DOI:
10.1039/C9TB01870K
(Review Article)
J. Mater. Chem. B, 2020,
8, 9-17
DNA-templated quantum dots and their applications in biosensors, bioimaging, and therapy
Received
30th August 2019
, Accepted 12th November 2019
First published on 13th November 2019
Abstract
Over the past 10 years, DNA functionalized quantum dots (QDs) have attracted considerable attention in sensing and imaging of disease-relevant biological targets, as well as cancer therapy. Considerable efforts have been devoted to obtaining DNA functionalized QDs with enhanced stability and quantum yield. Here, we focus on a one-pot method, in which phosphorothioate-modified DNA is used as the co-ligand on the basis of the strong binding of sulfur and Cd2+. After a short summary of the preparation of DNA-templated QDs, versatile bioapplications based on the constructed ratiometric fluorescent probes, nanobeacons and multiple bottom-up assemblies will be discussed. A substantial part of the review will focus on these applications, ranging from small molecule, biological macromolecule, cancer cell and pathogen sensing to in vitro and in vivo imaging. Besides, drug or siRNA delivery based on DNA-templated QD assemblies will also be briefly discussed here.
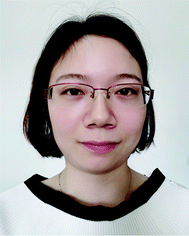
Yeling Yang
| Yeling Yang is working for her PhD degree from College of Chemistry and Molecular Sciences of Wuhan University in 2017. Currently, she is working as a joint PhD candidate in Wuhan University. Her research interests focus on the synthesis of bioinspired nanomaterials and application in biosensing and imaging. |
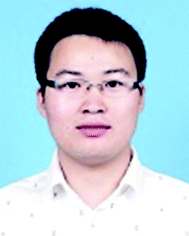
Guobin Mao
| Guobin Mao obtained his PhD degree in Analytical Chemistry from Wuhan University in 2019. He is currently a postdoctoral researcher at Shenzhen Institutes of Advanced Technology, Chinese Academy of Sciences. His research interests are focused on the development of fluorescent nanomaterials, and using these for biosensors and bioimaging. |
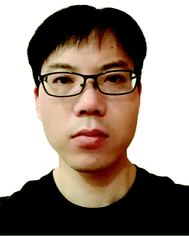
Xinghu Ji
| Xinghu Ji received his PhD degree in Analytical Chemistry from Wuhan University in 2007. In 2014, he worked at University of Kansas as a visiting scholar. He is currently an associate professor at Wuhan University. His research interests focus on microfluidics and applications in analytical chemistry for biology and medicine. |
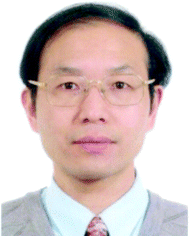
Zhike He
| Zhike He is Outstanding Professor of Chemistry College of Chemistry and Molecular Science, Wuhan University. He received his PhD degree in Analytical Chemistry from Wuhan University in 1995. He was also a Humboldt Research Fellow. Currently, his research interests cover nano-bio-analytical chemistry and applications in biology and medicine. |
1. Introduction
Colloidal quantum dots (QDs) are a class of quasi-zero-dimensional fluorescence nanocrystals with excellent properties, such as broad excitation spectra, narrow emission peaks, size-dependent spectra, good photo-stability, and easy functionalization.1 The methods to prepare QDs mainly include synthesis in the organic phase and aqueous phase. The QDs obtained from the organic phase have uniform particle size, high quantum yield and good stability, however a phase transfer process is necessary for bioanalytical applications.2,3 The QDs prepared in the aqueous phase are environment-friendly, easy to surface functionalize, and can be directly used in biological applications.4–6 Alivisatos7 and Nie8et al. firstly modified biomolecules on the surface of QDs and applied them to bioimaging in 1998, which is the pioneering report about biomedical applications of QDs. Now, a variety of biomolecules, such as peptides,9–11 nucleic acids,12–15 proteins16,17 and small molecules,18 are often applied for QD functionalization to further broaden their applications in bioanalysis.
DNA, which has small size, great stability, and low prices and can be easily modified, has attracted substantial attention in recent years. Quantum dot–DNA bioconjugates have low nonspecific binding and can assemble with other DNA functionalized nanomaterials through base-pairing.19–22 These higher order assemblies have been proven to be powerful tools for intracellular miRNA imaging,19 cancer cell detection,20,21 and drug23 and siRNA24 delivery. In addition, there is a special type of DNA named aptamers, which are oligonucleotide fragments obtained by a procedure of Systematic Evolution of Ligands by Exponential Enrichment (SELEX).25 Aptamer functionalized QDs can specifically interact with target molecules, including ions,26 small molecules27 and proteins,28 which greatly expands their applications. In summary, because of the high affinity to target molecules, low nonspecific binding and predictable programmability, DNA functionalized QDs are regarded as powerful tools for biosensors, bioimaging, and therapy. The methods for construction of DNA functionalized QDs mainly include electrostatic adsorption,29 covalent coupling,30 and metal–ligand interaction.31 Qiu et al. utilized lysozyme (Lys) to coat QDs, which gave the QDs positive charge, and then a sensor based on FRET from the QDs to 6-carboxy-x-rhodamine (Rox) was constructed through electrostatic adsorption between Lys-QDs with positive charge and DNA-Rox with negative charge.29 The method featured high simplicity and flexibility, however the electrostatic adsorption easily resulted in high non-specific binding. The covalent coupling method can effectively conjugate DNA onto QDs, however the amount of DNA modified on the surface of QDs is difficult to control, which is not conducive to sensitive biomolecule detection.32 Kelley's group systematically studied the role of different bases in QD synthesis and proposed a novel method for preparation of DNA functionalized QDs using phosphorothioate-modified DNA as a co-ligand.33 The DNA functionalized QDs can be prepared via a one-pot method without any chemical modification, thereby avoiding the decrease of the quantum yield and obtaining stability in the modification process. In addition, the amount of DNA on the surface of the QDs can be precisely controlled mainly by changing the number of phosphorothioate groups.34
Due to the good properties of QDs and the programmability of DNA, DNA functionalized QDs were widely applied for bioapplications including biosensors, bioimaging, and therapy.35 For biosensors, one of the detection principles is based on the breaking of fluorescence resonance energy transfer (FRET) between QDs and quenchers that include graphene oxide (GO),36 gold nanoparticles,37 and BHQ.31 The other principle is based on fluorescence enhancement or quenching through the interaction of QDs and targets. Our group constructed a QD-based ratiometric fluorescent probe using Rox and phosphorothioate co-modified DNA as the template.38 Due to the reference of Rox, this probe exhibited good potential for simple, rapid, and effective visual detection. For bioimaging, one of the principles is based on the specific interaction between an aptamer and acceptor protein. DNA functionalized QDs and the aptamer, which assembled into long double stranded DNA, were used to specifically target a biomarker of cancer cells.28 This method can achieve high imaging sensitivity due to the DNA-programmed hybridization chain reaction. The other principle, which is mainly used for nucleic acid imaging or enzyme activation monitoring, is breaking the FRET between QDs and a quencher through DNA hybridization or enzyme cleavage.39 For therapy, self-assembled DNA functionalized QDs were used as a nanocarrier.23,24 After binding with the target, the anti-cancer drug would be released and the fluorescence of the QDs can be used for drug deliver and therapy effect monitoring.23
