DOI:
10.1039/D0TC04449K
(Paper)
J. Mater. Chem. C, 2020,
8, 17176-17184
Highly efficient and thermally stable luminescence of Ca3Gd2Si6O18:Ce3+,Tb3+ phosphors based on efficient energy transfer†
Received
17th September 2020
, Accepted 26th October 2020
First published on 27th October 2020
Abstract
As phosphor-converted white light emitting diodes have emerged as new-generation light sources, developing phosphors with both high quantum efficiency (QE) and thermal stability is urgently required. One of the effective strategies is exploiting the nonradiative energy transfer to simultaneously improve the absorption of the activator and tune the emission of the sensitizer. In this work, we report a series of color-tunable phosphors by constructing an energy transfer system by co-doping Ce3+ and Tb3+ into Ca3Gd2Si6O18 (CGSO). We show that CGSO:Ce3+,Tb3+ not only exhibits color-tunable emission from violet (395 nm) to green (543 nm), but also possesses high internal QE (90.1%). Furthermore, we demonstrate that thermal stability can be significantly improved via efficient energy transfer from Ce3+ to Tb3+, which is mainly attributed to the fast energy transfer within the nearest Ce3+–Tb3+ pairs. Our results indicate that energy transfer from Ce3+ to Tb3+ provides a promising way to develop new green phosphors with high QE and superior thermal stability.
1. Introduction
Solid-state lighting (SSL) has been developed rapidly because of the excellent features such as long service time, energy saving and eco-friendliness.1–8 The phosphor converted white light emitting diode (w-LED), fabricated by combining LED chips with phosphors that can be efficiently excited by the chip light source, is the most widely used SSL to generate white light. Therefore, the luminescence properties of the phosphors play an important role in optimizing the final performance of w-LED devices. However, developing phosphors with high quantum efficiency (QE), excellent thermal stability and suitable emission is still challenging.9–12
Trivalent lanthanide ions (Ln3+) have been utilized in discovering luminescent materials because of their intra-configurational 4f → 4f transitions, which give them pure emission colors. Unfortunately, the 4f → 4f parity-forbidden transitions also endow them with quite weak absorption of the excitation light, leading to a relatively low emission efficiency.13–15 An alternative way of solving this problem is exploiting energy transfer (ET) from sensitizers with a large absorption cross section to Ln3+ by enhancing the absorptivity of excitation light in the UV or near ultraviolet (n-UV) range. Among Ln3+ ions, Tb3+ has been used as a green emission activator due to the 5D4 → 7F5 transition in some host materials for applications in lasers and lightings.16–18 However, the application of Tb3+ is limited by not only the narrow absorption lines caused by the forbidden 4f → 4f transitions but also the broad 4f8–4f75d1 parity-allowed excitation band located in the deep UV region (<300 nm).19–21 Another ion Ce3+ has strong 4f–5d emission bands that can not only match well with the absorption lines of Ln3+ but also the transition metal ions such as Mn2+ and Cr3+, and thus highly efficient ET from Ce3+ to acceptors can be realized.9,22–27 Since Ce3+ has similar ionic radii as well as the same valence as Tb3+, it can act as an effective sensitizer for Tb3+ to obtain a high QE (>80%) in some materials, such as in Ca2TbHf2Al3O12:Ce3+,9 La8Ba2(Si4P2O22N2)O2:Ce3+,Tb3+,21 and Y5Si3O12N:Ce3+,Tb3+.28
Although the reported phosphors possess high efficiency, most of them suffer from poor thermal stability (see Table S1 for a detailed comparison in the ESI†). Since the heat generated during the operation results in an unavoidable emission loss, it will limit their practical applications.29,30 In the Ce3+–Tb3+ system, most of the Ce3+ ions show limited thermal stability because the 4f–5d transitions of Ce3+ are sensitive to the crystal environment, which make the emissions of Ce3+ strongly depend on the structural properties of the host crystals. Considering that the excitation energy of Tb3+ is obtained by the ET from Ce3+, it will show a similar or even worse thermal stability than that of Ce3+ in some hosts (see Table S1 in the ESI†). In contrast, as reported by our group,26,27 the high thermal stability can be obtained when the ET rate from Ce3+ to Tb3+ is large enough to compete with the thermal quenching rate of Ce3+ 5d emission, at which the excitation energy of the Ce3+ 5d level will be captured by the thermally stable Tb3+ 5D4 level before thermal quenching happens. Therefore, the realization of efficient ET from Ce3+ to Tb3+ in proper hosts is of importance in developing phosphors with highly efficient luminescence and superior thermal stability.
In this paper, a series of novel phosphors, Ce3+ singly doped and Ce3+, Tb3+ co-doped Ca3Gd2Si6O18 (CGSO) were prepared via the traditional high-temperature solid state reaction routes. The crystal structure and optical properties of the samples were characterized by X-ray diffraction, high resolution transmission electron microscopy (HRTEM) and photoluminescence spectra. Moreover, the internal and external QE, thermal stability, ET process from Ce3+ to Tb3+ in CGSO:Ce3+,Tb3+ as well as the application performance of phosphors under 365 nm chip drive were also investigated in detail.
2. Experimental
2.1 Sample preparation
The powder samples of Ce3+ singly doped and Ce3+, Tb3+ co-doped CGSO were synthesized via the high temperature solid-state reaction.31 The stoichiometric amounts of CaCO3 (99.99%), Gd2O3 (99.99%), SiO2 (99.99%), CeO2 (99.99%), and Tb4O7 (99.99%) were used as raw materials. They were weighed and thoroughly ground in an agate mortar for 30 min. The resulting mixtures were heated at 1380 °C for 6 h under a 5% H2/95% N2 reducing atmosphere. Finally, the samples were cooled to room temperature in the furnace and ground again for the following characterization.
