In situ derivatization of Au nanoclusters via aurophilic interactions of a triphenylphosphine gold(I) salt with neurotransmitters and their rapid MALDI-TOF-MS detection in mice brain tissue extracts†
Received
22nd August 2019
, Accepted 30th October 2019
First published on 4th November 2019
Abstract
Matrix-assisted laser desorption/ionization (MALDI) mass spectrometry (MS) has attracted much attention for the detection of small molecules such as neurotransmitters due to its softness, high sensitivity, extensive compatibility and diverse mass analyzers. However, it has been really a difficult challenge to develop a highly specific organic compound as a matrix for the rapid, sensitive and selective detection of neurotransmitters. Herein, we report tris(triphenylphosphine)gold oxonium tetrafluoroborate ([Ph3PAu]3O+BF4−) for the first time as an efficient matrix for the rapid and simultaneous MALDI-MS detection of neurotransmitters. [Ph3PAu]3O+BF4− facilitates the in situ derivatization of gold nanoclusters (Au NCLs) during the interaction with neurotransmitters, which increases their ionization energy by absorbing more ultra-violet (UV) radiation during MALDI-TOF-MS detection. The results show that this [Ph3PAu]3O+BF4− matrix can exhibit a 10-fold faster response time compared to previously reported pyrylium matrices. In addition, [Ph3PAu]3O+BF4− can also provide the simultaneous derivatization of various neurotransmitters, including dopamine (DA), noradrenaline (NAd), serotonin (5-HT), γ-aminobutyric acid (GABA), histamine (H) and tyramine (TY), in mice brain tissue extracts, which can be detected in the MALDI-TOF-MS spectra.
Introduction
Neurotransmitters are important chemicals in the human brain, which act as messengers for the synaptic signal transmission process. Any imbalance in the activities of neurotransmitters can cause serious disorders such as Parkinson's disease (PD), Schizophrenia, and Alzheimer's disease (AD). Therefore, it is necessary to monitor the concentrations of various neurotransmitters for studying and diagnosing such illnesses. Recently, many researchers have explored various methods using different materials for developing biosensors for both in vitro and in vivo neurotransmitter detection. However, it has been challenging to monitor such neurotransmitters in medical treatments and clinical applications owing to the poor sensitivity, selectivity and response time of the developed biosensors.1
A biosensor is an analytical tool that can continuously provide real time monitoring and highlight the dynamic behaviours of the various neurotransmitters. It can also be a powerful tool for monitoring and treating patients with neurological brain disorders such as PD, AD, epilepsy and addiction. For instance, the primary symptom of PD is the gradual deterioration of the substantia nigra, which controls motor functioning skills such as balance and movement.2 It is located deep in the brainstem3,4 where dopamine (DA) is produced, and DA is responsible for neural communication between the striatum and the substantia nigra.5 The major PD symptoms appear only after dopaminergic neuronal death exceeds a critical threshold of 70–80%.6 A decreased level of DA is directly associated with an uncontrolled motor function, which leads to the inability to neutralize the imbalance in neurotransmitters.7 In particular, the equilibrium between DA and acetylcholine maintains the motor function in the striatum, and disruption in this equilibrium can bring about the progression of PD.8–10 In addition, it is known that a dysfunction in norepinephrine (NAd) is a contributing factor for PD, while serotonin (5-HT) and γ-aminobutyric acid (GABA) may also affect the conditions that cause secondary symptoms of PD.11,12 Moreover, it has been reported that the initial neurotransmitter and immune mediator of histamine (H) levels are significantly elevated in the brain of a patient with PD.13,14 The above findings illustrate that there is a complex interplay between various neurotransmitters that are closely related to the progression of neural diseases. Therefore, the simultaneous detection of multiple neurotransmitters in vivo is urgently needed for the better understanding of brain-related disorders and for the development of treatments and therapies for such diseases.