2. The preparation of DNA-templated quantum dots
A variety of methods for conjugating DNA onto QDs, such as covalent conjugation, electrostatic interaction, and direct dative interactions etc., have been reported in recent years.40–42 However, covalent conjugation, which uses reagents of EDC/NHS chemistry, may somewhat diminish the quantum yield, reduce the colloidal stability and give rise to uncontrolled valence.32,42 Electrostatic interaction, which is based on the opposite charges of the biomolecules and QDs, is usually nonspecific.42 Dative bonds, such as the bonds between monothiols and QDs, can be easily broken because of oxidation and high temperature, which resulted in particle aggregation and fluorescence quenching.43 Kelley's group first took advantage of multidentate phosphorothioated DNA as templates to direct CdTe QD growth.33 The template DNA included three parts, a natural phosphate (po) domain for biotargeting, a linker domain as a spacer and a phosphorothioate (ps) domain which has high affinity with Cd2+ for in situ growth of QDs (Fig. 1).35 Compared with traditional modification methods, biotemplated synthesis of QDs can directly attach DNA to nascent nano-particles, strengthen the dative bonds, improve the thermal stability and facilely regulate the valencies.43 In this section we mainly focus on the preparation of bioconjugating QDs directly using DNA as templates.
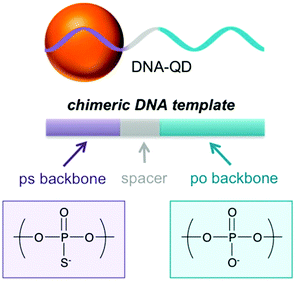 |
| Fig. 1 Schematic illustration of DNA-QDs. Chemical structure of the phosphorothioate linkage (purple) and phosphate linkage (blue) of the DNA template for QD synthesis. Reprinted with permission from ref. 35. Copyright 2018 American Chemical Society. | |
2.1 Two-step method
The two-step method, taking the most popular Cd-based QDs as a model, usually uses a Cd precursor and NaHTe. The Cd precursor was prepared by dissolving CdCl2 and ligands in ultrapure water. NaHTe was obtained through stirring a mixture of Te powder and NaBH4 under oxygen-free conditions and in an ice-bath for about 5 h. Ma et al. have previously shown that a mixture of CdCl2-GSH stock solution, freshly prepared NaHTe solution and phosphorothioate modified DNA can be used for the DNA-programmed growth of CdTe QDs.33 It should be noted that Cd-containing QDs are controversial because of the cytotoxicity.44,45 Zhang et al. proposed the preparation of DNA functionalized Zn2+ doped CdTe QDs, which have high quantum yield, low cytotoxicity, and wide application in biological sensing and imaging.46 However, the process of reducing Te powder is complicated and time-consuming.
2.2 One-step method
Zhang et al. proposed a one-step method to facilely prepare DNA-CdTe: Zn2+ QDs under air conditions.47 They firstly mixed CdCl2, ZnCl2, N-acetyl-L-cysteine (NAC) and ultrapure water and adjusted the pH to 9. Then Na2TeO3 and NaBH4 were added to the obtained precursor. However, many by-products may be easily generated from NaBH4 during reduction, and the precursor solution must be freshly prepared before use. Mao et al. developed a novel method for preparing stable CdZnTeS QDs by employing meso-2,3-dimercaptosuccinic acid (DMSA) as a S source and reducing reagent.38 The CdZnTeS QDs exhibit high quantum yield, good biocompatibility and enhanced stability under low pH and high salt concentrations due to using both DMSA and NAC as ligands.48
3. DNA-templated quantum dots for biosensors
Versatile applications of DNA-templated QDs have been continuously developed since this synthesis was pioneered by the Kelley group in 2009.35,41,49 Here, we mainly review the literature that established QD-bioconjugates as a new class of agents for small molecule, nucleic acid, protein, cancer cell and pathogen detection.
3.1 Detection of small molecules
Small molecules such as systemin, hydrogen peroxide, dopamine etc. play a vital role as physiological indicators of animals or plants. Liu et al. reported the detection of tomato systemin by combining graphene oxide (GO) and aptamer-functionalized CdTe:Zn2+ QDs (Fig. 2).27 The aptamer-functionalized QDs and the GO were brought together through π–π stacking. The fluorescence of the aptamer functionalized QDs was quenched by GO, and recovered after adding systemin. Accordingly, this detection method can also be extended to the detection of DNA, proteins and other substances, as long as the appropriate aptamer was selected from the DNA library.50,51
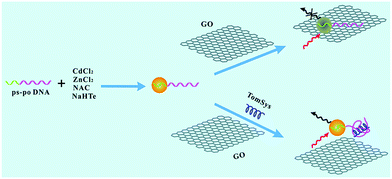 |
| Fig. 2 Schematic representation of the designed aptasensor for the detection of tomato systemin. Reproduced from ref. 27 with permission from the Royal Society of Chemistry. | |
It should be noted that single emission fluorescent probes have inherent limits. The quantification of the target analyte may be affected by multifarious factors such as environmental interference, instrumental parameters, and fluorescent probe concentration, etc.52 Conversely, ratiometric fluorescent probes, which are based on analyte-induced alterations in the ratio of the intensity of two emission bands, are less prone to be disturbed by the above problems. Mao et al. utilized phosphorothioate and Rox co-modified DNA to construct a ratiometric fluorescent probe, named Rox-DNA functionalized CdZnTeS QDs (Fig. 3).38 Compared with constructing a dual-emission probe by covalent coupling or direct mixing, this approach avoids chemical modification and complicated separation, and the ratiometric fluorescent probe synthesized in this manner is specifically sensitive to hydrogen peroxide (H2O2). H2O2, which is generated by oxidation of glucose, can quench the CdZnTeS QDs through a redox reaction, while the red fluorescence of Rox is invariable. The proposed probe has a detection limit of 0.075 μM for H2O2 and 0.042 μM for glucose. As the glucose concentration increased, the fluorescence color of the probe will show a transition from green to red under ultraviolet light irradiation, which can be used for the visual detection of blood glucose. In addition, this ratiometric fluorescent probe can also be used in the detection of dopamine (DA). DA can easily be oxidized to form dopamine quinone. Dopamine quinone can quench quantum dots and not affect the fluorescence of Rox, which can be applied for accurate dopamine detection in blood samples.53 The linear correlation was in the range of 10.0–1000.0 nM and the limit of detection (LOD) was estimated to be as low as 1.93 nM for DA.
