Effect of stress on the molecular structure and mechanical properties of supercontracted spider dragline silks†
Received
18th September 2019
, Accepted 16th November 2019
First published on 18th November 2019
Abstract
Supercontraction is one of the most interesting properties of spider dragline silks. In this study, changes in the secondary structures of the Nephila edulis spider dragline silk after it was subjected to different supercontraction processes were investigated by integrating synchrotron Fourier transform infrared (S-FTIR) microspectroscopy and mechanical characterization. The results showed that after free supercontraction, the β-sheet lost most of its orientation, while the helix and random coils were almost totally disordered. Interestingly, by conducting different types of supercontractions (i.e., stretching of the free supercontracted spider dragline silk to its original length or performing constrained supercontraction), it was found that although the molecular structures all changed after supercontraction, the mechanical properties almost remained unchanged when the length of the spider dragline silk did not change significantly. The other interesting conclusion obtained is that the manual stretching of a poorly oriented spider dragline silk cannot selectively improve the orientation degree of the β-sheet in the spider silk, but increase the orientation degree of all conformations (β-sheet, helix, and random). These experimental findings not only help to unveil the structure–property–function relationship of natural spider silks, but also provide a useful guideline for the design of biomimetic spider fiber materials.
Introduction
Nature has taught us numerous lessons about environmentally friendly technologies and futuristic materials with outstanding properties.1–3 Spider silk, which is considered to be the toughest natural material, possesses a unique combination of strength and extensibility. It has a wide range of potential applications including serving as a prototype model for the design of future materials.4–8 Spiders usually produce several kinds of spider silks for a variety of purposes ranging from building a web to swathing prey.9 For instance, the orb-web spiders have up to seven sets of silk-producing glands, each can synthesize a different type of spider silk with different and unique mechanical properties.9 Spider dragline silk, secreted from major ampullate, is one of the most fascinating types of spider silk due to its ultra-strong mechanical properties, and is used as a framework thread for the spider web and safety line.9–13 The unique physical properties of spider dragline silk can be attributed to the hierarchical structure of the silk protein in the fibrous state.12 X-ray diffraction studies have shown that spider dragline silk consists of ordered (crystalline) and amorphous (non-crystalline) structures. The ordered region arises through the stacking of pleated β-sheets that are regarded as the rigid nodes in the amorphous network linking protein chains.14–16
In addition to the extraordinary mechanical properties, spider dragline silk demonstrates an interesting phenomenon known as supercontraction, i.e., when unrestrained spider dragline silk is immersed in water, it displays a macroscopic reduction in length of about 50% and a concomitant swelling in diameter.17–19 Supercontraction refers to the interference of water molecules with the original arrangement of the molecular chains in the spider dragline silks. Previous studies on hydrated and supercontracted spider dragline silk samples reveal that water primarily affects the amorphous part of the silk.20–23 To date, a variety of experimental techniques, such as FTIR spectroscopy,20,23 Raman spectroscopy,24–27 X-ray diffraction,22,28,29 NMR spectroscopy,29–34 and birefringence,35 have been employed in the studies of spider dragline silk with supercontraction. The results from these studies suggest that supercontraction is driven by the recoverable disorientation of the molecular chains in the oriented amorphous region of the spider dragline silk.22,25,31,34–36 For example, Yang et al. studied the spider dragline silk with 13C and 2H NMR and concluded that the highly conserved YGGLGS(N)QGAGR blocks in the silk protein play a major role in the supercontraction process.30 Previous research from our laboratory and collaborators paid a lot of attention to the supercontraction behaviour of spider dragline silk.37–39 It was found that spider dragline silks spun under different conditions showed different supercontraction abilities. These silks with different supercontraction abilities exhibited different mechanical properties, which correlated well with Csh (maximum shrinkage of an unconstrained fiber under standard conditions). However, in the supercontracted state, the variation of mechanical properties with Csh declined significantly. These results suggest that the supercontracted state may be considered as an underlying reference state that is only slightly influenced by the reeling conditions or sample history.39 Also, it was found that the supercontraction behaviour of spider dragline silk was related to the proline content in the silk.38,40 Sampath et al. also suggested that the size and orientation of the crystalline component in the spider dragline silk may be correlated with the proline content.15 However, it is challenging to understand why Antheraea pernyi silkworm silk, which contains few proline residues can still contract in water,41 implying that proline may not be the only factor causing the supercontraction of animal silks.
The supercontraction apart from changing the length of spider dragline silk also reduces its stiffness by up to an order of magnitude while significantly increasing its extensibility. Such large variations in the mechanical properties can be attributed to the change in the secondary structures of the spider silk in the process of supercontraction.19,39,42–44 Ene et al. explored the effects of stretching on the molecular level of native and supercontracted spider dragline silks and found that the nanostructure of supercontracted silk was irreversibly transformed to the state similar to that of native spider silk at high stresses.23 Vehoff et al. concluded that the impact of humidity can clearly explain the significant variation of mechanical properties and stress–strain curves reported in the literature.44
After decades of study, researchers have gained considerable understanding of this supercontraction phenomenon of spider dragline silk. However, there are limited reports on either the change in the secondary structure or the reorientation of amorphous and crystalline regions in the spider dragline silk during the supercontraction process. It is vital to understand the change of microstructures in the spider dragline silk during the supercontraction process as it holds the key to understanding the nature of supercontraction and how it affects the mechanical properties.
FTIR has been a sensitive tool used in the characterization of protein secondary structures for a long time.45,46 Owing to the ultra-brightness and high spatial resolution of the synchrotron infrared beam, we have successfully used synchrotron FTIR (S-FTIR) spectroscopy previously to analyse the single silk fiber qualitatively and quantitatively. We were able to determine the content and orientation degree of different secondary structures in various natural and regenerated silk fibers.47–52 Therefore, in this study, we continue to use this technique to determine the change of secondary structure and molecular orientation in the Nephila edulis spider dragline silk during different supercontraction processes. Also, we correlated the change in these microscopic molecular structures to the macroscopic mechanical properties reflected on spider dragline silks after supercontraction in order to establish a reasonable structure–property relationship.
