White-light-emitting flexible display devices based on double network hydrogels crosslinked by YAG:Ce phosphors†
Received
27th September 2019
, Accepted 18th November 2019
First published on 20th November 2019
Abstract
White-light-emitting materials have attracted great research interest for applications in lighting, flexible display devices and biosensors. Traditional inorganic white-light-emitting materials are hard and brittle, making them difficult to apply in the field of flexible display devices. In this study, flexible and stretchable white-light-emitting double network composite hydrogels were prepared using vinyl-modified Ce-doped yttrium aluminum garnet phosphors (YAG:Ce–VTES) as crosslinkers and photoluminescent centers. The hydrogel is composed of an interpenetrating polyacrylamide (PAAm) network crosslinked by YAG:Ce–VTES and an ion-crosslinked calcium alginate network. The alginate/PAAm double network hydrogels show good stretchability and toughness, with a fracture elongation up to 600% and a compression strength up to 3.6 MPa. Moreover, white-light-emitting flexible display devices have been illuminated using a blue backlight with high luminous efficiency, optical stability and low cost. This strategy provides white-light-emitting hydrogels with good mechanical properties and lays a foundation for applications of flexible lighting and display devices.
Introduction
In recent years, white-light-emitting materials1,2 have attracted burgeoning interest in academia and industry due to their widespread applications in light emitting diodes,3–5 lighting6 and communication devices,7 especially in flexible display devices.8,9 The current strategy10 to prepare white-light-emitting materials is coating nano- or micro-scaled yellow phosphors (e.g., yttrium aluminum garnet doped Ce, denoted as YAG:Ce)11,12 on a blue backlight substrate. YAG:Ce has been known as a cost-efficient yellow phosphor for three decades. Its yellow luminescence is excited by blue light. White-light-emission is thus obtained by the combination of non-absorbed blue emission from blue light and broad yellow emission from YAG:Ce phosphors.13 However, such inorganic white-light-emitting phosphors are usually brittle14 and hard.15 The bonding strength between the phosphor powders and the substrate is insufficient, frequently leading to limited extensibility of the substrate for flexible display devices.16 Therefore, it is highly required to develop a stretchable and tough white-light-emitting material for flexible display devices.
Plenty of efforts have been made to combine light-emitting components and soft materials for fabricating white-light-emitting display devices with easy processing,8,17 good substrate adhesion18 and stable optical properties.19–21 Thiel et al.22 proposed a one-pot approach to prepare white-light-emitting gels made up of fluorescent silica gels and inorganic materials containing covalently bound chromophores with color tunability. Maji et al.23 prepared a white-light-emitting gel incorporated with lanthanide ions that were rotated on various substrates to obtain bright white light display devices. George et al.24 constructed a kind of clay-chromophore hybrid with solution processability that can be easily painted or written on glass and plastic surfaces. With the help of a commercial UV-lamp, the hybrid coated lamp glowed with a bright white color. However, most research on the preparation of white-light-emitting materials was based on the ingenious design of organic chromophores through a complicated multi-step organic synthesis.18,25 Besides, such white-light-emitting materials still suffer from unsatisfactory elasticity and toughness for flexible display devices because commonly used low-molecular weight organogels are not robust and it is difficult to tune their mechanical properties. Polymer hydrogels are a family of soft materials with highly controllable mechanical properties,26–28 and they are promising for the manufacture of flexible devices.29 They provide great opportunities to develop stretchable and tough materials for flexible display devices.
Herein, we propose a facile strategy to construct a white-light-emitting flexible display device based on a tough double network hydrogel with modified YAG:Ce phosphors as a crosslinking and reinforcing agent (Fig. 1). The first alginate network was crosslinked by using controllably released Ca2+ ions, which was subsequently used to host the free radical copolymerization of acrylamide (AAm) monomers with commercially available vinyl-modified YAG:Ce phosphors. The modified phosphors serve not only as macro-crosslinkers, but also as white-light emitting centers. Thus, the resulting double network hydrogels show excellent optical properties and high stretchability and good mechanical properties. The hydrogels are also integrated with a blue lamp to fabricate a flexible white-light-emitting display device illuminated by blue light. This work opens up a new avenue for the construction of white-light-emitting hydrogels, which is promising in the large-scale manufacture of flexible lighting and display devices.