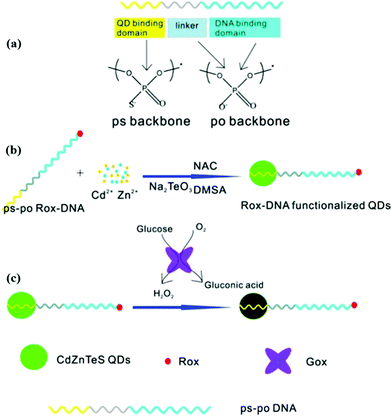 |
| Fig. 3 Schematic description of (a) the structure of ps-po DNA, (b) preparation of Rox-DNA functionalized CdZnTeS QDs, and (c) H2O2 detection based on Rox-DNA functionalized CdZnTeS QDs. Reprinted with permission from ref. 38. Copyright 2017 American Chemical Society. | |
3.2 Detection of nucleic acids
Nucleic acids participate in a series of major life activities such as growth, inheritance and mutation, and play a vital role in cell mutation and viral infection. So it is considerably important to simply and sensitively detect nucleic acids. Zhang et al. synthesized DNA functionalized QDs which can be used to detect hepatitis B virus (HBV) DNA.36 The measured linear range was 50–1600 nM. The LOD for HBV DNA detection was 10.4 nM. The sensor is formed based on the different affinity of GO to double-stranded DNA and single-stranded DNA, and the HBV DNA detection is based on the breaking of FRET from the QDs to GO. However, proteins, which have non-specific interactions with GO, can bring about a false positive result in this system. Compared with DNA detection, the detection of microRNAs has attracted more attention in recent years, because microRNA regulates gene expression and it is closely related to many human diseases such as cancer.54–56 However, due to the particularly low concentration of microRNAs in living cells, it is vital to construct sensors with high sensitivity. Up to now, various signal-amplification strategies have been developed by researchers, but here we mainly focus on nanomaterial based amplification for microRNA detection.57 Since AuNPs were modifided with oligonucleotides by the two groups of Mirkin–Letsinger58 and Alivisatos–Schultz,59 DNA functionalized AuNPs and multifarious assemblies have been widely applied in the construction of fluorescence biosensors60,61 and electrochemical biosensors62 due to efficient fluorescence quenching or electron transfer. Ma's group developed a fluorescence platform for microRNA sensitive detection by engineered conjugates of AuNP–oligonucleotide and DNA functionalized QDs to construct a DNA-programmed core–satellite biosensor (Fig. 4).63 By regulating the distance, the QDs can be quenched by the AuNPs through FRET. However, with the participation of designed fuel DNA that can trigger the disassembly of multiple QDs, low-abundance microRNA can be monitored with a three orders of magnitude improvement by the core–satellite probe. Three types of cancer cell lines (HeLa, MCF-7, and MDA-MB-231) that overexpressed miRNA-21 were examined in this manner. The copy numbers of target miRNA per pg of total RNA in the three types of cancer cells were calculated to be 323 ± 28, 746 ± 44, 706 ± 45, respectively.
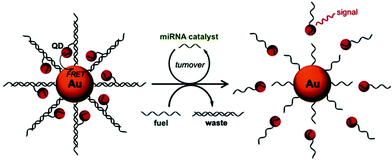 |
| Fig. 4 Illustration of the GNP–QD nanoassembly for catalytic miRNA sensing. The miRNA molecule serves as a catalyst to disassemble QDs from the GNP with the aid of fuel DNA strands. Reprinted with permission from ref. 63. Copyright 2015 Wiley. | |
3.3 Detection of proteins
Proteins are the main participant in approximately all life activities, and are deemed as a noteworthy indicator of physiological health. Abnormal protein levels may forebode a life-threatening problem and induce illness, so the precise determination of proteins is considerably significant.64 For protein detection, one of the principles is based on the breaking of FRET between QDs and a quencher. Liu et al. modified fiber paper with cytochrome C, which can quench QDs by photo-induced electron transfer.65 Combining the nanopaper with aptamer functionalized CdTe:Zn2+ QDs, they can realize the immediate detection of MUC1. The LOD of MUC1 was estimated to be as low as 10 nM with the proposed platform. Another detection principle is on the basis of fluorescence enhancement or quenching through the interaction between QDs and a protein. Based on this principle, Mao et al. developed a series of methods for the detection of protamine, trypsin, tyrosinase, and telomerase by a ratiometric fluorescent probe. Protamine is a cationic charged linear protein, which can directly quench QDs owing to the aggregation of the probe. Trypsin is a digestive enzyme that can hydrolyze protamine and restore the fluorescence of QDs. Due to the reference of Rox, this probe exhibits good potential for facile, accurate, and effective visual detection of two proteins.66 A good linear relationship (2.5–50 ng mL−1 for trypsin and 25–300 ng mL−1 for protamine) with a low limit of detection (0.87 ng mL−1 for trypsin and 4.73 ng mL−1 for protamine) can be obtained in this manner. Besides, telomerase activity can be detected with the Rox-DNA functionalized QDs.67 The QDs can be quenched by hydrogen peroxide, which is proved to be the substrate of the G-quadruplex catalytic reaction. However, the long DNA chain, which is extended by telomerase, can form a G-quadruplex with hemin, and catalyze the hydrolysis of hydrogen peroxide. The LOD was 10 cells, and visual detection of telomerase activity can be carried out with the probe-based paper sensor in bladder cancer patients’ urine. Furthermore, tyrosinase (TYR) catalyzes the formation of dopamine quinone by oxidizing dopamine. Therefore, the probe can also be used to detect TYR because quantum dots can be quenched by the products of dopamine oxidation (Fig. 5).53 The probe exhibits a strong linear correlation in the range of 10.0–100.0 ng mL−1 and the limit of detection was as low as 1.05 ng mL−1 for TYR. It can also be applied for on-site and accurate detection of tyrosinase in human serum by the naked eye.