Experimental section
Sample preparation
Spider dragline silks from N. edulis were used as samples in this work. Spider dragline silks were forcibly reeled from the spider at 20 mm s−1 (a speed comparable to natural spinning rates25) in laboratory conditions (20–25 °C and 40–45% relative humidity). Details of the silk reeling procedure can be found in our previous work.25,39 Six samples were designed based on the different supercontraction processes that are illustrated in Scheme 1. Sample A is the original spider dragline silk with a length of L0. Sample B is the free supercontracted spider dragline silk, i.e., the spider silk was first contracted without any constraints in the deionized water (the final length was about 55% L0) for 1 h and then dried in air. Sample C is the spider dragline silk, which after free contraction in water for 1 h was quickly stretched to its original length L0, and then dried in air. Samples D to F are the supercontracted spider dragline silks in the constrained conditions. Sample D is a 100% constrained sample, in which the final length after supercontraction was the same as the original length L0 and immersed in deionized water for 1 h, and then, dried in air. Samples E and F are 90% and 70% constrained samples, which set the final lengths after supercontraction to 90% and 70% of the original length L0, respectively. The detailed sample description is provided in Table 1. To easily and precisely control the length of the spider dragline silks after different supercontraction processes, the silk samples were first stuck on two feet of compasses. Then, by adjusting the distance between the two feet, the designed length of the silk sample after the supercontraction process could be controlled.
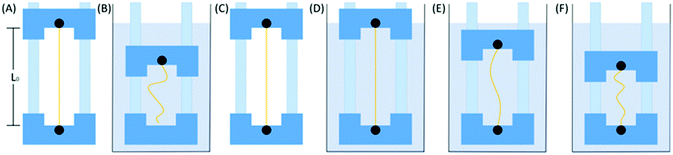 |
| Scheme 1 Description of the sample preparation method using a controlled supercontraction process. (A) Original N. edulis spider dragline silk with a length L0; (B) free supercontraction in water; (C) stretched to original length L0 after free supercontraction in water; (D) 100% constrained supercontraction in water; (E) 90% constrained supercontraction in water; and (F) 70% constrained supercontraction in water. | |
Table 1 Description of supercontracted Nephila edulis spider dragline silk samples
|
Description |
Final length |
Sample A |
Original spider dragline silk |
100% L0 |
Sample B |
After free supercontraction |
55% L0 |
Sample C |
Stretched to original length after free supercontraction |
100% L0 |
Sample D |
After 100% constrained supercontraction |
100% L0 |
Sample E |
After 90% constrained supercontraction |
90% L0 |
Sample F |
After 70% constrained supercontraction |
70% L0 |
Mechanical testing
The mechanical properties of one control and five supercontraction samples (total length of approximately 30 mm) were tested using an Instron 5565 mechanical testing machine (at 25 °C and 45% RH; gauge length: 10 mm; cross-head speed: 5 mm min−1) with a load cell of 2.5 N. At least 15 replicates from individual spider dragline silks were used for mechanical testing. The cross-section of the original spider dragline silks was observed by a Tescan 5136MM scanning electron microscopy (SEM) at 20 kV after sputtering with gold. The areas of the original spider dragline silks were calculated using software Atlas 2.9.9.9, which was provided with the SEM equipment. The diameters of the supercontraction samples were calculated from the diameter of the original spider dragline silk and the final length of the silk, under the hypothesis of constant volume.39,42,53
S-FTIR microspectroscopy
The experiments were performed using a Beamline U4 at the National Synchrotron Radiation Laboratory (NSRL), Hefei and Beamline BL01B1 at Shanghai Synchrotron Radiation Facility (SSRF), China. The description of Beamline U4 and BL01B1 can be found in our previous publications.47–49 The detailed experimental conditions can be found in the ESI.† The S-FTIR spectra obtained using an original synchrotron IR beam were defined as unpolarized spectra. In order to obtain the S-FTIR spectra with a specific polarized angle, an infrared polarizer was inserted into the optical path. The spectra obtained in these experiments were defined as polarized spectra. We used a PeakFit 4.12 to deconvolute the amide III band (1265 cm−1 for helix, 1224 cm−1 for β-sheet, and 1235 cm−1 for random coil) to calculate the content of different conformations in spider dragline silks as reported in our previous work.47,48 The method to calculate the absorbance profile and molecular order parameter (Smol) also can be found in our previous work.48 It should be noted that the spectra for the calculation of the content of secondary structures were collected under unpolarized conditions. The polarized spectra are only used for estimating the molecular order parameter Smol.