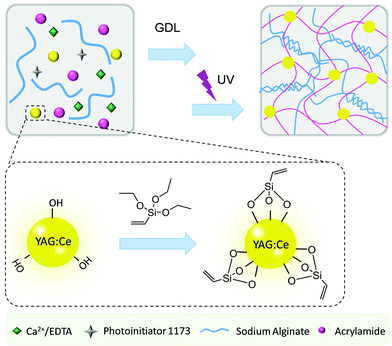 |
| Fig. 1 Schematic illustration of the preparation of alginate/PAAm double network composite hydrogels consisting of a Ca2+-crosslinked alginate network and a PAAm network crosslinked by vinyl-decorated YAG:Ce phosphors. | |
Results and discussion
Functionalization of YAG:Ce phosphors
YAG:Ce particles can be covalently modified with double bonds via the hydrolysis and condensation of VTES with the hydroxyl groups on the particle surface.30 The generated vinyl-decorated phosphor, denoted as YAG:Ce–VTES, could act as a multifunctional macro-crosslinker for the construction of a hydrogel network. The modified particles show characteristic vibration bands of C
C stretching (1600 cm−1), Si–O–C stretching (1153 cm−1) and CH2 wagging of Si–CH
CH2 (1040 cm−1) for YAG:Ce–VTES, indicating a successful modification of YAG:Ce phosphors with VTES (Fig. 2).
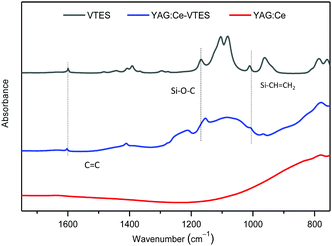 |
| Fig. 2 FTIR spectra of VTES, YAG:Ce and YAG:Ce–VTES. | |
Synthesis of alginate/PAAm double network composite hydrogels
The alginate/PAAm double network composite hydrogels were prepared by a one-pot and two-step procedure. First, the alginate network crosslinked by Ca2+ is synthesized in a controlled manner. Usually, chelation of Ca2+ ions with alginate chains is very rapid, which leads to inhomogeneous crosslinking. To tackle this problem, Ca2+ ions were chelated and protected by ethylenediaminetetraacetic acid (EDTA) to achieve a homogeneous distribution. Then, the Ca2+ ions were slowly released from EDTA cages, as triggered by H+ generated through the GDL hydrolysis, to chelate with sodium alginate, leading to the formation of an alginate network.31 Subsequently, the YAG:Ce–VTES particles and monomers were initiated by UV light to form a second network through free radical polymerization of AAm. As the monomers and the YAG:Ce–VTES particles are homogeneously dissolved and dispersed in the first alginate network, it is reasonable to assume the formation of a novel composite double network hydrogel. The obtained hydrogel is stretchable and flexible, and it can perfectly combine the fluorescent properties and mechanical properties.32 In order to investigate the effects of concentrations of AAm, alginate, and YAG:Ce–VTES on the properties of the hydrogels, a series of formulations was used for the synthesis of double network composite hydrogels (Table 1).