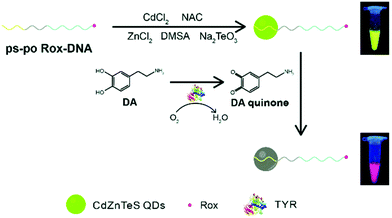 |
| Fig. 5 Schematic illustration of the route of one-pot synthesis of Rox-DNA-functionalized QDs and the principle of the novel biosensor for the detection of DA and TYR based on Rox-DNA-functionalized CdZnTeS QDs. Reproduced from ref. 53 with permission from the Royal Society of Chemistry. | |
3.4 Detection of cells and pathogens
Circulating tumor cells (CTCs), which invade the human peripheral blood from tumors, play a critical role in understanding the progression of metastasis, diagnosis, and treatment. However, CTCs are extremely rare in the blood and, therefore, tremendous research effort has been dedicated to seeking a variety of techniques to isolate and detect them.68–70 Among those methods, high affinity ligands such as antibodies and aptamers improved the sensitivity, yield, and purity of cancer cell capture.71–73 Zheng et al. developed an electrochemical biosensing strategy for green and ultrasensitive determination of tumor cells based on combining aptamer-DNA concatamer-CdTe QD probes with mercury-free anodic stripping voltammetry.74 The proposed electrochemical biosensor for K562 cells exhibited high sensitivity with a wide linear range from 1.0 × 102 to 1.0 × 107 cells per mL and the LOD was 60 cells per mL. Ding et al. constructed a near-infrared (NIR) silver sulfide (Ag2S) nanoassembly with DNA-labeled Ag2S QDs, a long DNA chain formed by a hybridization chain reaction (HCR), and aptamers that specifically recognize MUC1.20 The biologically penetrating nanoassembly co-operating with immune-magnetic spheres exhibits excellent performance in zebrafish tumor imaging and can discriminate as low as 6 MCF-7 cells in clinical samples. Li et al. utilized the aptamer sgc8c, DNA-labeled QDs, and DNA modified magnetic nanospheres to assemble a three-dimensional material through HCR (Fig. 6).21 The material has both a specific recognition capacity and amplified magnetic separation function, thereby it could facilely isolate and enumerate low abundance CTCs from human blood samples in 20 min. A specific interaction between a membrane protein and aptamer can also be applied for pathogen detection. Liu et al. used cytochrome C modified nanopaper, which, combined with aptamer functionalized QDs, gave access to point-of-care testing of E. coli O157:H7.65 The nanopaper-based platform was demonstrated to exhibit a highly sensitive response to E. coli O157:H7 with a wide detection range from 102 to 106 CFU mL−1.
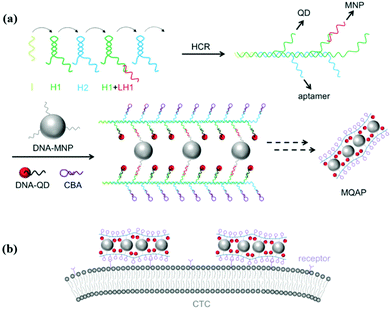 |
| Fig. 6 Schematic illustration of MQAPs for magnetic isolation of CTCs. (a) Construction of MQAPs in two steps: (i) hybridization chain reaction mediated formation of the polymeric DNA template and (ii) co-assembly of DNA-QDs, DNA-MNPs, and aptamers with the DNA template. (b) Multivalent binding between MQAPs and cell surface receptors for CTC isolation and detection. Reprinted with permission from ref. 21. Copyright 2018 Wiley. | |
4. DNA-templated quantum dots for bioimaging
QD related research articles have gone through a period of exponential increase since the advantages of these materials for biosensing were pointed out in 1998,7,8 and versatile bioconjugated QDs were exploited for in vitro and in vivo imaging.75 However, we focus on DNA-templated QDs here, which are regarded as promising imaging agents due to their unique photophysical properties, controlled valence and facile assembly.19,28,76
4.1
In vitro imaging
Li et al. employed enzyme-free HCR for QD-aptamer polymer (QAP) construction with QDs and cell-binding aptamers.28 Due to multivalent targeting and multiple QD-based signal amplification, QAPs offer high cell discrimination factors at low QD concentration, and further hold great promise as effective bioprobes for single cell imaging. Wei et al. reported a new type of DNA-templated heterobivalent QD nanoprobes, containing two main motifs for extracellular (nucleolin) and intracellular (microRNA) targeting and imaging, which are present on the cell surface and in the cell cytosol, respectively (Fig. 7).19 The QD probe could bypass the commonly existing lysosomal sequestration, and this approach opens up the possibility of intracellular biomolecule imaging. Ma et al. utilized BHQ1 and phosphorothioate comodified DNA directing CdTe:Zn2+ QD growth, and thereby developed a QD-based nanobeacon for intracellular single RNA imaging (Fig. 8).76 They have proved that the QD-based nanobeacon with just one conjugate DNA is more suitable and effective for single RNA imaging in live cells.