2.2 Characterizations
The crystal structure of samples was identified by X-ray diffraction (XRD) (Bruker D8 Focus diffractometer, in the 2θ range from 15° to 70° with Cu Kα radiation (λ = 1.54056 Å) operating at 40 kV and 30 mA). Structure refinement was carried out using the Rietveld method involving the FullProf program. HRTEM images and elemental distributions were obtained on a transmission electron microscope (JEM-2100F, JEOL) at an operating voltage of 200 kV. The emission and excitation spectra at room temperature and 77 K were recorded using the HITACHI F-7000 spectrometer. Fluorescence microscopy photoluminescence images (BX53M Instruments; OLYMPUS, Japan) of the powder particles were recorded in a dark-field mode using a 350 nm UV lamp excitation. The internal and external QE (IQE and EQE) were also measured using a spectrophotometer (QE-2100, Otsuka Photal Electronics). The IQE and EQE values were calculated by the quantum yield measurement software. The IQE defined as the ratio of the number of photons emitted (Iem) to the number of photons absorbed (Iabs) is expressed as | 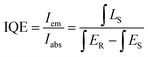 | (1) |
where LS is the emission spectrum of the sample, ES and ER are the spectra of excitation light with and without the sample in the integrating sphere. Iabs is calculated by the following equation: | 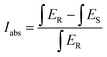 | (2) |
The EQE defined as the ratio of the number of photons emitted (Iem) to the number of photons excited (Iex) is expressed as
| 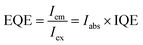 | (3) |
The fluorescence decays of Ce3+ were measured by an FLS920 fluorimeter (Edinburgh Instruments, Livingston, UK) using a hydrogen flash lamp (nF900; Edinburgh Instruments). The temperature-dependent photoluminescence spectra were also recorded on an F-7000 spectrometer with an external heater. A process controller (OMEGA CN76000) equipped with a thermocouple was used to measure the temperature and control the heating rate.
The commercial blue phosphor BAM:Eu2+, the as-synthesized CGSO:4%Ce3+,50%Tb3+, commercial red phosphor K2SiF6:Mn4+ and a UV-LED chip (365 nm) were used to fabricate a w-LED. The electroluminescence spectra of the fabricated w-LEDs were recorded using a HAAS 2000 photoelectric measuring system (350–1100, EVERFINE, China). The LED was operated at a bias current of 20 mA and a voltage of 3.4 V. All the measurements were conducted at room temperature unless mentioned specially.
3. Results and discussion
3.1 Structure analysis
All the synthesized samples were characterized by powder XRD to verify the purity. Fig. 1a shows the representative XRD patterns of CGSO:4%Ce3+, CGSO:4%Ce3+,10%Tb3+ as well as CGSO:4%Ce3+,50%Tb3+, and the calculated XRD pattern of CGSO from the Rietveld structural refinement is also shown for comparison. Clearly, all the obtained XRD patterns are in good agreement with that of calculated CGSO and no impurity phase is detected. The results indicate that the introduction of Ce3+ and/or Tb3+ into the CGSO host retains the phase purity and the doping ions are homogeneously doped in the host lattice at the current doping level. The diffraction peaks are slightly shifted to the high diffraction degree with the increase of Tb3+ concentration, which can be theoretically explained by the lattice shrinkage due to the substitution of smaller Tb3+ (r = 1.04 Å when CN = 8; r = 0.98 Å when CN = 7; r = 0.92 Å when CN = 6) for larger Gd3+ (r = 1.05 Å when CN = 8; r = 1.00 Å when CN = 7; r = 0.94 Å when CN = 6).32 In order to further investigate the structure of the as-synthesized samples, Rietveld structural refinement for the composition of CGSO:4%Ce3+ was performed by using the previously reported crystallographic data of Ca3Y2(Si3O9)2 as a starting model.33 The observed, calculated and the different patterns of the XRD refinement of CGSO:4%Ce3+ are shown in Fig. 1b, and the crystallographic data and refinement parameters of CGSO:4%Ce3+ are summarized in Table S2 in the ESI.† All the final weighted R factors (Rwp) are acceptable, which confirmed the phase purity of the sample. As illustrated in Fig. 1c and d, the obtained CGSO crystallizes in the monoclinic space group C2/c (15) according to the refinement results and there are three cation sites to occupy for Ca2+ and Gd3+. The first site is coordinated with eight oxygen atoms of which six O atoms are connected with two Si3O9 rings by sharing edges, and the remaining two are linked with the other two Si3O9 rings by sharing apices. The second site is coordinated with seven O atoms of which three O atoms comprise two edges of a Si3O9 ring, and the other four O atoms are connected with Si3O9 rings by sharing apices. The third site is coordinated with six O atoms from six Si3O9 rings.33,34 Considering the relatively large ionic radii of Ce3+, it has the possibility to occupy the three different sites when introduced into the CGSO crystal.