Table 1 The formulations for the syntheses of hydrogels with different AAm, sodium alginate and YAG:Ce–VTES concentrations
|
AAm (mol L−1) |
Alginate (wt/vol%) |
YAG:Ce–VTES (mg mL−1) |
H2O (mL) |
Photoinitiator 1173 (μL) |
A2S2Y2 |
2 |
2 |
2 |
5 |
5 |
A3S2Y2 |
3 |
2 |
2 |
5 |
5 |
A4S2Y2 |
4 |
2 |
2 |
5 |
5 |
A5S2Y2 |
5 |
2 |
2 |
5 |
5 |
A2S0.5Y2 |
2 |
0.5 |
2 |
5 |
5 |
A2S1Y2 |
2 |
1 |
2 |
5 |
5 |
A2S3Y2 |
2 |
3 |
2 |
5 |
5 |
A2S4Y2 |
2 |
4 |
2 |
5 |
5 |
A2S2Y0 |
2 |
2 |
0 |
5 |
5 |
A2S2Y4 |
2 |
2 |
4 |
5 |
5 |
A2S2Y6 |
2 |
2 |
6 |
5 |
5 |
A2S2Y8 |
2 |
2 |
8 |
5 |
5 |
A2S2Y10 |
2 |
2 |
10 |
5 |
5 |
The obtained hydrogels are yellow and opaque in visible light (Fig. 3a). The spherical YAG:Ce phosphor particles with a uniform diameter of 1–2 μm are nicely embedded within the hydrogel (Fig. 3b–d). The YAG:Ce–VTES phosphors as macro-crosslinkers are well distributed in the hydrogel network. No agglomeration takes place in the hydrogel matrix.
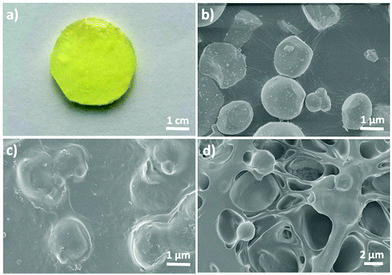 |
| Fig. 3 (a) Digital photograph and SEM images of the (c) surface and (d) cross-section of the hydrogel A2S2Y10. (b) SEM images of YAG:Ce phosphors used in this work. | |
Mechanical properties of alginate/PAAm double network hydrogels
The influences of AAm, alginate and YAG:Ce–VTES concentrations on the tensile and compression properties of alginate/PAAm double network composite hydrogels were systematically investigated. First, the concentrations of YAG:Ce–VTES (2 mg mL−1) and sodium alginate (2 wt/vol%) were fixed to study the effect of AAm concentration on the mechanical properties of the double network composite hydrogels. When the AAm concentration increased from 2 to 5 M, the tensile strength was monotonically increased from 50 to 180 kPa, and the corresponding tensile fracture strain was enhanced from 201% to 645% (Fig. 4a and b). The compression fracture strength and strain monotonically increased from 0.7 to 3.6 MPa with increasing AAm concentration from 2 to 5 M (Fig. 4c). These results indicate that increasing AAm concentration is beneficial to improving the mechanical properties of double network composite hydrogels.
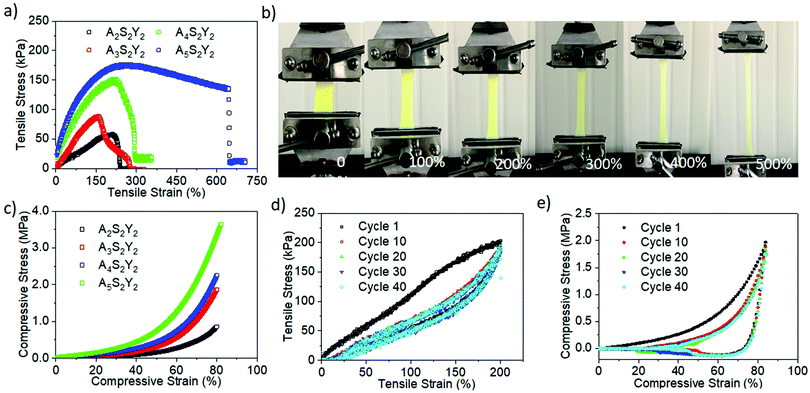 |
| Fig. 4 Representative (a) tensile and (c) compression stress–strain curves of double network composite hydrogels with different contents of AAm. (b) Snapshots of the A5S2Y2 hydrogel under uniaxial stretching at different strains. (d) Cyclic tensile and (e) compression loading–uploading stress–strain curves of the A5S2Y2 hydrogel. | |
Moreover, cyclic tensile loading–unloading tests were performed to evaluate the reversibility of the hydrogels (Fig. 4d). An obvious hysteresis was observed at the first cycle, indicating the destruction of the calcium alginate network. The loop area decreases for subsequent cycles. The loop areas, or dissipated energy, almost remain constant for the second and the following cycles. For the given tensile strains, although the first network is partly damaged, the residual first network and the flexible PAAm second network dissipate energy for cyclic loadings. On the other hand, cyclic compression loading–unloading tests produced overlapping hysteresis loops for the second and subsequent cycles (Fig. 4e). No significant loop area decay takes place for more than 40 cycles. These results suggest an excellent reversibility of the double network hydrogels.