Matrix-assisted laser desorption ionization (MALDI) is an ionization technique that can be used for the analysis of neurotransmitters when coupled with mass spectrometry (MS).15–18 It has several advantages over other methods due to its benignity, high sensitivity, compatibility and diverse mass analyzers. The sample for a MALDI-MS analysis is prepared by dropping or spraying a solution containing an organic compound, called matrix, over the surface of neurotransmitters. The neurotransmitters entrapped within the matrix co-crystallize during the crystallization of the matrix on drying. Singly protonated ions are generated from the neurotransmitters during desorption and ionization by means of a laser beam. The protonated ions are then accelerated at a fixed potential and separate from each other on the basis of their mass-to-charge ratio (m/z). The charged neurotransmitters are then detected and measured using different types of mass analyzers such as quadrupole mass analyzers, ion trap analyzers, and time of flight (TOF) analyzers. In the case of neurotransmitters, TOF mass analyzers are mainly used. During MALDI-TOF analysis, the m/z ratio is measured by determining the time required for the ions to travel through the length of the flight tube. An ion mirror at the rear end of the flight tube is incorporated in a few TOF mass analyzers, which serves to reflect back ions through the flight tube to a detector. Thus, the ion mirror not only increases the length of the flight tube, but also corrects small differences in energy among ions.19
Numerous organic compounds have already been used as matrices for MALDI-TOF-MS, among which α-cyano-4-hydroxycinnamic acid (CHCA), 2,5-dihydroxy benzoic acid (DHB), and 3,5-dimethoxy-4-hydroxycinnamic acid and sinapinic acid (SA) have been found to be the most successful for detecting neurotransmitters. The matrix solution consists of water, a mixture of organic solvents such as ethanol/methanol or acetonitrile, and a strong acid like trifluoro acetic acid, which dissolves the matrix. The solvents penetrate the cell walls and extract the neurotransmitters. When the solvents evaporate, ‘co-crystallization’ of neurotransmitters and other cellular compounds entrapped within the matrix solution takes place.20 However, most of the conventional matrices are not specific enough for the sensitive and selective detection of neurotransmitters. To overcome this, recently, 2,4-diphenylpyrylium tetrafluoroborate has been reported as an efficient matrix for the direct, targeted and quantitative MALDI imaging of neurotransmitters.21–27 By using this pyrylium matrix, the sensitivity and selectivity for neurotransmitters have been increased several folds by using MALDI-MS. However, this matrix is limited by its slow reaction rate during the derivatization of neurotransmitters.
To improve the reaction rate, in this study, we have used tris(triphenylphosphine)gold oxonium tetrafluoroborate ([Ph3PAu]3O+BF4−) as a specific matrix for the rapid and simultaneous detection of neurotransmitters by using MALDI-TOF-MS. [Ph3PAu]3O+BF4− can increase the reaction rate several folds due to the rapid formation of metallic gold nanoclusters (Au NCLs) in the presence of neurotransmitters. The Au NCLs can absorb more UV light from MALDI-TOF-MS and increase the ionization of neurotransmitters to achieve simultaneous detection. [Ph3PAu]3O+BF4− can also allow to visually differentiate catecholamine neurotransmitters from non-catecholamine neurotransmitters by the formation of wine-red coloured Au nanoparticles (NPs).
Experimental
Materials and methods
All the chemicals purchased were of analytical reagent grade and used as received without any further purification, unless otherwise specified. Organophosphine gold(I) salts such as tris(triphenylphosphine)gold oxonium tetrafluoroborate ([Ph3PAu]3O+BF4−), chloro triphenylphosphine gold(I) ([Ph3PAu]+Cl−) and trimethyloxonium tetrafluoroborate ([CH3]3O+BF4−), and neurotransmitters such as dopamine hydrochloride (DA), noradrenaline hydrochloride (NAd), serotonin hydrochloride (5-HT), γ-aminobutyric acid (GABA) and histamine (H), were purchased from Sigma Aldrich suppliers in Dalian, China. 2,4,6-Trimethylpyrylium tetrafluoroborate (TMP) was obtained from Tokyo Chemical Industry Co., Ltd, while 2,6-diphenylpyrylium tetrafluoroborate (DPP) and 2,4,5-triphenylpyrylium tetrafluoroborate (TPP) matrices were gifted by Professor Guiling Ning from the State Key Laboratory of Fine Chemicals at Dalian University of Technology. Mice brain samples were obtained from Dalian Medical University in Dalian, China. The animal experiments were performed in compliance with the relevant Chinese government laws and institutional guidelines, and the ethical committee has approved our experiments.