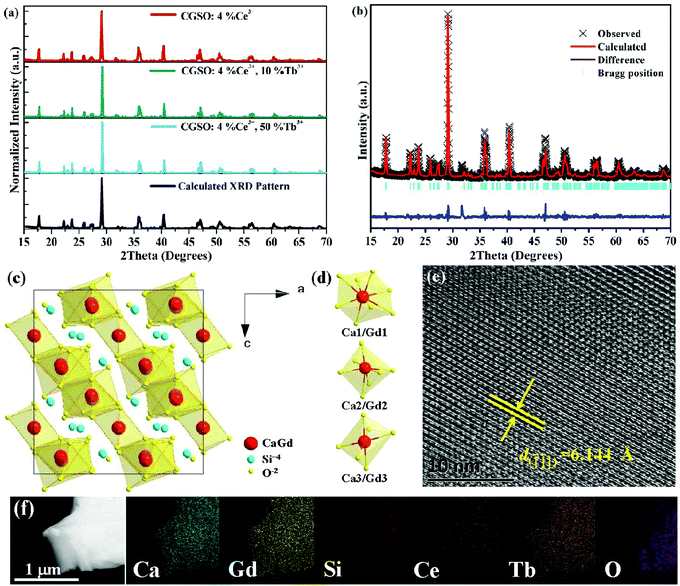 |
| Fig. 1 (a) XRD patterns of CGSO:4%Ce3+, CGSO:4%Ce3+,10%Tb3+ and CGSO:4%Ce3+,50%Tb3+; (b) Rietveld refinements of the XRD pattern of CGSO:4%Ce3+; (c) crystal structure of CGSO; (d) the coordination environment of Ca/Gd units in the lattice. (e) HRTEM images of CGSO; (f) morphology and elemental distributions of CGSO:4%Ce3+,50%Tb3+ (the scale bar is 1 μm). | |
As shown in Fig. 1e, the fine structure observation of CGSO:4%Ce3+,50%Tb3+ was performed by HRTEM to corroborate the lattice contraction of the solid solution. The clear lattice fringes in the HRTEM image indicate high crystallinity of the sample. The distance between adjacent fringes is estimated to be 6.144 Å, corresponding well with the d(![[1 with combining macron]](https://www.rsc.org/images/entities/char_0031_0304.gif)
1) spacing (6.096 Å) of the refined structure of CGSO:4%Ce3+. Fig. 1f depicts the morphology and elemental distributions of CGSO:4%Ce3+,50%Tb3+. It can be seen from the morphology that the sample displays slightly agglomerate spheres due to the high reaction temperature. The elemental distribution images give direct evidence that Ca, Gd, Si, Ce, Tb, and O homogeneously distribute in CGSO:4%Ce3+,50%Tb3+.
3.2 Photoluminescence properties
The excitation and emission spectra of CGSO:4%Ce3+ at room temperature are described in Fig. 2a. The excitation spectrum monitored at 395 nm exhibits a broad excitation band ranging from 230 to 380 nm, which is assigned to the Ce3+ parity-allowed 4f–5d transitions. Upon 325 nm excitation, the Ce3+ doped CGSO shows an intense violet emission and the corresponding emission spectrum consists of an asymmetric emission band peaking at 395 nm, originating from the lowest 5d level to the doublet ground levels (2F5/2 and 2F7/2) of the 4f configurations of Ce3+. To confirm the existence of more than one site that is occupied by Ce3+ ions, the low-temperature (77 K) emission spectrum of CGSO:0.5%Ce3+ was also measured. As shown in Fig. 2b, an additional shoulder located at 360 nm appears in the short-wavelength side of the emission spectrum. The emission spectrum can be well decomposed into four Gaussian bands on the energy scale, peaking at 355 nm (28
159 cm−1), 374 nm (26
709 cm−1), 385 nm (25
944 cm−1) and 414 nm (24
140 cm−1), respectively (see Fig. S1 for details, ESI†), which suggests that Ce3+ ions occupy at least two different sites, considering that the energy separation between the 2F7/2 and 2F5/2 ground levels of Ce3+ is about 1800 cm−1. Besides, Ce3+ highly prefers sites with large coordination number because of its relatively large ionic radius (1.01 Å), and it has been reported that the emission of six-coordinated Ce3+ in oxides is usually in the long-wavelength range (>550 nm), owing to the strong crystal field splitting.35–37 Therefore, Ce3+ only occupies eight- and seven-coordinated sites in the CGSO crystal.