To further investigate the influence of alginate on the properties of alginate/PAAm double network hydrogels, tensile and compression tests were also conducted on the gels with 2 mg mL−1 YAG:Ce–VTES and 2 M AAm. As the alginate concentration was increased from 0.5 to 4 wt/vol%, the tensile strength was monotonically increased from 15 to 112 kPa, and the corresponding tensile fracture strain was enhanced from 81% to 135% (Fig. 5a). On the other hand, the compression fracture strength was monotonically increased from 0.7 to 2.4 MPa with increasing the sodium alginate content from 0.5 to 4 wt/vol% (Fig. 5b). These results suggest that the increase of sodium alginate content tends to cause a rigid network.
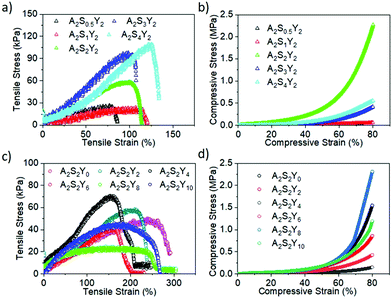 |
| Fig. 5 Representative tensile and compression stress–strain curves of double network composite hydrogels with different contents of alginate (a and b) and YAG:Ce–VTES (c and d). | |
The content of YAG:Ce–VTES as a crosslinker for the PAAm network plays an important role in the mechanical properties of the double network composite hydrogels. As the YAG:Ce–VTES concentration was increased from 0 to 4 mg mL−1, the tensile fracture strain was decreased from 280% to 180% while the corresponding tensile strength was increased from 40 to 65 kPa (Fig. 5c). Furthermore, the tensile fracture strain was increased from 180% to 250% and the corresponding tensile strength was decreased from 40 to 20 kPa when YAG:Ce–VTES concentration was increased from 6 to 10 mg mL−1. Interestingly, the compression fracture strength was improved from 0.1 to 2.4 MPa with increasing YAG:Ce–VTES particle content (Fig. 5d). Although the tensile strength is not significantly relevant, the compression fracture strength is improved with increasing the content of YAG:Ce–VTES particles due to the enhanced crosslink density of network. The mechanical properties of the obtained hydrogels can be regulated by changing the concentration of YAG:Ce, or the content of sodium alginate or AAm.
Fluorescence emission property and flexible display device
The quantum efficiency (QE) of the composite hydrogel (A2S2Y10) reached up to 65.4% obtained by an absolute method employing an integrating sphere. The fluorescence properties of the double network hydrogels were investigated by varying the content of YAG:Ce–VTES in the hydrogels (Fig. 6a). The fluorescence intensity monotonically increased with YAG:Ce–VTES concentration, indicating that it is possible to control the luminous intensity of the hydrogels. The influence of stretching on the yellow photoluminescence properties of the hydrogels has also been explored (Fig. 6b). Taking the A2S2Y10 hydrogel as an example, the fluorescence intensity decreases with increasing strain from 0% to 400%. When the tensile strain is 400%, the fluorescence intensity is reduced by 35.4%. This can be explained by the attenuated thickness of the hydrogel sample upon stretching. Note that the fluorescence emission wavelength is unchanged, regardless of the stretching degree. It is reasonable to predict that the white-light-emitting property of flexible display devices will be stable through a combination of yellow light and blue light, which is beneficial to the practical applications upon external strains.