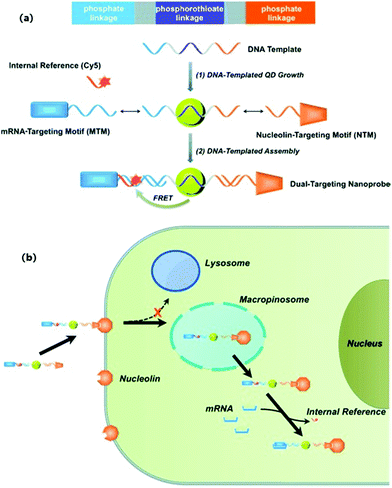 |
| Fig. 7 (a) Schematic illustration of the construction of a DNA-templated heterobivalent QD nanoprobe; (b) the dual-targeting strategy and intracellular delivery route of the heterobivalent QD nanoprobe. Reprinted with permission from ref. 19. Copyright 2014 Wiley. | |
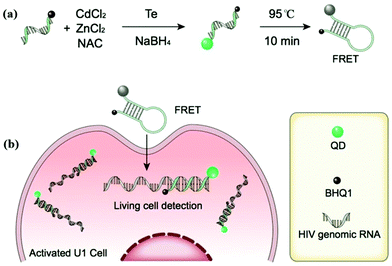 |
| Fig. 8 (a) Schematic diagram of the procedure for preparing QD-NBs; (b) schematic illustration of QD-NBs for HIV-1 genomic RNA detection in living cells. Reprinted with permission from ref. 76. Copyright 2019 American Chemical Society. | |
4.2
In vivo imaging
Based on the specific interaction between aptamer-functionalized CdTe:Zn2+ QDs and MUC1 on the surface of A549 cancer cells, Zhang et al. achieved in vitro imaging and injected aptamer-functionalized QDs into tumor-bearing mice for in vivo imaging. This is the first time that DNA functionalized quantum dots are used for in vitro and in vivo imaging, simultaneously.46
5. DNA-templated quantum dots for therapy
Cancer has overtaken heart disease to become the first cause of death worldwide. Fortunately, many methods based on nanomaterials and nanotechnology have been developed and continued, such as photodynamic therapy (PDT),77,78 photothermal therapy (PTT),79,80 gene therapy81 and chemotherapy,82 which have emerged as powerful cancer therapy technologies. DNA functionalized quantum dots were usually used as a probe for cancer diagnosis, monitoring of drug release, and imaging-guided therapy evaluation. Wang et al. reported a type of self-assembly based on DNA-templated gold nanoparticle (GNP)-quantum dot (QD) complexes (GQC) for photothermal (PT) imaging in vitro (Fig. 9).83 The photoluminescence of the QDs was quenched by GNP, and activated at elevated temperature, so the constructed nanoprobe can be used to monitor the PT effect with good spatial resolution. The DNA-templated GQC can be also utilized to identify and track cancer cells post-treatment for therapy evaluation. In addition, a series of studies reported by Ma's group and Kelley's group demonstrated that the self-assemblies based on DNA functionalized QDs can be used as a carrier of anti-cancer drugs.23,24,84 Luo et al. reported a novel class of microRNA-mediated drug release system based on a DNA programmed GNP–QD complex.23 The microRNA could specifically catalyze the disassembly of DNA programmed GNP and DNA programmed QDs, thus the fluorescence of the QDs was restored and doxorubicin was released for killing cancer cells. The activated fluorescence of QDs can provide reliable feedback for microRNA-catalyzed drug release. Zhang et al. developed self-assembled quantum dot DNA hydrogels, which are size-tunable with high quantum yield, low cytotoxicity, and good photo-stability (Fig. 10).24 The QD DNA hydrogels can load doxorubicin for chemotherapy or siRNA for gene therapy. Besides that, a loaded aptamer could specifically bind with a target protein, thereby synergistically enhancing the therapeutic efficacy. Therefore, the assembly based on DNA functionalized QDs can act as an excellent nanocarrier for specific binding and controlled drug release. For future directions, combining with other DNA functionalized nanoparticles, utilizing biosensing technology and spatiotemporal control of bio-active compound release in cellular or animal models should be further explored.
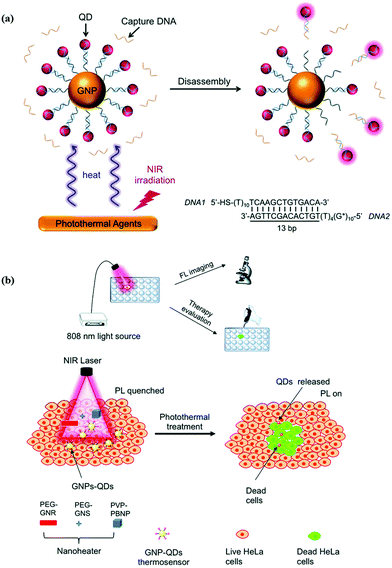 |
| Fig. 9 Schematic illustration of GQC for photothermal sensing and imaging. (a) Photothermal-triggered disassembly of GQC and activation of QD PL. (b) Photothermal imaging and labeling of cancer cells with GQC and a variety of nanoheaters. Reproduced from ref. 83 with permission from the Royal Society of Chemistry. | |
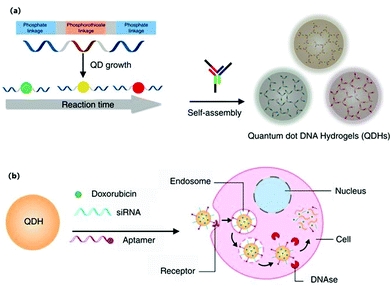 |
| Fig. 10 Schematic illustrations of synthesis of self-assembled QDHs, modification with therapeutic or targeting agents, and the cellular uptake mechanism. (a) Synthesis of DNA-functionalized QDs and the subsequent formation of QDH through hybridization with DNA three-way junction nanostructures. (b) Modifications of QDH for cell-specific targeting with an aptamer and siRNA/drug delivery. Doxorubicin and siRNA are released upon cellular uptake via endocytosis. Reprinted with permission from ref. 24. Copyright 2017 Springer Nature. | |
6. Conclusion
This review has summarized the recent preparation of biotemplated DNA functionalized QDs, and bioanalysis based on DNA functionalized QDs including biosensors, bioimaging, and cancer therapy. Since DNA functionalized QDs prepared via a one-pot method were developed in 2009, a lot of new methods have been proposed for preparing DNA functionalized QDs, which enhanced the stability, reduced the cytotoxicity and simplified the synthesis process. At the same time, DNA functionalized near-infrared QDs were also prepared and applied for bioimaging of cancer cells. Several fluorescence probes based on DNA functionalized QDs were constructed for better bioanalysis, such as ratiometric fluorescence probes, and QD-nanobeacons. In addition, many self-assemblies based on DNA functionalized QDs were formed for imaging of cancer cell membrane receptors and intracellular microRNA, monitoring of drug release, and cancer therapy.