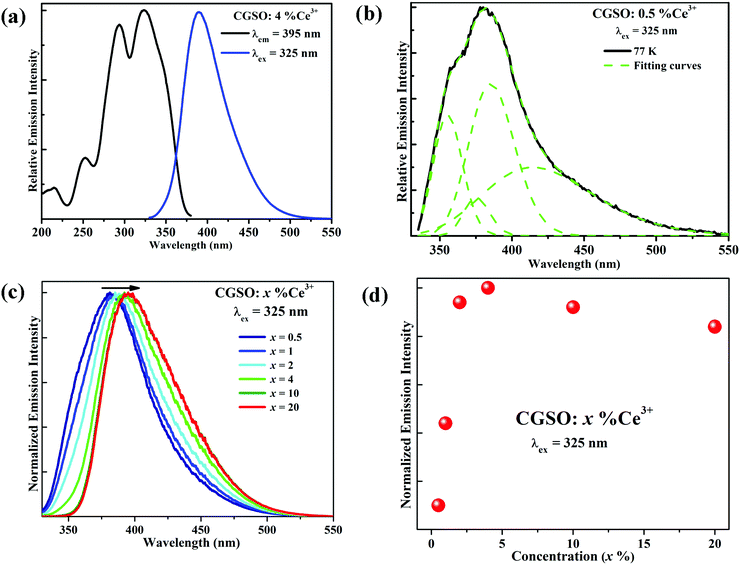 |
| Fig. 2 (a) The excitation and emission spectra of CGSO:4%Ce3+ at room temperature; (b) the emission spectrum of CGSO:0.5%Ce3+ at 77 K, the emission spectrum is fitted to display the Gaussian curves (dashed lines) characteristic of Ce3+; (c) the normalized emission spectra of sample series CGSO:x%Ce3+ (x = 0.5–20) under 325 nm excitation; (d) the emission intensities of Ce3+ as a function of Ce3+ concentration under 325 nm excitation. | |
In order to further investigate the luminescence properties, a series of Ce3+ with different doping content, CGSO:x%Ce3+ (0.5 ≤ x ≤ 20), were synthesized and the normalized emission spectra are presented in Fig. 2c. Previously, with the increase of Ce3+ concentration, the peak position of the Ce3+ emission band shifts to longer wavelength under 325 nm excitation from 381 nm to 397 nm. The above red-shift phenomenon is usually ascribed to the increase of the probability of ET from Ce3+ ions at higher 5d energy levels to those at the lower energy levels in a Ce3+ heavily doped host, leading to the energy decrease of the short-wavelength emission.38,39 Consequently, the peak positions of the emission spectra change with the doping process. The dependence of the Ce3+ emission intensity on its doping concentration is also displayed in Fig. 2d. The emission intensity of Ce3+ is found to increase gradually with the increase of Ce3+ contents, and it reaches the maximum at x = 4%, after which (x > 4%) the emission intensity decreases due to concentration quenching.40
One may notice from Fig. 2c that most of the emission of Ce3+ singly doped CGSO is shorter than 450 nm in wavelength, which limits its application in w-LEDs as a blue phosphor. However, the Ce3+ ion can be used as an effective sensitizer due to the 4f–5d transitions, transferring its energy to Tb3+ ions. As shown in Fig. 3a, for Tb3+ singly doped CGSO, when monitoring the well-known green emission at 543 nm due to the 5D4 → 7F5 characteristic transitions of Tb3+, the excitation spectrum contains not only the typical strong excitation band in the range of <300 nm that is attributed to the first spin-allowed 4f–5d transitions of Tb3+, but also some weak excitation lines in the range of 350–380 nm and at 484 nm due to the 4f–4f forbidden transition lines of Tb3+. Upon UV light (243 nm) excitation, the emission spectrum of Tb3+ is dominated by the green emission at 543 nm due to its large values of the branching ratio. A series of strong emission lines at 490, 585 and 622 nm that originate from the 5D4 → 7FJ (J = 6, 4, and 3) characteristic transitions of Tb3+ are also shown in the spectrum. Obviously, Tb3+ singly doped CGSO is useless for w-LEDs because it cannot be effectively excited by the n-UV light. However, the obvious overlap between the 4f–5d emission band of Ce3+ (350–510 nm) and the 4f–4f excitation lines of Tb3+ around 484 nm indicates the possibility of ET from Ce3+ to Tb3+, making it possible to tune the emission color from violet to green in the Ce3+ and Tb3+ co-doped CGSO. In Fig. 3a, for Ce3+ and Tb3+ co-doped CGSO, the excitation spectrum for monitoring Tb3+ emission at 543 nm shows the remarkable 5d bands of Ce3+ in the range of 300–400 nm, which is similar to that of Ce3+ emission at 395 nm. Moreover, upon excitation at 325 nm, not only the emission band of Ce3+ but also the strong emission lines peaking at 543 nm of Tb3+ appear in the emission spectrum. The above results indicate the occurrence of ET from Ce3+ to Tb3+ in Ce3+ and Tb3+ co-doped CGSO (see Fig. S2, ESI†). Therefore, Ce3+ ions can serve as a sensitizer for the Tb3+ activated phosphor to enhance the absorptivity of excitation light in the n-UV region, and then achieving an efficient green emission for w-LEDs based on n-UV LEDs.
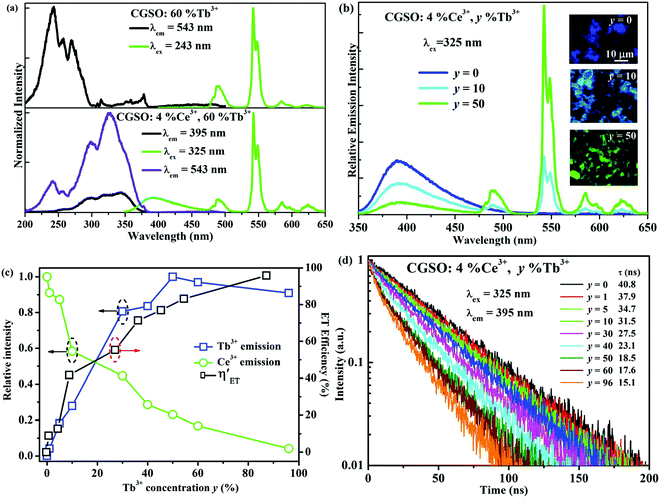 |
| Fig. 3 (a) The excitation and emission spectra of CGSO:60%Tb3+ and CGSO:4%Ce3+,60%Tb3+; (b) the emission spectra of sample series CGSO:4%Ce3+,y%Tb3+ (y = 0, 10, 50) under 325 nm excitation and the insets show the fluorescence microscopy photoluminescence images of CGSO:4%Ce3+,y%Tb3+ (y = 0, 10, 50) under 350 nm excitation; (c) the emission intensities of Ce3+ and Tb3+, the ET efficiency ηET′ as a function of Tb3+ concentration under 325 nm excitation; (d) fluorescence decay curves of Ce3+ in CGSO:4%Ce3+,y%Tb3+ (y = 0–96) phosphors (excited at 325 nm, monitored at 395 nm). | |