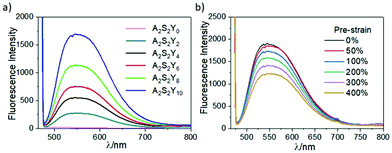 |
| Fig. 6 Fluorescence spectra of double network hydrogels with different YAG:Ce–VTES contents (a) and pre-strains (b). | |
Commonly used white-light-emitting display devices are based on the yellow–blue complementary color principle. Yellow phosphors are able to emit white light combined with a blue light irradiation. Therefore, it is expected that the prepared alginate/PAAm hydrogels containing YAG:Ce phosphors would emit white light under blue light. This is demonstrated by fabricating a flexible white-light emitting display device from the double network composite hydrogels. The viscous hydrogel precursor solution was first deposited on the surface of a commercial blue LED to form a homogeneous coating. The alginate/PAAm hydrogels (A2S2Y10) were in situ formed on the surface under UV irradiation. Thus, an easy-to-prepare white-light-emitting-diode (WLED) was fabricated. Once turned on, the LED displays a stable and bright highly pure white light (Fig. 7a). Significantly, the CIE (Commission International de L’Eclairage) coordinates of this white light are calculated to be (0.33, 0.32), which are very close to the standard ones (0.33, 0.33) (Fig. 7b). This performance is beneficial to constructing a white-light-emitting flexible display device with easy-processing and high color purity.
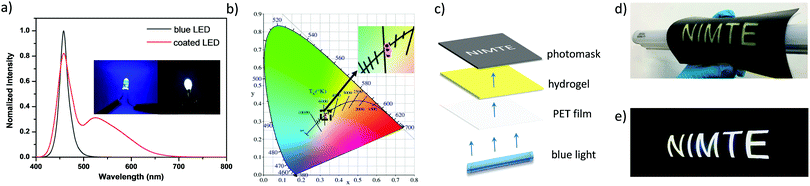 |
| Fig. 7 (a) Emission spectra of an illuminated ultraviolet LED and the same LED coated with the double network hydrogel (A2S2Y10). The CIE chromatography of the coated LED with varying current is shown in (b). (c) Schematic of the prototype for the white-light-emitting flexible display device under natural light (d) and under blue backlight (e). | |
We further regulated the current of the LED (20, 18, 16, 14, 12, 10, 8, 6, 4, 2 mA) to investigate the relationship between the white-light-emission and the intensity of blue light. Although the CIE coordinates slightly shift with varying intensity of the blue light (Fig. 7b and Table S1, ESI†), they all exhibit a stable white-light-emission property with an ideal white light CIE coordinate, regardless of the intensity of the blue light. Fig. 7c illustrates the prototype of a patterned white-light-emitting flexible display device. First, a PET substrate was coated with the hydrogel precursor. Upon UV irradiation, the hydrogel layer can be obtained and adhered onto the surface. A photomask with hollow letters “NIMTE”, for example, was covered on the top to produce a “sandwich-like” flexible device (Fig. 7d). When illuminated by a blue lamp, the device emitted a bright white light to clearly display the letters “NIMTE” (Fig. 7e). Therefore, we are able to not only prepare a prototype white-light-emitting lighting device but also fabricate a flexible display device with the assistance of a photomask.