Result and discussion
Conformational changes in spider dragline silk after free supercontraction
In our previous works, a robust method was set up to semi-quantitatively determine the content of the secondary structure in single animal silks by analyzing the amide III band in S-FTIR spectra.47,48 As described before, amide III bands are chosen to perform the calculation since the amide I bands of the single spider dragline silks are significantly distorted in their baseline due to the scattering effect of the samples. The polarization of the IR light has a significant effect on the FTIR spectra of the silk fibers. Therefore, in current experiments, the spectra that were used for the calculation of the secondary structures were collected under unpolarized conditions. It should be noted that in this work, we focus on understanding the relative changes of the β-sheet contents instead of their “true” values. For the N. edulis dragline silk, the peaks at 1265, 1235, and 1224 cm−1 were assigned respectively to helix (may include α-helix and 310 helix),54 random coil, and β-sheet conformations as before.47 Fig. S1 (ESI†) is the amide III bands of the S-FTIR spectra of three different single spider dragline silk samples, i.e., the original one, the one after free supercontraction, and the one stretched to its original length after free supercontraction. It showed clearly that the shape of the amide III band varied significantly. Fig. 1 shows the deconvolution results of these amide III bands and Table 2 lists the contents of the secondary structures in different single dragline silk samples. The β-sheet content of the original spider dragline silk was about 16.1%, which is consistent with our previous report.55 After free supercontraction, the β-sheet content increased to 21.2%, while both the random coil and helix content decreased accordingly. These results suggest that during the supercontraction process, a certain amount of random coil and helix conformation was converted to β-sheet conformation. It has been reported that supercontraction can cause a permanent change in the secondary structure of spider dragline silk as compared to its dry native structure.56 An earlier work also found that by soaking spider dragline silk in water, there was an increase in the fraction of alanine residues adopting β-sheet conformations.57 Although they did not specifically refer to the concept of supercontraction, the phenomenon is similar as per their NMR results and our S-FTIR analysis in this study. According to the literature, water can disrupt the intermolecular interactions between the protein chains that results in a reduced steric hindrance. This phenomenon promotes the chain movement in the amorphous region and thus induces β-sheet formation in the animal silks.53,58–60 Therefore, during the supercontraction process, water acts as a plasticizer and significantly increases the mobility of the molecular chains in the spider dragline silk,61,62 inducing those hydrophobic poly(Ala)n segments converting from random coil and/or helix conformation to more thermodynamically favoured β-sheet structures.36 Similarly, Lefèvre et al. reported that there was a 3% increase of β-sheet in both N. clavipes (from 36% to 39%) and A. diadematus (from 27% to 30%) spider silk after supercontraction.27 In addition, we also found that the β-sheet in Antheraea pernyi silkworm silk increased 8% after supercontraction.63
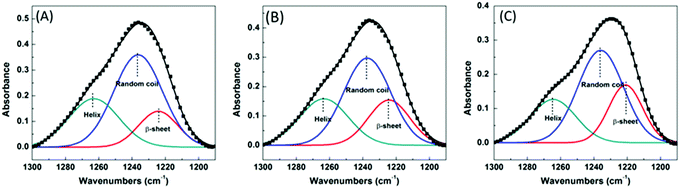 |
| Fig. 1 Deconvolution results of amide III bands in S-FTIR spectra of single spider dragline silk. (A) Original spider dragline silk; (B) after free supercontraction; and (C) stretched to original length after free supercontraction. | |
Table 2 Comparison of secondary structures of different single N. edulis spider dragline silk samples
Sample |
β-Sheet (%) |
Random coil (%) |
Helix (%) |
Original |
16.1 ± 1.1 |
54.9 ± 2.1 |
29.0 ± 1.2 |
After free supercontraction |
21.2 ± 1.3 |
50.4 ± 1.8 |
28.4 ± 1.4 |
Stretched to original length after free supercontraction |
24.1 ± 1.3 |
51.3 ± 1.4 |
24.6 ± 1.1 |
After 100% constrained supercontraction |
16.3 ± 0.7 |
64.3 ± 1.5 |
19.4 ± 2.4 |
After 90% constrained supercontraction |
17.1 ± 0.6 |
55.9 ± 0.9 |
27.1 ± 1.1 |
After 70% constrained supercontraction |
19.9 ± 1.7 |
51.9 ± 1.2 |
28.2 ± 1.2 |
When the spider dragline silk after free supercontraction was stretched to its original length, the content of conformation only varied by a little amount. There was a slight increase in the β-sheet and random coil content at the expense of the decrease in the helix content. This is because after stretching, the molecular chains in the helix region became more extended and some poly(Ala)n part converted to a more stable β-sheet structure, while others adopted the random coil structure. Although there was not much change in the contents of different conformations, it can be assumed that the orientation of the molecular chains would change by a large amount.
Supercontraction is known to cause a loss in the orientation of the molecular chains in the spider dragline silk as is evidenced by various characterization techniques.22,31–36 For instance, Fornes et al. used birefringence to determine the changes of molecular orientation in the spider silk, concluding that the molecular chains lost orientation after supercontraction.35 However, the change of birefringence can only inform about the total orientation in spider dragline silk, but cannot indicate what precisely happens to the secondary structure (β-sheet, helix, or random coil region). In our previous work, it has been proved that polarized S-FTIR microspectroscopy is a suitable tool to explore the orientation of different conformations in the single silk fiber.48 Fig. S2 (ESI†) shows a series of S-FTIR microspectra in the 1500–800 cm−1 region of a single original N. edulis spider dragline silk at different polarization angles (Ω, 0–90° in 10° steps). It is clearly shown that the absorption of the β-sheet peak at 1222 and 965 cm−1 became progressively smaller from Ω = 0° (infrared beam parallel to the fiber axis) to Ω = 90° (perpendicular to the fiber axis), indicating the dichroism along the fiber axis. In the meantime, the shape of the amide III band also demonstrated a significant dependence on Ω. As per our previous work,48 the amide III band at different Ω was deconvoluted into the β-sheet, random coil, and helix component. By plotting the relative intensity of each conformation at different polarization angles, a polar plot of β-sheet (1224 cm−1), random coil (1235 cm−1), and helix (1265 cm−1) component in amide III band of original N. edulis spider dragline silk is presented in Fig. 2A. The figure shows intuitively that all the β-sheet, helix, and random coil in the original spider dragline silk had a preference for the orientation parallel to the fiber axis. In the meantime, the values of molecular order parameter (Smol) of the β-sheet, random coil, and helix are listed in Table 3. The Smol of the β-sheet was 0.828, which indicates that the β-sheet region in the spider dragline silk was highly oriented along the fiber axis. Meanwhile, the Smol of the helix and random coil were 0.133 and 0.069, respectively, suggesting a better orientation of the helix region as compared to the random coil region; however, both were much worse than the β-sheet region. Such results on the orientation of the original spider dragline silk are consistent with our previous studies on Antheraea pernyi silkworm silk with S-FTIR48 and Samia Cynthia ricini silkworm silk in other research groups with Raman spectroscopy.64 The phenomenon of poor orientation but not the total disorder of the helix and random coil region in the original spider dragline silk was consistent with the results from the Raman study of N. edulis and N. clavipes spider dragline silk,64 and solid-state NMR study of N. edulis spider dragline silk,33 which reported a certain degree of orientation along the fiber axis for the amorphous region. However, the results from the previous ATR-IR spectroscopy study of N. clavipes spider dragline silk indicated that the amorphous phase almost had no orientation.65