In order to investigate the ET process from Ce3+ to Tb3+ in the CGSO host further, the emission intensities for a fixed Ce3+ concentration at 4 mol% and various Tb3+ concentrations y% were measured under the excitation at 325 nm of Ce3+. As shown in Fig. 3b, with the increases of y from 0 to 50, the green emission intensities of Tb3+ peaking at 543 nm due to 5D4 → 7F5 transitions of Tb3+ increase accompanied by the decline of the violet emission band of Ce3+. The green emission line at 543 nm dominates the emission spectrum gradually (see Fig. 3b), resulting from the enhanced ET from Ce3+ to Tb3+ as well as the tremendous absorption coefficient of the 4f–5d allowed transition of Ce3+, which is visually reflected by the inset fluorescence microscopy photoluminescence images shown in Fig. 3b of the selected CGSO:4%Ce3+,y%Tb3+ (y = 0, 10, 50) phosphors under 350 nm excitation. As shown in Fig. 3c, the emission intensity of Tb3+ reaches a maximum value at y = 50, beyond which it starts to decrease because of the Tb3+–Tb3+ internal concentration quenching. The decay curves of Tb3+ 5D4 → 7F5 emission (543 nm) in CGSO:4%Ce3+,y%Tb3+ (y = 0, 30, 96) under 325 nm excitation were also measured and are shown in Fig. S3 (ESI†). One can find that the decay curves of Tb3+ decrease gradually with increasing Tb3+ concentration due to the energy diffusion among Tb3+ ions, which finally results in the above concentration quenching.26,41
To judge the possibility of the as-synthesized phosphors for the practical application in w-LEDs, the key parameters, IQE, Iabs and EQE of the Ce3+ singly doped and Ce3+, Tb3+ co-doped CGSO, were measured, as shown in Table 1 (the corresponding QE measurements are also shown in Fig. S4, ESI†). It is obvious that the IQE and EQE increase with the introduction of Tb3+ when compared with that of the Ce3+ singly doped sample (see Table 1), indicating the absence of QE loss during the ET process from Ce3+ to Tb3+. Additionally, the IQE of CGSO:4%Ce3+,50%Tb3+ was determined to be as high as 90.1% while its EQE was reached up to 72% under 325 nm excitation. While Some reported Ce3+/Eu2+ and Tb3+ co-doped phosphors, including their chemical composition, the effective excitation wavelength and QE are summarized in Table S1 (ESI†) for detailed comparison. One can find that the QE of CGSO:4%Ce3+,50%Tb3+ is higher than that of most reported phosphors, implying its potential application for w-LEDs as a green phosphor. It is noteworthy that the QE in Ce3+–Tb3+ is apparently higher than that in Eu2+–Tb3+ because of the existence of the unreduced Eu3+ in the as-synthesized samples.
Table 1 The IQE, Iabs and EQE of CGSO:4%Ce3+,y%Tb3+ (y = 0, 10, 30, 50)
Samples |
IQE (%) |
I
abs (%) |
EQE (%) |
CGSO:4%Ce3+ |
81.1 |
85.1 |
69.0 |
CGSO:4%Ce3+,10%Tb3+ |
95.1 |
84.6 |
80.5 |
CGSO:4%Ce3+,30%Tb3+ |
94.3 |
81.8 |
77.1 |
CGSO:4%Ce3+,50%Tb3+ |
90.1 |
80.4 |
72.4 |
In order to understand the ET mechanism in CGSO:Ce3+,Tb3+, the critical distance for the ET from Ce3+ to Tb3+, defined as the distance at which the probability of ET is equal to that of the radiative transition of Ce3+, is estimated using the following equation, as proposed by Blasse:42
| 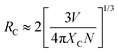 | (4) |
where
V is the volume of the unit cell,
XC is the critical concentration, and
N is the number of total sites to be occupied by the doped ions in the CGSO unit cell. For the CGSO:Ce
3+,Tb
3+ lattice,
V = 1552.05 Å
3,
N = 8, and
XC = 0.48, which is the sum of the concentration of Ce
3+ and Tb
3+. Therefore,
Rc is calculated to be 9.17 Å, which means that the average distance between Ce
3+ and its nearest Tb
3+ is larger than 5 Å, indicating that an exchange interaction may play a negligible role in this situation. In CGSO, the shortest Tb
3+–Tb
3+ distance is 3.7 Å, but a large amount of Tb
3+ (>60 mol%) is needed to completely quench the Ce
3+ emission, and thus we believe that the ET from Ce
3+ to Tb
3+ mainly takes places
via an electric dipole–quadrupole interaction,
26,27 which can also be verified by the linear fitting results according to Dexter's ET expressions of multipolar interactions and Reisfeld's approximation as shown in Fig. S5 (ESI
†). Moreover, the next-nearest neighbor Tb
3+ of Ce
3+ (6.6 Å) in CGSO is about two orders of magnitude smaller than that for the nearest neighbor Tb
3+ of Ce
3+ (3.7 Å), indicating that ET from Ce
3+ to Tb
3+ mainly occurs within the nearest Ce
3+–Tb
3+ pairs requiring high concentration of Tb
3+.