The obtained alginate/PAAm double network composite hydrogel exhibits great potential for flexible display devices with highly controllable mechanical and optical properties. The tunable mechanical strength relies on the contents of the monomers and crosslinker (YAG:Ce–VTES) in the hydrogel. In this work, the light-emitting hydrogels show a fracture elongation as high as 600%, which is more than twice that reported in the literature.33 At the same time, the optical properties of the hydrogels can be facilely tuned by varying the content of YAG:Ce phosphor powders within the hydrogels. Moreover, the fabrication strategy consisting of in situ coating of hydrogel precursor and subsequent UV irradiated in situ polymerization is not only very suitable for large-scale industrial manufacturing, but also ensures a robust interfacial adhesion to the substrate.34 Therefore, this work presents the promising potential of commercially available phosphor-based double network hydrogels as flexible lighting and display devices with robust and stretchable mechanical properties.
Conclusions
In summary, we have developed a facile strategy to prepare alginate/PAAm double network composite hydrogels consisting of an ion-crosslinked alginate network and a PAAm network crosslinked by YAG:Ce–VTES phosphor particles. Owing to the synergistic effect of the double networks, the obtained hydrogels have superior mechanical properties, which can be regulated via changing the concentration of YAG:Ce, or the content of alginate or AAm. Combined with the yellow light emission property of YAG:Ce phosphors, the obtained composite hydrogels demonstrated a remarkable white-light-emission property mixed with the blue light irradiation. Thanks to the unique white-light-emission of the hydrogels, both lighting devices and flexible display devices can be facilely manufactured. The present strategy provides a facile way to fabricate white-light-emission materials, which would find great potential applications in flexible lighting and display devices.
Experimental
Materials
YAG:Ce phosphor (emission wavelength = 552 nm, diameter = 1–2 μm) was purchased from Jiujiang Zhihui Technology New Materials Co. Ltd (China). Vinyltriethoxysilane (VTES), sodium alginate, acrylamide (AAm), glucono delta-lactone (GDL), calcium disodium edetate (EDTA-CaNa), persulfate (KPS) and 2-hydroxy-2-methyl-phenyl-propane-1-one (Irgacure-1173, photo-initiator) were purchased from Aldrich (USA). All reagents were used as received without further purification.
Synthesis of YAG:Ce–VTES
YAG:Ce (20 mg) was suspended in water (10 mL) by sonication for 10 min. VTES (5 mL) was then added into the mixture and maintained at 75 °C for 2 h. Absolute ethanol (5 mL) was subsequently added to the suspension with sonication for 10 min. YAG:Ce–VTES was separated by centrifugation at 8000 rpm for 3 min and washed using ethanol three times. YAG:Ce–VTES was finally obtained by drying at 40 °C for 1 h.
Synthesis of alginate/PAAm double network composite hydrogels
A Ca2+/EDTA solution (C = 50 mM) was prepared by dissolving EDTA–CaNa in water. To synthesize the alginate/PAAm double network hydrogels, sodium alginate and AAm monomer were added into the Ca2+/EDTA buffer solution with mild stirring. Meanwhile, certain amounts of Irgacure-1173, YAG:Ce–VTES and GDL were added into another Ca2+/EDTA buffer solution. Subsequently, the two solutions were mixed for 30 min to form hydrogel precursors, which were carefully poured into a glass plate mold sandwiching a silicone rubber spacer (thickness = 1.5 mm). The precursor solutions were then exposed to UV (6 W, 360 nm) irradiation for 2 h. Finally, the hydrogels were obtained after leaving for another 24 h. The formulations for the preparation of hydrogels are shown in Table 1.