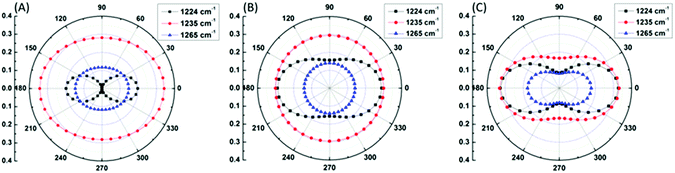 |
| Fig. 2 Polar plot of the different components of the conformations (β-sheet: 1224 cm−1, random coil: 1235 cm−1, helix: 1265 cm−1) of the single spider dragline silk. (A) Original spider dragline silk; (B) after free supercontraction; and (C) stretched to original length after free supercontraction. | |
Table 3 Molecular order parameters (Smol) of different conformations in single spider dragline silk of N. edulis
Sample |
S
mol
|
β-Sheet |
Random coil |
Helix |
Original |
0.828 |
0.069 |
0.133 |
After free supercontraction |
0.222 |
0.006 |
0.002 |
Stretched to original length after free supercontraction |
0.490 |
0.247 |
0.279 |
After 100% constrained supercontraction |
0.713 |
0.135 |
0.237 |
After 90% constrained supercontraction |
0.603 |
0.094 |
0.199 |
After 70% constrained supercontraction |
0.501 |
0.087 |
0.169 |
After free supercontraction in water, the orientation of the molecular chains in spider dragline silk decreased dramatically. As shown in Fig. 2B, the polar plot of the helix and random component was almost a circle, indicating the total loss of orientation. Meanwhile, the dichroism of the β-sheet component along the fiber axis became much worse. Semi-quantitatively, Smol of β-sheet decreased from 0.828 to 0.222 while the Smol of the helix and random coil was close to 0 (Table 3). This is strong evidence for the fact that during the supercontraction process, water acted as a plasticizer that broke the hydrogen bonds formed during the spinning process, resulting in an entropy-driven recoiling of the molecular chains.24,55 Hence, the phenomenon caused a random arrangement of the random coil and helix components in the spider dragline silk because the hydrogen bonds between the chain segments in the amorphous region (helix and random coil) were expected to be more vulnerable to water molecules.40 Although it was difficult for water to penetrate into the β-sheet nanocrystals to affect the hydrogen bonds inside, the random rearrangement of the amorphous region deviated the β-sheet nanocrystals from their original orientation; thus, decreasing the orientation level of the β-sheet component by a large amount.
Of note, the content of the secondary structures and molecular order parameters of the spider dragline silk obtained by this work are not totally consistent with the values reported in other literature (Table S1, ESI†). This difference is intrinsic due to the difference definitions of the secondary structures, especially for β-sheet or β-sheet crystals in different characterization methods. Take the XRD measurements as an example, the content of β-sheet crystals of B. mori silkworm silk and N. clavipes spider dragline silk in the literature are changed from 37–56% and 10–28%, respectively.14,15,66 This considerable disagreement can be attributed to both uncertainty inherent in the method and the differences between the specimens measured.67 However, the changing trend of the β-sheet obtained is effective, which can be used to understand the relative change in secondary structure during supercontraction.
After stretching the spider dragline silk after free supercontraction, the orientation of all components increased (Fig. 2C). However, the orientation level of the β-sheet component (Smol = 0.490) could not recover to its original level (Smol = 0.828). On the contrary, the orientation level of the helix and random coil clearly increased compared to those in the original spider dragline silk (Table 3). Such a phenomenon suggests that manual stretching aligned the whole molecular chain along the fiber axis, which is not the same as in the natural spinning process (only the β-sheet structure was highly oriented along the fiber axis). In the experimental setup, the length of the spider dragline silk remained the same; hence, the improved orientation level of the helix and random coil should sacrifice the orientation level of the β-sheet. However, notably, in the natural spinning process, animals can align the crystalline region (β-sheet) extremely well along the fiber axis, but leave the amorphous region (helix and random) relatively disordered. This may be one of the important reasons for the excellent combination of strength and extensibility in the natural animal silk, demonstrating a portfolio of outstanding mechanical properties (Fig. 3).
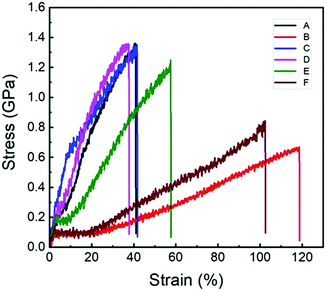 |
| Fig. 3 Representative stress–strain curves of the N. edulis spider dragline silks at different conditions. (A) Original spider dragline silk; (B) after free supercontraction; (C) stretched to original length after free supercontraction; (D) after 100% constrained supercontraction; (E) after 90% constrained supercontraction; and (F) after 70% constrained supercontraction. | |
Mechanical properties of spider dragline silk after free supercontraction
Fig. 3 shows the representative stress–strain curves of the original spider dragline silk (curve A) and the sample after free supercontraction (curve B) as well as the one stretched to its original length after free supercontraction (curve C). Their related mechanical properties are listed in Table 4. As shown, after free supercontraction, the Young's modulus and breaking stress of the spider dragline silk decreased significantly (from 10.5 GPa to 5.9 GPa and from 1.3 GPa to 0.6 GPa, respectively), but the breaking strain increased by a large amount (from 39% to 117%). When stretched to the original length after free supercontraction, the mechanical properties seemed to recover extremely well. The values of Young's modulus, breaking stress, and breaking strain were almost the same as those of the original spider dragline silk. However, a closer look into the stress–strain curves revealed that the position of the yielding point was changed. The yield stress and strain of the original spider dragline silk were approximately 0.2 GPa and 4%, but after the free supercontraction and then stretching to its original length, they increased to 0.6 Ga and 10%, respectively. This meant after such a treatment, the spider dragline silk actually became tougher, i.e., had a larger breaking energy.