However, many challenges still need to be faced. Firstly, DNA functionalized near-infrared QDs with high quantum yield and good stability should be prepared, which would provide good potential in in vivo bioapplications. Then, combining with other DNA nanotechnology, such as DNA origami, DNA hydrogels, and DNA functionalized nanoparticles, self-assemblies based on DNA functionalized QDs through DNA hybridization can be constructed for more extensive bioapplications. Finally, the valence of DNA functionalized QDs should be more precisely controlled and deeper applications should be explored. With this aim, we will continually contribute to the development of DNA functionalized QDs.
Conflicts of interest
There are no conflicts to declare.
Acknowledgements
This work was supported by the National Natural Science Foundation of China (21675119, 21974101) and National Major Science and Technology Projects (2018ZX10301405).
Notes and references
- S. Mazumder, R. Dey, M. K. Mitra, S. Mukherjee and G. C. Das, J. Nanomater., 2009, 815734, DOI:10.1155/2009/815734.
- J. Zhou, Y. Yang and C. Y. Zhang, Chem. Rev., 2015, 115, 11669–11717 CrossRef CAS PubMed.
- I. L. Medintz, H. T. Uyeda, E. R. Goldman and H. Mattoussi, Nat. Mater., 2005, 4, 435–446 CrossRef CAS PubMed.
- R. Gill, M. Zayats and I. Willner, Angew. Chem., Int. Ed., 2008, 47, 7602–7625 CrossRef CAS PubMed.
- A. Aboulaich, D. Billaud, M. Abyan, L. Balan, J. J. Gaumet, G. Medjadhi, J. Ghanbaja and R. Schneider, ACS Appl. Mater. Interfaces, 2012, 4, 2561–2569 CrossRef CAS PubMed.
- L. H. Jing, S. V. Kershaw, Y. L. Li, X. D. Huang, Y. Y. Li, A. L. Rogach and M. Y. Gao, Chem. Rev., 2016, 116, 10623–10730 CrossRef CAS PubMed.
- M. Bruchez Jr, M. Moronne, P. Gin, S. Weiss and A. P. Alivisatos, Science, 1998, 281, 2013–2016 CrossRef CAS PubMed.
- W. C. W. Chan and S. M. Nie, Science, 1998, 281, 2016–2018 CrossRef CAS PubMed.
- W. B. Cai, D. W. Shin, K. Chen, O. Gheysens, Q. Z. Cao, S. X. Wang, S. S. Gambhir and X. Y. Chen, Nano Lett., 2006, 6, 669–676 CrossRef CAS PubMed.
- G. Ruan, A. Agrawal, A. I. Marcus and S. Nie, J. Am. Chem. Soc., 2007, 129, 14759–14766 CrossRef CAS PubMed.
- O. K. Nag, M. H. Stewart, J. R. Deschamps, K. Susumu, E. Oh, V. Tsytsarev, Q. Tang, A. L. Efros, R. Vaxenburg, B. J. Black, Y. C. Chen, T. J. O’Shaughnessy, S. H. North, L. D. Field, P. E. Dawson, J. J. Pancrazio, I. L. Medintz, Y. Chen, R. S. Erzurumlu, A. L. Huston and J. B. Delehanty, ACS Nano, 2017, 11, 5598–5613 CrossRef CAS PubMed.
- C. Y. Zhang, H. C. Yeh, M. T. Kuroki and T. H. Wang, Nat. Mater., 2005, 4, 826–831 CrossRef CAS PubMed.
- Y. Wang, P. D. Howes, E. Kim, C. D. Spicer, M. R. Thomas, Y. Y. Lin, S. W. Crowder, I. J. Pence and M. M. Stevens, ACS Appl. Mater. Interfaces, 2018, 10, 28290–28300 CrossRef CAS PubMed.
- R. Hu, X. B. Zhang, R. M. Kong, X. H. Zhao, J. Jiang and W. Tan, J. Mater. Chem., 2011, 21, 16323–16334 RSC.
- D. Mandal, S. Khatun, A. N. Gupta and A. Chandra, Nanotechnology, 2019, 30, 255501–255508 CrossRef CAS PubMed.
- B. A. Kairdolf, X. M. Qian and S. M. Nie, Anal. Chem., 2016, 89, 1015–1031 CrossRef PubMed.
- J. J. Wang, Y. Lin, Y. Z. Jiang, Z. H. Zheng, H. Y. Xie, C. Lv, Z. L. Chen, L. H. Xiong, Z. L. Zhang, H. Z. Wang and D. W. Pang, Anal. Chem., 2019, 91, 7280–7287 CrossRef CAS PubMed.
- I. L. Medintz, M. H. Stewart, S. A. Trammell, K. Susumu, J. B. Delehanty, B. C. Mei, J. S. Melinger, J. B. Blanco-Canosa, P. E. Dawson and H. Mattoussi, Nat. Mater., 2010, 9, 676–684 CrossRef CAS PubMed.
- W. Wei, X. W. He and N. Ma, Angew. Chem., Int. Ed., 2014, 53, 5573–5577 CrossRef CAS PubMed.
- C. P. Ding, C. L. Zhang, X. Y. Yin, X. Y. Cao, M. F. Cai and Y. Z. Xian, Anal. Chem., 2018, 90, 6702–6709 CrossRef CAS PubMed.
- Z. Li, G. L. Wang, Y. Shen, N. N. Guo and N. Ma, Adv. Funct. Mater., 2018, 28, 1707152 CrossRef.
- Z. T. Deng, S. Pal, A. Samanta, H. Yan and Y. Liu, Chem. Sci., 2013, 4, 2234–2240 RSC.
- X. C. Luo, Z. Li, G. L. Wang, X. W. He, X. Q. Shen, Q. H. Sun, L. Wang, R. Y. Yue and N. Ma, ACS Appl. Mater. Interfaces, 2017, 9, 33624–33631 CrossRef CAS PubMed.
- L. B. Zhang, S. R. Jean, S. Ahmed, P. M. Aldridge, X. Y. Li, F. J. Fan, E. H. Sargent and S. O. Kelley, Nat. Commun., 2017, 8, 381 CrossRef PubMed.
- X. H. Lou, J. R. Qian, Y. Xiao, L. Viel, A. E. Gerdon, E. T. Lagally, P. Atzberger, T. M. Tarasow, A. J. Heeger and H. T. Soh, Proc. Natl. Acad. Sci. U. S. A., 2009, 106, 2989–2994 CrossRef CAS PubMed.