To further confirm the ET interaction between Ce3+ and Tb3+, the decay curves of Ce3+ 5d–4f emission (395 nm) on the concentration of Tb3+ in CGSO:4%Ce3+,y%Tb3+ (y = 0–96) under 325 nm excitation were obtained. One can find from Fig. 3d that the decay curve of Ce3+ deviates from the single exponential profile with increasing Tb3+ doping content, which indicates the occurrence of nonradiative ET from Ce3+ to Tb3+. The effective lifetime of Ce3+ is defined as:
|  | (5) |
where
I(
t) is the intensity at time
t. As shown in
Fig. 3d, the effective lifetime decreases monotonically with Tb
3+ concentration, strongly demonstrating the ET from Ce
3+ to Tb
3+. The ET efficiencies
ηET from Ce
3+ to Tb
3+ were also calculated based on the effective lifetime as given in Table S3 (ESI
†), from which one can find that
ηET increases with
y and reaches 63% at
y = 96, indicating an enhanced ET with the increase of Tb
3+ concentration. However, the ET efficiency from Ce
3+ to Tb
3+, labeled as
ηET′, can also be calculated based on the Tb
3+ concentration dependence of the emission intensity of Ce
3+, as listed in Table S3 (ESI
†), and the value of
ηET′ is as high as 96% for
y = 96 (see
Fig. 3c). As mentioned in our previous works,
26,27 the greater difference between the values of
ηET and
ηET′ further proves the possibility of the undetected fast decay component in the decay curve of Ce
3+. Therefore, the ET from Ce
3+ to Tb
3+ is a fast process that mainly occurs from some “quick quenching” Ce
3+ ions to their nearest neighbor Tb
3+.
3.3 Photoluminescence thermal stability
Another key characteristic with which phosphors should be judged is the luminescence thermal stability, since the operating temperature is usually high in the LED/laser diode device. Fig. 4a shows the temperature dependences of Ce3+, Tb3+ and the total emission intensities in CGSO:4%Ce3+,y%Tb3+ (y = 0, 5, 50). Under the excitation of 325 nm, the emission intensities of Ce3+ with various y values show similar thermal quenching behavior with the increasing temperature. The relative intensity drops to nearly 75% when the temperature is raised up from 30 °C to 150 °C, demonstrating a relatively poor thermal stability of Ce3+ in CGSO due to the larger thermal ionization of the excited 5d electron caused by the smaller bandgap of the CGSO host. Furthermore, Tb3+ emissions decline very slowly compared with that of Ce3+ with increasing temperature and it still maintains 92% even at 210 °C for y = 50, which indicates a superior thermal stability of Tb3+ in CGSO. Thus, the introduction of Tb3+ into Ce3+ doped CGSO considerably improves the thermal stability of the total emission intensity with increasing y. The maintained total emission intensity has been raised from 75% for y = 0 to 92% for y = 50 at 150 °C, indicating a comparable or superior thermal stability than most of the reported phosphors (Table S1, ESI†). To better understand the relationship between the total emission intensities and temperature, the activation energy ΔEa of CGSO:4%Ce3+,y%Tb3+ (y = 0, 5, 50) is given in Fig. S6 (ESI†). Obviously, ΔEa exhibits a slight increment with the increase of y, which further indicates the improved thermal stability of the total emission intensity with the introduction of Tb3+. As mentioned in our previous works,26,27 the Tb3+ concentration dependent thermal stability should be attributed to the fast ET from Ce3+ to Tb3+ within the nearest Ce3+–Tb3+ pairs as well as the energy diffusion among Tb3+ ions, which is supported by the large different decline rates between effective lifetime and emission intensity of Ce3+. Moreover, as shown in Fig. 4b–d, the locations of the emission peaks show nearly no change with increasing temperature due to the weak electron–phonon coupling between the 4f electrons and their surroundings, indicating the excellent fluorescence thermal stability of CGSO:4%Ce3+,50%Tb3+.
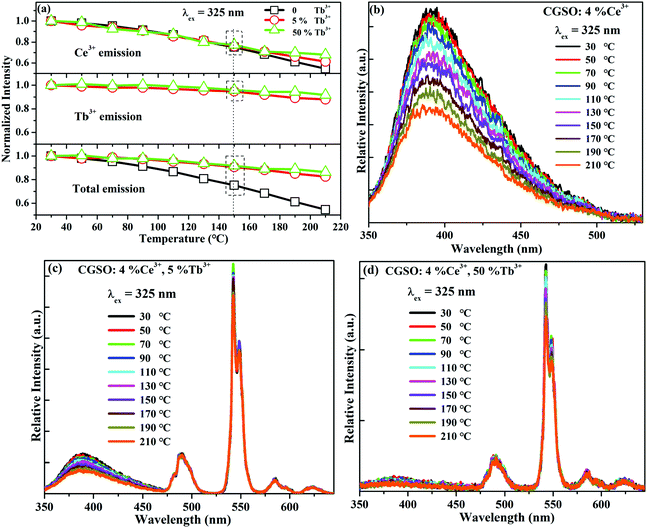 |
| Fig. 4 (a) The emission intensities of Ce3+, Tb3+ and the total emission intensities in CGSO:4%Ce3+,y%Tb3+ (y = 0, 5, 50) as a function of the temperature; temperature dependent emission spectra of CGSO:4%Ce3+ (b), CGSO:4%Ce3+,5%Tb3+ (c), and CGSO:4%Ce3+,50%Tb3+ (d) under 325 nm excitation. | |
3.4 Electroluminescence spectrum of the fabricated w-LED device
To demonstrate that CGSO:4%Ce3+,50%Tb3+ can serve as a potential green color component for w-LEDs, a prototype of w-LEDs was fabricated by combining a 365 nm UV LED chip with a mixture of commercial blue phosphor BAM:Eu2+, the as-synthesized CGSO:4%Ce3+,50%Tb3+ and commercial red phosphor KSF:Mn4+. The emission spectrum of the as-fabricated w-LEDs is shown in Fig. 5, where the weight ratio of the three phosphors is 0.7
:
50
:
6. The corresponding luminous efficiency (LE), CCT, CRI (Ra), and CIE chromaticity coordinates were determined to be 15.73 lm W−1, 5028 K, 61.4 and (0.3417, 0.3197), respectively. The w-LED packaging results indicate that the as-synthesized CGSO:4%Ce3+,50%Tb3+ is a promising candidate as a green emitting phosphor based on n-UV LEDs.
