova and R. Selesovska, Anal. Lett., 2016, 49, 122–134 CrossRef.
- X. Liu, J. Liu, Y. Huang, R. Zhao, G. Liu and Y. Chen, J. Chromatogr. A, 2009, 1216, 7533–7538 CrossRef CAS PubMed.
- A. R. De Oliveira, L. B. De Caland, E. G. De Oliveira, E. S. T. D. Egito, M. D. F. F. Pedrosa and A. A. D. S. Junior, J. Braz. Chem. Soc., 2015, 26, 649–659 Search PubMed.
- R. Schofield, L. V. Ramanathan, K. Murata, M. Fleisher, M. S. Pessin and D. Carlow, Methods Mol. Biol., 2016, 1383, 213–222 CrossRef CAS PubMed.
- C. Kuo, H. Wu, H. Kou, S. Chiou, D. Wu and S. Wu, J. Chromatogr. A, 2003, 1014, 93–101 CrossRef CAS PubMed.
- F. Meng, F. Gan and G. Ye, Mikrochim. Acta, 2019, 186, 371 CrossRef PubMed.
- F. Karami, S. Ranjbar, Y. Ghasemi and M. Negahdaripour, J. Pharm. Anal., 2019, 9, 373–391 CrossRef PubMed.
- M. Mollaei, S. M. Ghoreishi and A. Khoobi, Measurement, 2020, 152, 107362 CrossRef.
- N. Jandaghi, S. Jahani, M. M. Foroughi, M. Kazemipour and M. Ansari, Microchim. Acta, 2020, 187, 24 CrossRef CAS PubMed.
- Z. Fathi, S. Jahani, M. S. Zandi and M. M. Foroughi, Anal. Bioanal. Chem., 2020, 412, 1011–1024 CrossRef CAS PubMed.
- C. Guan, A. Sumboja, H. Wu, W. Ren, X. Liu, H. Zhang, Z. Liu, C. Cheng, S. J. Pennycook and J. Wang, Adv. Mater., 2017, 29, 1704117 CrossRef PubMed.
- Y. Yi, G. Zhu, H. Sun, J. Sun and X. Wu, Biosens. Bioelectron., 2016, 86, 62–67 CrossRef CAS PubMed.
- J. Liu, N. P. Wickramaratne, S. Z. Qiao and M. Jaroniec, Nat. Mater., 2015, 14, 763–774 CrossRef CAS PubMed.
- Q. Xu, F. Yan, J. Lei, C. Leng and H. Ju, Chem.–Eur. J., 2012, 18, 4994–4998 CrossRef CAS PubMed.
- H. Dong, Z. Zhu, H. Ju and F. Yan, Biosens. Bioelectron., 2012, 33, 228–232 CrossRef CAS PubMed.
- C. Lu, G. Pan, Z. Mao, L. Shi, Q. Huang, W. Tian, Y. Hu, H. Wu, Z. Wang and K. Sun, J. Mater. Chem. A, 2020, 8, 8875–8882 RSC.
- J. Du, A. Chen, L. Liu, B. Li and Y. Zhang, Carbon, 2020, 160, 265–272 CrossRef CAS.
- Q. Qi, H. Zhang, P. Zhang, Z. Bao, W. Zheng, W. Tian, W. Zhang, M. Zhou and Z. Sun, 2D Materials, 2020, 7, 025049 CrossRef CAS.
- D.-G. Lee, S. M. Kim, H. Jeong, J. Kim and I. S. Lee, ACS Nano, 2014, 8, 4510–4521 CrossRef CAS PubMed.
- R. Liu, S. M. Mahurin, C. Li, R. R. Unocic, J. C. Idrobo, H. Gao, S. J. Pennycook and S. Dai, Angew. Chem., Int. Ed., 2011, 50, 6799–6802 CrossRef CAS PubMed.
- X. Fang, J. Zang, X. Wang, M.-S. Zheng and N. Zheng, J. Mater. Chem. A, 2014, 2, 6191–6197 RSC.
- Y. Pang, K. Wang, H. Xie, Y. Sun, M.-M. Titirici and G.-L. Chai, ACS Catal., 2020, 10, 7434–7442 CrossRef CAS.
- Y. Liu, K. Ai and L. Lu, Chem. Rev., 2014, 114, 5057–5115 CrossRef CAS PubMed.
- G. Cheng, M.-D. Zhou and S.-Y. Zheng, ACS Appl. Mater. Interfaces, 2014, 6, 12719–12728 CrossRef CAS PubMed.
- R. Zhang, R. Jamal, Y. Ge, W. Zhang, Z. Yu, Y. Yan, Y. Liu and T. Abdiryim, Carbon, 2020, 161, 842–855 CrossRef CAS.
- X. Wang, X. Li, L. Zhang, Y. Yoon, P. K. Weber, H. Wang, J. Guo and H. Dai, Science, 2009, 324, 768–771 CrossRef CAS PubMed.
- P. Chen, T. Zhou, L. Xing, K. Xu, Y. Tong, H. Xie, L. Zhang, W. Yan, W. Chu, C. Wu and Y. Xie, Angew. Chem., Int. Ed., 2017, 56, 610–614 CrossRef CAS PubMed.
- X. Han, X. Ling, D. Yu, D. Xie, L. Li, S. Peng, C. Zhong, N. Zhao, Y. Deng and W. Hu, Adv. Mater., 2019, 31, 1905622 CrossRef CAS PubMed.
- H. Fei, J. Dong, C. Wan, Z. Zhao, X. Xu, Z. Lin, Y. Wang, H. Liu, K. Zang, J. Luo, S. Zhao, W. Hu, W. Yan, I. Shakir, Y. Huang and X. Duan, Adv. Mater., 2018, 30, 1802146 CrossRef PubMed.
- K. Jiang, S. Siahrostami, T. Zheng, Y. Hu, S. Hwang, E. Stavitski, Y. Peng, J. Dynes, M. Gangisetty, D. Su, K. Attenkofer and H. Wang, Energy Environ. Sci., 2018, 11, 893–903 RSC.
- R. Liu, S. M. Mahurin, C. Li, R. R. Unocic, J. C. Idrobo, H. Gao, S. J. Pennycook and S. Dai, Angew. Chem., Int. Ed., 2011, 50, 6799–6802 CrossRef CAS PubMed.
- H. R. S. Lima, E. Airton de Oliveira Farias, P. R. S. Teixeira, C. Eiras and L. C. C. Nunes, J. Solid State Electrochem., 2019, 23, 3153–3164 CrossRef CAS.
- A. A. Ensafi, F. Rezaloo and B. Rezaei, J. Taiwan Inst. Chem. Eng., 2017, 78, 45–50 CrossRef CAS.
- E. M. Materon, A. Wong, O. Fatibello-Filho and R. C. Faria, J. Electroanal. Chem., 2018, 827, 64–72 CrossRef CAS.
- E. Asadian, S. Shahrokhian, A. Iraji Zad and F. Ghorbani-Bidkorbeh, Sens. Actuators, B, 2017, 239, 617–627 CrossRef CAS.
- J. G. Manjunatha, J. Electrochem. Sci. Eng., 2017, 7, 39–49 CrossRef CAS.
Footnote |
† These authors contributed equally to this work. |
|
This journal is © The Royal Society of Chemistry 2021 |