- Y. M. Miao, X. J. Sun, J. Z. Lv and G. Q. Yan, Anal. Chem., 2019, 91, 5036–5042 CrossRef CAS PubMed.
- C. Liu, G. B. Mao, C. Su, X. H. Ji, Z. X. Chen and Z. K. He, Anal. Methods, 2015, 7, 7748–7752 RSC.
- Z. Li, X. W. He, X. C. Luo, G. L. Wang and N. Ma, Anal. Chem., 2016, 88, 9355–9358 CrossRef CAS PubMed.
- T. Qiu, D. Zhao, G. H. Zhou, Y. Liang, Z. K. He, Z. H. Liu, X. N. Peng and L. Zhou, Analyst, 2010, 135, 2394–2399 RSC.
- Z. H. Lin, D. Pan, T. Y. Hu, Z. P. Liu and X. G. Su, Microchim. Acta, 2015, 182, 1933–1939 CrossRef CAS.
- D. Wu, G. F. Song, Z. Li, T. Zhang, W. Wei, M. Z. Chen, X. W. He and N. Ma, Chem. Sci., 2015, 6, 3839–3844 RSC.
- W. R. Algar, D. E. Prasuhn, M. H. Stewart, T. L. Jennings, J. B. Blanco-Canosa, P. E. Dawson and I. L. Medintz, Bioconjugate Chem., 2011, 22, 825–858 CrossRef CAS PubMed.
- N. Ma, E. H. Sargent and S. O. Kelley, Nat. Nanotechnol., 2009, 4, 121–125 CrossRef CAS.
- G. Tikhomirov, S. Hoogland, P. E. Lee, A. Fischer, E. H. Sargent and S. O. Kelley, Nat. Nanotechnol., 2011, 6, 485–490 CrossRef CAS PubMed.
- G. L. Wang, Z. Li and N. Ma, ACS Chem. Biol., 2018, 13, 1705–1713 CrossRef CAS PubMed.
- C. L. Zhang, J. Xu, S. M. Zhang, X. H. Ji and Z. K. He, Chem. – Eur. J., 2012, 18, 8296–8300 CrossRef CAS PubMed.
- F. Aldeek, X. Ji and H. Mattoussi, J. Phys. Chem. C, 2013, 117, 15429–15437 CrossRef CAS.
- G. B. Mao, Q. Cai, F. B. Wang, C. L. Luo, X. H. Ji and Z. K. He, Anal. Chem., 2017, 89, 11628–11635 CrossRef CAS PubMed.
- R. Y. Yue, Z. Li, G. L. Wang, J. Y. Li and N. Ma, ACS Sens., 2019, 4, 250–256 CrossRef CAS PubMed.
- A. Banerjee, T. Pons, N. Lequeux and B. Dubertret, Interface Focus, 2016, 6, 20160064 CrossRef PubMed.
- C. L. Zhang, C. P. Ding, D. S. Xiang, L. Li, X. H. Ji, Z. K. He and Y. Z. Xian, Chin. J. Chem., 2016, 34, 317–325 CrossRef CAS.
- K. E. Sapsford, W. R. Algar, L. Berti, K. B. Gemmill, B. J. Casey, E. Oh, M. H. Stewart and I. L. Medintz, Chem. Rev., 2013, 113, 1904–2074 CrossRef CAS PubMed.
- J. C. Park, S. Y. Choi, M. Y. Yang, L. Nan, H. Na, H. N. Lee, H. J. Chung, C. A. Hong and Y. S. Nam, ACS Appl. Mater. Interfaces, 2019, 11, 33525–33534 CrossRef CAS PubMed.
- P. Reiss, M. Carrière, C. Lincheneau, L. Vaure and S. Tamang, Chem. Rev., 2016, 116, 10731–10819 CrossRef CAS PubMed.
- J. Lu, M. Tang and T. Zhang, J. Appl. Toxicol., 2019, 39, 72–86 CrossRef CAS.
- C. L. Zhang, X. H. Ji, Y. Zhang, G. H. Zhou, X. L. Ke, H. Z. Wang, P. Tinnefeld and Z. K. He, Anal. Chem., 2013, 85, 5843–5849 CrossRef CAS PubMed.
- C. L. Zhang, J. Yan, C. Liu, X. H. Ji and Z. K. He, ACS Appl. Mater. Interfaces, 2014, 6, 3189–3194 CrossRef CAS PubMed.
- G. B. Mao, C. Liu, M. Y. Du, Y. W. Zhang, X. H. Ji and Z. K. He, Talanta, 2018, 185, 123–132 CrossRef CAS PubMed.
- A. Goryacheva, A. S. Novikova, D. D. Drozd, P. S. Pidenko, T. S. Ponomaryeva, A. A. Bakal, P. K. Mishra, N. V. Beloglazova and I. Y. Goryacheva, Trends Anal. Chem., 2019, 111, 197–205 CrossRef.
- M. Kimoto, R. Yamashige, K. Matsunaga, S. Yokoyama and I. Hirao, Nat. Biotechnol., 2013, 31, 453–457 CrossRef CAS PubMed.
- H. T. Ma, J. P. Liu, M. M. Ali, M. A. I. Mahmood, L. Labanieh, M. R. Lu, S. M. Iqbal, Q. Zhang, W. A. Zhao and Y. Wan, Chem. Soc. Rev., 2015, 44, 1240–1256 RSC.
- M. H. Lee, J. S. Kim and J. L. Sessler, Chem. Soc. Rev., 2015, 44, 4185–4191 RSC.
- G. B. Mao, M. Y. Du, X. X. Wang, X. H. Ji and Z. K. He, Analyst, 2018, 143, 5295–5301 RSC.
- F. Jou, C. H. Lu, Y. C. Ou, S. S. Wang, S. L. Hsu, I. Willner and J. A. Ho, Chem. Sci., 2015, 6, 659–665 RSC.
- E. Kim, P. D. Howes, S. W. Crowder and M. M. Stevens, ACS Sens., 2016, 2, 111–118 CrossRef PubMed.
- J. B. Li, L. Huang, X. Xiao, Y. J. Chen, X. X. Wang, Z. Q. Zhou, C. Y. Zhang and Y. Zhang, J. Am. Chem. Soc., 2016, 138, 15943–15949 CrossRef CAS PubMed.