Table 4 Mechanical properties of N. edulis spider dragline silks at different conditions
Sample |
Breaking stress (GPa) |
Breaking strain (%) |
Young's modulus (GPa) |
Original |
1.3 ± 0.1 |
39 ± 6 |
10.5 ± 1.2 |
After free supercontraction |
0.6 ± 0.1 |
117 ± 5 |
5.9 ± 0.8 |
Stretched to original length after free supercontraction |
1.3 ± 0.1 |
40 ± 5 |
10.4 ± 1.9 |
After 100% constrained supercontraction |
1.3 ± 0.1 |
34 ± 4 |
11.1 ± 1.1 |
After 90% constrained supercontraction |
1.2 ± 0.1 |
61 ± 6 |
9.1 ± 1.6 |
After 70% constrained supercontraction |
0.8 ± 0.1 |
100 ± 6 |
7.7 ± 1.1 |
Conformational changes in the spider dragline silk after constrained supercontraction
The spider fibers in the web are fixed in nature, implying that the supercontraction of the spider dragline silk mostly occurs under constrained conditions. To deeply analyse the effect of supercontraction on the structure of the spider dragline silk, three different constrained supercontraction samples were prepared (Table 1). By using the same deconvolution method on the amide III band (Fig. S3, ESI†), the contents of β-sheet, random coil, and helix conformation of different constrained supercontraction samples were obtained (Table 2). Compared to the original spider dragline silk, in the totally constrained silk, though there was no change in the fiber length, the composition of conformations still varied. The primary difference was in the random coil and helix contents. After 100% constrained supercontraction, the helix content decreased by about 10%, while the random coil content increased by about 10%, with β-sheet content remaining almost unchanged. With the decrease in the degree of confinement, both the β-sheet and helix content increased, while the random coil content decreased. In this study, for the spider dragline silk with the lowest degree of confinement (70% constrained supercontraction), there were approximately 3% random coils converting into β-sheets while there was almost no change for the helix as compared to the original counterpart. A substantial contraction stress can be generated in the spider silk during the constrained supercontraction process.56,68,69 For 100% constrained supercontraction, the molecular chains sustained the strongest contraction stress because the total length of the spider dragline silk was unchanged. The rearrangement of random coil was not enough to counteract the stress; therefore, a considerable proportion of helix (about 10%) had to break down into the random coil. However, when conducting 90% constrained supercontraction, there was an increased space for the rearrangement of the random coil, which only needed about 2% helix to break down to balance the contraction stress. With the decrease in the constrained degree to 70%, there was enough room for the random coil to rearrange; hence, no helix was needed for breaking down. In addition, as random coils could rearrange relatively freely in such conditions, some random coils even could assemble into β-sheet (about 3%), though it was less than that in the free supercontraction (about 5%).
In addition to variation in contents, the orientation of different conformations also changed after being subjected to constrained supercontraction. Fig. S4 (ESI†) shows the difference in the shape of polarized S-FTIR microspectra in amide III band between the original and 100% constrained spider dragline silk. The value of Smol of different conformations of single spider dragline silk after constrained supercontraction is presented in Table 3. The Smol of the random coil and helix in the spider dragline silk after 100% constrained supercontraction increased quite significantly as compared to the original ones. This phenomenon can also be attributed to the strong contraction stress generated during the 100% constrained supercontraction process. On the other hand, Smol of the β-sheet decreased because the rearrangement of the molecular chains in the random coil and helix region dragged the β-sheet structures to deviate from their original direction in order to maintain the fiber length. With the decrease in constrained degree, the molecular chains had more space to rearrange; hence, the contraction stress became increasingly smaller from 100% to 70% constrained supercontraction. Therefore, Smol of β-sheet, random coil, and helix decreased correspondingly with the decrease in the constrained degree.
Mechanical properties of spider dragline silk after constrained supercontraction
The mechanical properties of the spider dragline silk after 100% constrained supercontraction seemed not to change significantly as compared to the original spider silk. The stress–strain curve was similar (Fig. 3) and the breaking stress was almost the same (Table 4). The only difference was the increase in Young's modulus by a little amount (from 10.5 to 11.1 GPa), while the breaking strain decreased by a little amount (from 39% to 34%). The spider dragline silk seemed to have become a little bit stiffer after 100% constrained supercontraction. However, when the constrained degree was decreased during the supercontraction process, the spider dragline silk clearly became softer, i.e. both the Young's modulus and breaking stress decreased, while the breaking strain increased (Fig. 3 and Table 4). The mechanical properties of the spider dragline silk after 70% constrained supercontraction were close to the data after free supercontraction.
Relationship between microscopic structures and macroscopic mechanical properties of spider dragline silk after supercontraction
As per the discussed results, it can be concluded that the β-sheet in the ordered region aligned extremely well along the fiber axis in the original spider dragline silk (Smol = 0.828), which gave the silk excellent strength (high breaking stress and Young's modulus). Meanwhile, the orientation of the helix and random coil in the amorphous region was extremely poor (Smol = 0.133 and 0.069), which endowed the spider dragline silk a considerable extensibility (breaking strain). These properties make spider dragline silk a good example for the excellent balance of breaking stress (strength) and breaking strain (extensibility) with an extremely high breaking energy (toughness).