Characterization
Fluorescence emission spectra were recorded using a HITACHI FL-4500 (Japan) fluorescence spectrophotometer. The mechanical performance of the hydrogels was investigated using a universal testing machine (CMT 5105, China) on dumbbell specimens (12 mm × 1.2 mm × 1.5 mm) at 50 mm min−1 for uniaxial tensile tests and on cylindrical specimens (1.5 mm in height and 9 mm in diameter) for compression tests. Cyclic tensile loading–unloading tests were performed at a fixed strain of 100%, while cyclic compression loading–unloading tests were performed at a fixed strain of 80%. The functional groups of YAG:Ce particles, VTES and YAG:Ce–VTES after lyophilization were investigated by Fourier transform infrared spectroscopy (FTIR, NICOLET 6700, America). The spectra were recorded from 1750 to 750 cm−1 for samples in KBr pellets for 64 scans at 4 cm−1 resolution. The QE of the lyophilized alginate/PAAm (A2S2Y10) was directly measured by an integrating sphere (Shenzhen Candle Lighting Technology Co., Ltd) using the single photon counting mode, which was excited at the maximum absorption wavelength using a 150 W Xenon lamp.
Conflicts of interest
There are no conflicts to declare.
Acknowledgements
This study was supported by the National Natural Science Foundation of China (21574145, 51803227, 51873224), the Zhejiang Provincial Natural Science Foundation of China (LY17E030011, LQ19E030010), MOE Key Laboratory of Macromolecular Synthesis and Functionalization, Zhejiang University (2018MSF04), and CAS President's International Fellowship for Visiting Scientists (2019VBA0016).
Notes and references
- E. Schubert and J. Kim, Science, 2005, 308, 1274–1278 CrossRef CAS.
- C. Kang, J. Lee, D. Kong, J. Shim, S. Kim, S. Mun, S. Choi, M. Park, J. Kim and D. Lee, ACS Photonics, 2018, 5, 4413–4422 CrossRef CAS.
- S. Huo, P. Duan, T. Jiao, Q. Peng and M. Liu, Angew. Chem., Int. Ed., 2017, 56, 12174–12178 CrossRef CAS.
- D. K. Maiti, S. Roy, A. Baral and A. Banerjee, J. Mater. Chem. C, 2014, 2, 6574–6581 RSC.
- S. Lee, Y. K. Kim and J. Jang, Nanoscale, 2016, 8, 17551–17559 RSC.
- P. Pust, P. J. Schmidt and W. Schnick, Nat. Mater., 2015, 14, 454–458 CrossRef CAS PubMed.
- K. T. Kamtekar, A. P. Monkman and M. R. Bryce, Adv. Mater., 2010, 22, 572–582 CrossRef CAS PubMed.
- H. Wu, G. Zhou, J. Zou, C. L. Ho, W. Y. Wong, W. Yang, J. Peng and Y. Cao, Adv. Mater., 2009, 21, 4181–4184 CrossRef CAS.
- K. Benson, A. Ghimire, A. Pattammattel and C. V. Kumar, Adv. Funct. Mater., 2017, 27, 1702955 CrossRef.
- J. Liu, W. Li and K. Sun, Mater. Rev., 2007, 8, 116–120 Search PubMed.
- B. Wang, J. Ling, Y. Zhou, W. Xu, H. Lin, S. Lu, Z. Qin and M. Hong, J. Lumin., 2019, 213, 421–426 CrossRef CAS.
- S. Das, N. Okamura, S. Yagi and A. Ajayaghosh, J. Am. Chem. Soc., 2019, 141, 5635–5639 CrossRef CAS PubMed.
- M. Melucci, M. Zambianchi, G. Barbarella, I. Manet, M. Montalti, S. Bonacchi, E. Rampazzo, D. C. Rambaldi, A. Zattoni and P. Reschiglian, J. Mater. Chem., 2010, 20, 9903–9909 RSC.
- R. Liang, D. Yan, R. Tian, X. Yu, W. Shi, C. Li, M. Wei, D. G. Evans and X. Duan, Chem. Mater., 2014, 26, 2595–2600 CrossRef CAS.
- F. Zhang, Z. Shi, Z. Ma, Y. Li, S. Li, D. Wu, T. Xu, X. Li, C. Shan and G. Du, Nanoscale, 2018, 10, 20131–20139 RSC.