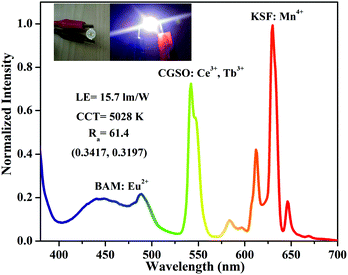 |
| Fig. 5 The emission spectrum of the fabricated w-LED based on the UV-LED chip (365 nm) and BAM:Eu2+, CGSO:4%Ce3+,50%Tb3+ and KSF:Mn4+ phosphors. | |
4. Conclusions
In summary, we obtained a high IQE (90.1%) and EQE (72.4%) of green emission in Ce3+ and Tb3+ activated CGSO. Since Tb3+ can be well sensitized by Ce3+, the Ce3+ and Tb3+ co-doped samples can be effectively excited by the n-UV excitation. The emission color of the co-doped samples can also be tuned from violet to green due to the enhanced ET from Ce3+ to Tb3+. Moreover, CGSO:4%Ce3+,50%Tb3+ shows excellent thermal stability wherein 92% of the emission intensity can be retained when the temperature is elevated to 150 °C, which is attributed to the fast ET from Ce3+ to Tb3+ as well as the energy diffusion among Tb3+ ions. Finally, the green phosphor CGSO:4%Ce3+,50%Tb3+ was employed to fabricate a w-LEDs lamp, indicating its potential to serve as a green phosphor. The results can provide a new insight in developing potential inorganic luminescent materials with high luminescence efficiency as well as good thermal stability.
Conflicts of interest
There are no conflicts to declare.
Acknowledgements
This work was partially supported by the National Natural Science Foundation of China (Grant No. 11904186, 11864028 and 51962026), the Natural Science Foundation of Inner Mongolia (Grant No. 2019BS01003), the Research Program of Science and Technology at Universities of Inner Mongolia (Grant No. NJZZ20001) and the Program of Higher-Level Talents of Inner Mongolia University (Grant No. 5185108).
References
- P. Pust, P. J. Schmidt and W. Schnick, Nat. Mater., 2015, 14, 454 CrossRef CAS.
- J. Cho, J. H. Park and J. K. Kim, Laser Photonics Rev., 2017, 11, 1600147 CrossRef.
- G. J. Hoerder, M. Seibald, D. Baumann, T. Schröder, S. Peschke, P. C. Schmid, T. Tyborski, P. Pust, I. Stoll, M. Bergler, C. Patzig, S. Reißaus, M. Krause, L. Berthold, T. Höche, D. Johrendt and H. Huppertz, Nat. Commun., 2019, 10, 1824 CrossRef.
- W. Strek, B. Cichy, L. Radosinski, P. Gluchowski, L. Marciniak, M. Lukaszewicz and D. Hreniak, Light: Sci. Appl., 2015, 4, e237 CrossRef CAS.
- Y. Wei, G. Xing, K. Liu, G. Li, P. Dang, S. Liang, M. Liu, Z. Cheng, D. Jin and J. Lin, Light: Sci. Appl., 2019, 8, 15 CrossRef.
- Q. Yao, P. Hu, P. Sun, M. Liu, R. Dong, F. Chaoke, Y. Liu, J. Jiang and H. Jiang, Adv. Mater., 2020, 32, 1907888 CrossRef CAS.
- D. Zhang, W. Xiao, C. Liu, X. Liu, J. Ren, B. Xu and J. Qiu, Nat. Commun., 2020, 11, 2805 CrossRef CAS.
- Y. Xiao, W. Xiao, L. Zhang, Z. Hao, G. H. Pan, Y. Yang, X. Zhang and J. Zhang, J. Mater. Chem. C, 2018, 6, 12159–12163 RSC.
- W. Xiao, X. Liu, J. Zhang and J. Qiu, Adv. Opt. Mater., 2019, 7, 1801677 CrossRef.
- H. B. Xu, W. D. Zhuang, R. H. Liu, Y. H. Liu, T. L. Zhou, Y. Cho, W. Gao, C. P. Yan, N. Hirosaki and R. J. Xie, RSC Adv., 2018, 8, 35271 RSC.
- W. Xiao, X. Zhang, Z. Hao, G. H. Pan, Y. Luo, L. Zhang and J. Zhang, Inorg. Chem., 2015, 54, 3189 CrossRef CAS.
- Y. Wei, Z. Gao, S. Liu, S. Chen, G. Xing, W. Wang, P. Dang, A. A. Al Kheraif, G. Li and J. Lin, Adv. Opt. Mater., 2019, 8, 1901859 CrossRef.
- D. C. Yu, R. Martin-Rodriguez, Q. Y. Zhang, A. Meijerink and F. T. Rabouw, Light: Sci. Appl., 2015, 4, e344 CrossRef CAS.
- C. Lee, H. Park, W. Kim and S. Park, Phys. Chem. Chem. Phys., 2019, 21, 24026 RSC.