For spider dragline silk after free contraction, it is easy to understand that both the breaking stress and Young's modulus decreased by a large amount (only about half of the original value) because the molecular chains were almost randomly oriented. On the other hand, the apparent breaking strain was quite large (117%), but when considered after free contraction, the spider dragline silk shrank to 55% of its original length, hence the normalized breaking strain on the basis of original length was approximately 19%. This is probably because after free contraction, the β-sheet content in the spider dragline silk increased (from 16.1% to 21.2%), which made the spider silk stiffer.
Although the microscopic structure changed quite significantly between the original spider dragline silk and the one stretched after free contraction to its original length (for instance β-sheet content increased from 16.1% to 24.1%, while its Smol decreased from 0.828 to 0.490), the breaking stress, Young's modulus, and breaking strain almost remained the same. This result implies that the β-sheet content and the orientation degree of molecular chain in spider dragline silk have a synergistic effect on the final mechanical properties. Also, as shown in Fig. 3, the yield stress and strain increased from 0.2 to 0.6 GPa and 4% to 10%, respectively. This can be explained by the increase in the β-sheet content, but decrease in its orientation degree, which sustained more stretching until the molecular chains slipped from each other. On the other hand, compared to the free supercontraction, the orientation degree of all the conformation components increased after re-stretching. This led to the results that the orientation degree of the β-sheet component was still lower than that in the original counterpart, while the random coil and helical components were much higher than those in the original spider dragline silk. In other words, although the post-draw in the artificial spinning process can efficiently improve the orientation of all the molecular chains in the regenerated silk fiber,49,50 it still cannot mimic the natural spinning process, wherein, the β-sheet component is extremely well-aligned along the fiber axis while the random coil and helical components are poorly oriented, providing natural animal silks with an excellent combination of strength and extensibility. Therefore, there is still an obstacle between the current artificial spinning methods and the natural spinning process, encumbering the improvement of the mechanical properties of the regenerated silk fibers.
The biological function of supercontraction of spider dragline silk is still under debate.39,70,71 However, it is certain that if the supercontraction happens in nature, it should happen in constrained conditions. The results shown above indicate that for a 100% constrained supercontraction, the microscopic structure of the spider dragline silk changed significantly except for the β-sheet content. However, the mechanical properties remained almost unchanged as compared to the original spider dragline silk. For 90% constrained supercontraction spider dragline silk, the mechanical properties were also similar to those of the original and 100% constrained supercontraction spider silks (the apparent breaking strain was 61%, but when normalized to its original length, it was 45%). Therefore, we can conclude that the supercontraction of spider dragline silk actually has a little effect on its mechanical properties when the length of the spider silk is unchanged or changes a little (<10%). This conclusion is actually in accord with spider webs in nature, i.e., when accounting for rain or moisture, supercontraction happens. Although the microscopic structure may change after supercontraction, the mechanical properties of the spider web remain unchanged to maintain its functionality.
Conclusions
In this study, the content and orientation degree of different conformations in spider dragline silk after different supercontraction treatments have been extensively investigated using S-FTIR microspectroscopy. The mechanical properties of these different supercontracted spider dragline silks were also tested in order to establish the relationship between the microscopic molecular structures and macroscopic mechanical properties. These results show that the content and orientation degree of different conformations in spider dragline silk generally all changed no matter which kind of supercontraction has been performed. Specifically, for the important β-sheet structures, its content either remained almost unchanged (100% and 90% constrained supercontraction) or increased (free supercontraction, stretched to its original length after free supercontraction, and 70% constrained supercontraction), but its orientation degrees were all decreased. Considering the mechanical properties, the supercontracted spider dragline silks with similar lengths to the original ones (100% and 90% constrained supercontraction as well as stretched to its original length after free supercontraction) also displayed similar mechanical properties to the original ones. This phenomenon may reveal the mechanism behind how spiders maintain the function of the web via keeping the mechanical properties of the dragline silk constant by adjusting the molecular structure when rain or moisture is encountered in nature.
Another important conclusion that can be drawn from this study is that manual stretching improved the orientation degree of all the conformations (β-sheet, helix, and random) in spider dragline silk. However, it is not an ideal situation that natural animal silks possess excellent comprehensive mechanical properties, wherein, the β-sheet component is highly aligned along the fiber axis while the random coil and helical components are poorly oriented. Therefore, although the current post-draw procedure in artificial spinning processes can enhance the mechanical properties of regenerated silk fibers to a certain extent, it still cannot totally mimic the miraculous structure of the animal silks in nature and subsequently obtain the regenerated silk fibers with an extraordinary portfolio of mechanical properties close to its natural counterparts.
Conflicts of interest
There are no conflicts to declare.
Acknowledgements
This work was supported by the National Natural Science Foundation of China (No. 21574023, 21574024, 51973116, U1832109, and 21935002). We thank Prof. Min Chen, Dr Yuzhao Tang, Ms Jiajia Zhong, and Dr Xiaojie Zhou at Beamline BL01B1, Shanghai Synchrotron Radiation Facility (SSRF); Prof. Zeming Qi at Beamline U4, National Synchrotron Radiation Laboratory (NSRL), for assistance during data collection. We thank Dr Yuhong Yang at Fudan University for her valuable suggestions and discussions.
References
- Y. Wu, D. U. Shah, B. Wang, J. Liu, X. Ren, M. H. Ramage and O. A. Scherman, Adv. Mater., 2018, 30, e1707169 CrossRef.
- D. Kang, P. V. Pikhitsa, Y. W. Choi, C. Lee, S. S. Shin, L. F. Piao, B. Park, K. Y. Suh, T. I. Kim and M. Choi, Nature, 2014, 516, 222–226 CrossRef CAS.