- Y. X. Chen, K. J. Huang and K. X. Niu, Biosens. Bioelectron., 2018, 99, 612–624 CrossRef CAS PubMed.
- C. A. Mirkin, R. L. Letsinger, R. C. A. Mucic and J. J. Storhoff, Nature, 1996, 382, 607–609 CrossRef CAS PubMed.
- P. Alivisatos, K. P. Johnsson, X. G. Peng, T. E. Wilson, C. J. Loweth, M. P. Bruchez and P. G. Schultz, Nature, 1996, 382, 609–611 CrossRef PubMed.
- J. Y. Huang, H. T. Lin, T. H. Chen, C. A. Chen, H. T. Chang and O. F. Chen, ACS Sens., 2018, 3, 174–182 CrossRef CAS PubMed.
- X. L. Zhao, L. G. Xu, M. Z. Sun, W. Ma, X. L. Wu, H. Kuang, L. B. Wang and C. L. Xu, Small, 2016, 12, 4662–4668 CrossRef CAS PubMed.
- T. Lee, Y. Lee, S. Y. Park, K. Hong, Y. Kim, C. Park, Y. H. Chung, M. H. Lee and J. Min, Colloids Surf., B, 2019, 175, 343–350 CrossRef CAS PubMed.
- X. W. He, T. Zeng, Z. Li, G. L. Wang and N. Ma, Angew. Chem., Int. Ed., 2016, 55, 3073–3076 CrossRef CAS PubMed.
- P. Das, A. Sedighi and U. J. Krull, Anal. Chim. Acta, 2018, 1041, 1–24 CrossRef CAS PubMed.
- Y. C. Liu, G. B. Mao, W. Wang, S. B. Tian, X. H. Ji, M. Liu and Z. K. He, Chem. Commun., 2019, 55, 2660–2663 RSC.
- G. B. Mao, W. Q. Peng, S. B. Tian, J. Zheng, X. H. Ji and Z. K. He, Sens. Actuators, B, 2019, 283, 755–760 CrossRef CAS.
- Y. X. Ma, G. B. Mao, Y. C. Zhong, G. Q. Wu, W. J. Wu, Y. H. Zhan, Z. K. He and W. R. Huang, Talanta, 2019, 194, 199–204 CrossRef CAS PubMed.
- A. Adams, P. I. Okagbare, J. Feng, M. L. Hupert, D. Patterson, J. Göttert, R. L. McCarley, D. Nikitopoulos, M. C. Murphy and S. A. Soper, J. Am. Chem. Soc., 2008, 130, 8633–8641 CrossRef CAS PubMed.
- J. A. Phillips, Y. Xu, Z. Xia, Z. H. Fan and W. H. Tan, Anal. Chem., 2009, 81, 1033–1039 CrossRef CAS PubMed.
- P. G. Schiro, M. Zhao, J. S. Kuo, K. M. Koehler, D. E. Sabath and D. T. Chiu, Angew. Chem., Int. Ed., 2012, 51, 4618–4622 CrossRef CAS PubMed.
- Y. L. Chen, D. Tyagi, M. Lyu, A. J. Carrier, C. Nganou, B. Youden, W. Wang, S. F. Cui, M. Servos, K. Oakes, S. N. He and X. Zhang, Anal. Chem., 2019, 91, 4017–4022 CrossRef CAS PubMed.
- T. Gao, L. L. Li, T. S. Chen, L. Shi, Y. Yang and G. X. Li, Anal. Chem., 2019, 91, 1126–1132 CrossRef CAS PubMed.
- X. Y. Zhou, Y. J. Li, H. P. Wu, W. Huang, H. X. Ju and S. J. Ding, Biosens. Bioelectron., 2019, 130, 88–94 CrossRef CAS PubMed.
- Y. Zheng, X. Y. Wang, S. Q. He, Z. H. Gao, Y. Di, K. L. Lu, K. Li and J. D. Wang, Biosens. Bioelectron., 2019, 126, 261–268 CrossRef CAS PubMed.
- K. D. Wegner and N. Hildebrandt, Chem. Soc. Rev., 2015, 44, 4792–4834 RSC.
- Y. X. Ma, G. B. Mao, W. R. Huang, G. Q. Wu, W. Yin, X. H. Ji, Z. S. Deng, Z. M. Cai, X. E. Zhang, Z. K. He and Z. Q. Cui, J. Am. Chem. Soc., 2019, 141, 13454–13458 CrossRef CAS PubMed.
- Y. Zhang, L. Zhang, Z. Z. Wang, F. M. Wang, L. H. Kang, F. F. Cao, K. Dong, J. S. Ren and X. G. Qu, Biomaterials, 2019, 223, 119462 CrossRef CAS PubMed.
- S. Li, X. Q. Shen, Q. H. Xu and Y. Cao, Nanoscale, 2019, 11, 19551–19560 RSC.
- C. Zhang, D. W. Zheng, C. X. Li, M. Z. Zou, W. Y. Yu, M. D. Liu, S. Y. Peng, Z. L. Zhong and X. Z. Zhang, Biomaterials, 2019, 223, 119472 CrossRef CAS PubMed.
- S. N. Li, L. Y. Zhang, X. Liang, T. T. Wang, X. J. Chen, C. M. Liu, L. Li and C. G. Wang, Chem. Eng. J., 2019, 378, 122175 CrossRef CAS.
- A. Hasanzadeh, F. Radmanesh, J. Kiani, M. Bayandori, Y. Fatahi, A. R. Aref and M. Karimi, Nanotechnology, 2019, 30, 135101 CrossRef CAS PubMed.
- L. Kelland, Nat. Rev. Cancer, 2007, 7, 573–584 CrossRef CAS PubMed.
- G. L. Wang, Z. Li, X. C. Luo, R. Y. Yue and N. Ma, Nanoscale, 2018, 10, 16508–16520 RSC.
- L. B. Zhang, S. R. Jean, X. Y. Li, T. Sack, Z. J. Wang, S. Ahmed, G. J. Das, A. Zaragoza, E. H. Sargent and S. O. Kelley, Nano Lett., 2018, 18, 6222–6228 CrossRef CAS PubMed.
Footnote |
† Yeling Yang and Guobin Mao contributed equally to this work. |
|
This journal is © The Royal Society of Chemistry 2020 |