- S. Lamrini, P. Koopmann, M. Schäfer, K. Scholle and P. Fuhrberg, Appl. Phys. B: Lasers Opt., 2012, 106, 315 CrossRef CAS.
- Z. Xia and R. S. Liu, J. Phys. Chem. C, 2012, 116, 15604 CrossRef CAS.
- W. Shao, C. K. Lim, Q. Li, M. T. Swihart and P. N. Prasad, Nano Lett., 2018, 18, 4892 Search PubMed.
- Z. Dou, J. Yu, Y. Cui, Y. Yang, Z. Wang, D. Yang and G. Qian, J. Am. Chem. Soc., 2014, 136, 5527 CrossRef CAS.
- M. Jiao, Q. Xu, M. Liu, C. Yang and Y. Yu, Phys. Chem. Chem. Phys., 2018, 20, 26995 RSC.
- Z. Wang, Q. Zhu, X. Wang, X. Li, X. Sun, B. N. Kim and J. G. Li, Inorg. Chem., 2018, 58, 890 CrossRef.
- L. Dong, L. Zhang, Y. Jia, B. Shao, W. Lv, S. Zhao and H. You, CrystEngComm, 2019, 21, 6226 RSC.
- P. Dorenbos, J. Lumin., 2000, 91, 155 CrossRef CAS.
- J. L. Sommerdijk and J. M. P. J. Verstegen, J. Lumin., 1974, 9, 415 CrossRef CAS.
- P. P. Dai, C. Li, X. T. Zhang, J. Xu, X. Chen, X. L. Wang, Y. Jia, X. Wang and Y. C. Liu, Light: Sci. Appl., 2016, 5, e16024 CrossRef CAS.
- Y. Xiao and Z. Hao, J. Mater. Chem. C, 2020, 8, 1151–1152 RSC.
- Y. Xiao, Z. Hao, L. Zhang, W. Xiao, D. Wu, X. Zhang, G. H. Pan, Y. Luo and J. Zhang, Inorg. Chem., 2017, 56, 4538 CrossRef.
- D. Wu, W. Xiao, L. Zhang, X. Zhang, Z. Hao, G. H. Pan, Y. Luo and J. Zhang, J. Mater. Chem. C, 2017, 5, 11910 RSC.
- S. A. Khan, N. Z. Khan, W. W. Ji, L. Ali, H. Abadikhah, L. Hao, X. Xu, S. Agathopoulos, Q. Khan and L. Zhu, Dyes Pigm., 2019, 160, 675 CrossRef CAS.
- Y. H. Kim, P. Arunkumar, B. Y. Kim, S. Unithrattil, E. Kim, S. H. Moon, J. Y. Hyun, K. H. Kim, D. Lee, J. S. Lee and W. B. Im, Nat. Mater., 2017, 16, 543 CrossRef CAS.
- L. Wang, R. J. Xie, Y. Li, X. Wang, C. G. Ma, D. Luo, T. Takeda, Y. T. Tsai, R. S. Liu and N. Hirosaki, Light: Sci. Appl., 2016, 5, e16155 CrossRef CAS.
- G. Xiang, X. Liu, W. Liu, B. Wang, Z. Liu, S. Jiang, X. Zhou, L. Li, Y. Jin and J. Zhang, J. Am. Ceram. Soc., 2020, 103, 2540 CrossRef CAS.
- R. D. Shannon, Acta Crystallogr., Sect. A: Cryst. Phys., Diffr., Theor. Gen. Crystallogr., 1976, 32, 751–767 CrossRef.
- H. Yamane, T. Nagasawa, M. Shimada and T. Endo, Acta Crystallogr., 1997, C53, 1533 CAS.
- A. P. Tyutyunnik, I. I. Leonidov, L. L. Surat, I. F. Berger and V. G. Zubkov, J. Solid State Chem., 2013, 197, 447 CrossRef CAS.
- T. Hasegawa, S. W. Kim, T. Ueda, T. Ishigaki, K. Uematsu, H. Takaba, K. Toda and M. Sato, J. Mater. Chem. C, 2017, 5, 9472–9478 RSC.
- T. Hasegawa, M. Iwaki, S. W. Kim, T. Ueda, K. Uematsu, K. Toda and M. Sato, J. Alloys Compd., 2019, 797, 1181–1189 CrossRef CAS.
- L. Feng, Z. Hao, X. Zhang, L. Zhang, G. H. Pan, Y. Luo, L. Zhang, H. Zhao and J. Zhang, Dalton Trans., 2016, 45, 1539–1545 RSC.
- W. Zhou, F. Pan, L. Zhou, D. Hou, Y. Huang, Y. Tao and H. Liang, Inorg. Chem., 2016, 55, 10415 CrossRef CAS.
- P. Dorenbos, Phys. Rev. B: Condens. Matter Mater. Phys., 2001, 64, 125117 CrossRef.
- J. M. P. J. Verstegen, J. L. Sommerdijk and J. G. Verriet, J. Lumin., 1973, 6, 425–431 CrossRef CAS.
- Y. Liu, X. Rong, M. Li, M. S. Molokeev, J. Zhao and Z. Xia, Angew. Chem., Int. Ed., 2020, 59, 11634–11640 CrossRef CAS.
- G. Blasse, Phys. Lett., 1968, 28, 444 CrossRef CAS.
Footnote |
† Electronic supplementary information (ESI) available: Fig. S1–S6, Tables S1–S3. See DOI: 10.1039/d0tc04449k |
|
This journal is © The Royal Society of Chemistry 2020 |
Click here to see how this site uses Cookies. View our privacy policy here.