- E. Filippidi, T. R. Cristiani, C. D. Eisenbach, J. H. Waite, J. N. Israelachvili, B. K. Ahn and M. T. Valentine, Science, 2017, 358, 502–505 CrossRef CAS.
- F. Vollrath and D. P. Knight, Nature, 2001, 410, 541–548 CrossRef CAS.
- C. Vepari and D. L. Kaplan, Prog. Polym. Sci., 2007, 32, 991–1007 CrossRef CAS.
- F. Vollrath and D. Porter, Polymer, 2009, 50, 5623–5632 CrossRef CAS.
- D. Ebrahimi, O. Tokareva, N. G. Rim, J. Y. Wong, D. L. Kaplan and M. J. Buehler, ACS Biomater. Sci. Eng., 2015, 1, 864–876 CrossRef CAS.
- M. Heim, D. Keerl and T. Scheibel, Angew. Chem., Int. Ed., 2009, 48, 3584–3596 CrossRef CAS.
- A. Rising and J. Johansson, Nat. Chem. Biol., 2015, 11, 309–3015 CrossRef CAS.
- N. Du, Z. Yang, X. Y. Liu, Y. Li and H. Y. Xu, Adv. Funct. Mater., 2011, 21, 772–778 CrossRef CAS.
- S. Kubik, Angew. Chem., Int. Ed., 2002, 41, 2721–2723 CrossRef CAS.
- G. Xu, L. Gong, Z. Yang and X. Y. Liu, Soft Matter, 2014, 10, 2116–2123 RSC.
- R. Ene, C. Krywka, S. G. Kang, P. Papadopoulos, M. Burghammer, E. Di Cola, M. Muller and F. Kremer, Polymer, 2012, 53, 5507–5512 CrossRef CAS.
- D. T. Grubb and L. W. Jelinski, Macromolecules, 1997, 30, 2860–2867 CrossRef CAS.
- S. Sampath, T. Isdebski, J. E. Jenkins, J. V. Ayon, R. W. Henning, J. P. Orgel, O. Antipoa and J. L. Yarger, Soft Matter, 2012, 8, 6713–6722 RSC.
- C. Riekel, M. Müller and F. Vollrath, Macromolecules, 1999, 32, 4464–4466 CrossRef CAS.
- R. W. Work, Text. Res. J., 1977, 47, 650–662 CrossRef.
- R. W. Work, Text. Res. J., 1982, 52, 349–356 CrossRef CAS.
- Z. Z. Shao and F. Vollrath, Polymer, 1999, 40, 1799–1806 CrossRef CAS.
- R. Ene, P. Papadopoulos and F. Kremer, Polymer, 2010, 51, 4784–4789 CrossRef CAS.
- X. Li, P. T. Eles and C. A. Michal, Biomacromolecules, 2009, 10, 1270–1275 CrossRef CAS PubMed.
- S. Sampath and J. L. Yarger, RSC Adv., 2015, 5, 1462–1473 RSC.
- R. Ene, P. Papadopoulos and F. Kremer, Soft Matter, 2009, 5, 4568–4574 RSC.
- Z. Z. Shao, F. Vollrath, J. Sirichaisit and R. J. Young, Polymer, 1999, 40, 2493–2500 CrossRef CAS.
- Z. Z. Shao, R. J. Young and F. Vollrath, Int. J. Biol. Macromol., 1999, 24, 295–300 CrossRef CAS PubMed.
- T. Giesa, R. Schuetz, P. Fratzl, M. J. Buehler and A. Masic, ACS Nano, 2017, 11, 9750–9758 CrossRef CAS.
- J. Dionne, T. Lefèvre, P. Bilodeau, M. Lamarre and M. Auger, Phys. Chem. Chem. Phys., 2017, 19, 31487–31498 RSC.
- D. T. Grubb and G. Ji, Int. J. Biol. Macromol., 1999, 24, 203–210 CrossRef CAS.
- J. E. Jenkins, S. Sampath, E. Butler, J. Kim, R. W. Henning, G. P. Holland and J. L. Yarger, Biomacromolecules, 2013, 14, 3472–3483 CrossRef CAS.
- Z. T. Yang, O. Liivak, A. Seidel, G. L. Verde, D. B. Zax and L. W. Jelinski, J. Am. Chem. Soc., 2000, 1222, 9019–9025 CrossRef.
- G. P. Holland, R. V. Lewis and J. L. Yarger, J. Am. Chem. Soc., 2003, 126, 5867–5872 CrossRef.
- A. H. Simmons, C. A. Michal and L. W. Jelinski, Science, 1996, 271, 84–87 CrossRef CAS.
- J. D. van Beek, S. Hess, F. Vollrath and B. H. Meier, Proc. Natl. Acad. Sci. U. S. A., 2002, 99, 10266–10271 CrossRef CAS.
- P. T. Eles and C. A. Michal, Macromolecules, 2004, 37, 1342–1345 CrossRef CAS.
- R. E. Fornes, R. W. Work and N. Morosoff, J. Polym. Sci., Polym. Phys. Ed., 1983, 21, 1163–1172 CrossRef CAS.
- A. Simmons, E. Ray and L. W. Jelinski, Macromolecules, 1994, 27, 5237 CrossRef.
- Y. Liu, Z. Z. Shao and F. Vollrath, Chem. Commun., 2005, 2489–2491 RSC.
- F. Vollrath and D. Porter, Soft Matter, 2006, 2, 377–385 RSC.
- Y. Liu, Z. Z. Shao and F. Vollrath, Nat. Mater., 2005, 4, 901–905 CrossRef CAS.
- Y. Liu, A. Sponner, D. Porter and F. Vollrath, Biomacromolecules, 2008, 9, 116–121 CrossRef CAS.