- A. A. Setlur, W. J. Heward, Y. Gao, A. M. Srivastava, R. G. Chandran and M. V. Shankar, Chem. Mater., 2006, 18, 3314–3322 CrossRef CAS.
- T. Wang, M. Liu, Q. Ji and Y. Wang, RSC Adv., 2015, 5, 103433–103438 RSC.
- D. Li, J. Wang and X. Ma, Adv. Opt. Mater., 2018, 6, 1800273 CrossRef.
- F. Wang, M. Kreiter, B. He, S. Pang and C. Liu, Chem. Commun., 2010, 46, 3309–3311 RSC.
- Y. C. Wu, D. Y. Wang, T. M. Chen, C. S. Lee, K. J. Chen and H. C. Kuo, ACS Appl. Mater. Interfaces, 2011, 3, 3195–3199 CrossRef CAS PubMed.
- Y. Li, P. Miao, W. Zhou, X. Gong and X. Zhao, J. Mater. Chem. A, 2017, 5, 21452–21459 RSC.
- M. Hemgesberg, S. Bay, S. Schütz, G. Dörr, S. Ernst, W. Kowalsky, T. J. J. Müller, G. Wagenblast and W. R. Thiel, Microporous Mesoporous Mater., 2013, 174, 1–9 CrossRef CAS.
- P. Sutar, V. M. Suresh and T. K. Maji, Chem. Commun., 2015, 51, 9876–9879 RSC.
- K. V. Rao, K. K. R. Datta, M. Eswaramoorthy and S. J. George, Adv. Mater., 2013, 25, 1713–1718 CrossRef CAS.
- Z. Xie, C. Chen, S. Xu, J. Li, Y. Zhang, S. Liu, J. Xu and Z. Chi, Angew. Chem., Int. Ed., 2015, 54, 7181–7184 CrossRef CAS.
- J. Gong, Y. Katsuyama, T. Kurokawa and Y. Osada, Adv. Mater., 2003, 15, 1155–1158 CrossRef CAS.
- J. Ma, J. Luo, Y. Liu, Y. Wei, T. Cai, X. Yu, H. Liu, C. Liu and J. C. Crittenden, J. Mater. Chem. A, 2018, 6, 20110–20120 RSC.
- J. Sun, X. Zhao, W. R. K. Illeperuma, O. Chaudhuri, K. H. Oh, D. J. Mooney, J. J. Vlassak and Z. Suo, Nature, 2012, 489, 133–136 CrossRef CAS.
- K. Wang, X. Zhang, C. Li, H. Zhang, X. Sun, N. Xu and Y. Ma, J. Mater. Chem. A, 2014, 2, 19726–19732 RSC.
- L. Wang, F. Zhao, X. Yang, C. Pan and H. Huang, RSC Adv., 2015, 5, 26339–26345 RSC.
- G. Du, F. Wu, Y. Cong, L. Nie, S. Liu, G. Gao and J. Fu, Chem. Commun., 2015, 51, 15534–15537 RSC.
- J. Du, S. Xu, S. Feng, L. Yu, J. Wang and Y. Liu, Soft Matter, 2016, 12, 1649–1654 RSC.
- J. Jia and H. Jia, Chin. J. Lumin., 2017, 38, 1493–1502 CrossRef.
- H. Lin, C. Sher, C. Lin, H. Tu, X. Chen, Y. Lai, C. Lin, H. Chen, P. Yu, H. Meng, G. Chi, K. Honjo, T. Chen and H. Kuo, ACS Appl. Mater. Interfaces, 2017, 9, 35279–35286 CrossRef CAS PubMed.
Footnote |
† Electronic supplementary information (ESI) available. See DOI: 10.1039/c9tc05311e |
|
This journal is © The Royal Society of Chemistry 2020 |