- C. J. Fu, D. Porter, X. Chen, F. Vollrath and Z. Z. Shao, Adv. Funct. Mater., 2011, 21, 729–737 CrossRef CAS.
- G. V. Guinea, M. Elices, J. Pérez-Rigueiro and G. R. Plaza, J. Exp. Biol., 2005, 208, 25–30 CrossRef CAS.
- A. Schäfer, T. Vehoff, A. Glišović and T. Salditt, Eur. Biophys. J. Biophys., 2008, 37, 197–204 CrossRef.
- T. Vehoff, A. Glišović, H. Schollmeyer, A. Zippelius and T. Salditt, Biophys. J., 2007, 93, 4425–4432 CrossRef CAS.
- M. Jackson and H. H. Mantsch, Crit. Rev. Biochem. Mol. Biol., 1995, 30, 95–120 CrossRef CAS.
- A. Barth and C. Zscherp, Q. Rev. Biophys., 2002, 35, 369–430 CrossRef CAS.
- S. J. Ling, Z. M. Qi, D. P. Knight, Z. Z. Shao and X. Chen, Biomacromolecules, 2011, 12, 3344–3349 CrossRef CAS.
- S. J. Ling, Z. M. Qi, D. P. Knight, Y. Huang, L. Huang, H. Zhou, Z. Z. Shao and X. Chen, Biomacromolecules, 2013, 14, 1885–1892 CrossRef CAS.
- G. Q. Fang, Z. K. Zheng, J. R. Yao, M. Chen, Y. Z. Tang, J. J. Zhong, Z. M. Qi, Z. Li, Z. Z. Shao and X. Chen, J. Mater. Chem. B, 2015, 3, 3940–3947 RSC.
- G. Q. Fang, Y. F. Huang, Y. Z. Tang, Z. M. Qi, J. Yao, Z. Z. Shao and X. Chen, ACS Biomater. Sci. Eng., 2016, 2, 1992–2000 CrossRef CAS.
- G. Q. Fang, Y. Z. Tang, Z. M. Qi, J. R. Yao, Z. Z. Shao and X. Chen, J. Mater. Chem. B, 2017, 5, 6042–6048 RSC.
- G. Q. Fang, S. Sapru, S. Behera, J. R. Yao, Z. Z. Shao, S. C. Kundu and X. Chen, J. Mater. Chem. B, 2016, 4, 4337–4347 RSC.
- G. R. Plaza, G. V. Guinea, J. Pérez-Rigueiro and M. Elices, J. Polym. Sci. Polym. Phys., 2006, 44, 994–999 CrossRef CAS.
- J. L. Yarger, B. R. Cherry and A. van der Vaart, Nat. Rev. Mater., 2018, 3, 8000 Search PubMed.
- J. M. Gosline, M. W. Denny and M. E. DeMont, Nature, 1984, 309, 551–552 CrossRef CAS.
- T. A. Blackledge, C. Boutry, S. C. Wong, A. Baji, A. Dhinojwala, V. Sahni and I. Agnarsson, J. Exp. Biol., 2009, 212, 1981–1989 CrossRef.
- A. Seidel, O. Liivak and L. W. Jelinski, Macromolecules, 1998, 31, 6733–6736 CrossRef CAS.
- K. Yazawa, K. Ishida, H. Masunaga, T. Hikima and K. Numata, Biomacromolecules, 2016, 17, 1057–1066 CrossRef CAS.
- J. Guan, D. Porter and F. Vollrath, Biomacromolecules, 2013, 14, 930–937 CrossRef CAS.
- C. Mo, P. Wu, X. Chen and Z. Z. Shao, Vib. Spectrosc., 2009, 51, 105–109 CrossRef CAS.
- X. Hu, D. Kaplan and P. Cebe, Thermochim. Acta, 2007, 461, 137–144 CrossRef CAS.
- Y. S. Kim, L. M. Dong, M. A. Hickner, T. E. Glass, V. Webb and J. E. McGrath, Macromolecules, 2003, 36, 6281–6285 CrossRef CAS.
- Y. Wang, J. C. Wen, B. Peng, B. W. Hu, X. Chen and Z. Z. Shao, Biomacromolecules, 2018, 19, 1999–2006 CrossRef CAS.
- T. Lefèvre, M. E. Rousseau and M. Pézolet, Biophys. J., 2007, 92, 2885–2895 CrossRef.
- F. Paquet-Mercier, T. Lefèvre, M. Auger and M. Pézolet, Soft Matter, 2013, 9, 208–215 RSC.
- Y. Wang, J. Guo, L. Zhou, C. Ye, F. G. Omenetto, D. L. Kaplan and S. J. Ling, Adv. Funct. Mater., 2018, 28, 1805305 CrossRef.
- J. J. Zhong, Y. W. Liu, J. Ren, Y. Z. Tang, Z. M. Qi, X. J. Zhou, X. Chen, Z. Z. Shao, M. Chen, D. L. Kaplan and S. J. Ling, ACS Biomater. Sci. Eng., 2019, 5, 3161–3183 CrossRef CAS.
- K. N. Sacage, P. A. Guerette and J. M. Gosline, Biomacromolecules, 2004, 5, 675–679 CrossRef.
- F. I. Bell, I. J. McEwen and C. Viney, Nature, 2002, 416, 37 CrossRef CAS.
- M. Elices, G. R. Plaza, J. Pérez-Rigueiro and G. V. Guinea, J. Mech. Behav. Biomed., 2011, 4, 658–669 CrossRef.
- C. Boutry and T. A. Blackledge, J. Exp. Biol., 2010, 213, 3505–3514 CrossRef.
Footnotes |
† Electronic supplementary information (ESI) available. See DOI: 10.1039/c9tb02032b |
‡ These authors contributed equally to this work. |
|
This journal is © The Royal Society of Chemistry 2020 |