Various neurotransmitters dissolved in organophosphine gold(I) salt dissolved in acetonitrile solvent were thoroughly mixed using a vortex shaker purchased from local chemical industries in Dalian, China. The samples were washed by using a centrifugation unit purchased from local chemical industries in Dalian, China before MALDI-TOF-MS analysis. The centrifugation was performed at 12
000 rpm for 5 min. The mass spectrometric measurements were taken at the National Chromatographic Research and Analysis Center at Dalian Institute of Chemical Physics (DICP) by using a MALDI-TOF/TOF 5800 instrument under 349 nm UV light irradiation, while UV-vis measurements were performed at the division of Bio mass conversion in the CAS Key Laboratory of Separation Science for Analytical Chemistry of DICP. Scanning electron microscopy (SEM), transmission electron microscopy (TEM) and energy dispersive X-ray spectroscopy (EDS) measurements for elemental distribution were taken in Dalian National Laboratory for Clean Energy at DICP.
Sample preparation for MALDI-TOF-MS
First, 1 mM [Ph3PAu]3O+BF4− and 1 mM neurotransmitter (DA, NAd, 5-HT, GABA & H) stock solutions were prepared using 100% acetonitrile as the solvent. Second, different concentrations of neurotransmitter solutions were prepared by adding selected amounts of neurotransmitters from the respective stock into 100 μM of [Ph3PAu]3O+BF4− solution. The final concentrations of [Ph3PAu]3O+BF4−, solvent and standard additional matrix have been optimized and the results are shown in S1–S3 (ESI†). Then, the mixture was agitated for 20 min using a vortex shaker. After 20 min, the solutions were transferred out of the vortex shaker and allowed to react for additional 3 h. A wine-red colour of the colloidal suspension was observed to confirm the formation Au NPs upon adding neurotransmitters, especially DA, NAd and 5-HT into the [Ph3PAu]3O+BF4− solution, while Au NCLs were obtained in the case of GABA and H. The obtained Au samples were washed by centrifugation at 12
000 rpm for 5 min. After centrifugation, the supernatants were collected and dropped on to a MALDI plate for the analysis.21–25
Preparation of mice brain tissue extracts
Nine-week-old male Kunming mice (20–22 g) were housed in individually ventilated cages and kept in a regulated environment (22 °C temperature & 50% humidity) with free access to standard food and water. All the experiments were performed according to the guidelines of the Chinese Society of Laboratory Animal Sciences to ensure animal welfare. The brain extracts were prepared as reported previously.28,29 The mice were sacrificed by decapitation after anesthetization with chloral hydrate (100 mg kg−1). The head was immediately removed and heated up to 80 °C for 16 s using microwave irradiation. The brains were rapidly removed from the cranium and three areas (striatum, hippocampus and prefrontal cortex) were dissected. The tissues of each area were collected from five different mice brains and then pooled together, homogenized by an automated Mini bead beater homogenizer (from Biospec products) and divided in three samples that were processed separately. The striatum, hippocampus and prefrontal cortex tissues (each 90 mg) were individually homogenized by using 300 μL of ice-cold acetonitrile containing 0.3% formic acid. The homogenization was performed for five times for 30 s (the interval was kept as 10 s each time). The homogenates were centrifuged at 12
000 rpm for 30 min at 4 °C. The supernatants were collected and filtered through 0.20 μm filter membranes. The filtered supernatants were directly used for the MALDI-TOF-MS analysis after derivatization with 100 μM [Ph3PAu]3O+BF4−.
Detection mechanism
During the interaction, first, the Au–O–Au bonds of [Ph3PAu]3O+BF4− cleave in the presence of neurotransmitters dissolved in acetonitrile. After cleavage, different ligands containing Au+ ions such as [Ph3PAu]+, [(Ph3P)2Au]+ and [(Ph3PAu)3]+ are released. Then, the nitrogen atom from the primary amine groups of various neurotransmitters acts as an aggregation or auration center to generate ammonium derivatives with Au–N–Au bonds to form Au NCLs. However, only [Ph3PAu]+ has been observed to be involved in auration, while [(Ph3P)2Au]+ and [(Ph3PAu)3]+ are not involved, as their Au+ ions are already stabilized with Ph3P ligands. Numerous research findings have already discussed the mechanism of organophosphine-supported AuNCLs around the nitrogen atom of primary amines. It has also been reported that one central nucleophilic atom can carry as many as six Au+ clustering units. The aurophilic interaction can be realized in two conditions, namely, the Au atom should be monoatomic and the distance between two Au atoms should be as close as possible, with linear orientation. Aurophilic interactions are much weaker than ionic and covalent bonding, but they can have the strength equal to that of hydrogen bonding.30–40 The detailed mechanism for the formation of Au NCLs such as monomers, dimers and trimers is shown in Scheme 1. Au NCLs can be further converted into Au NPs in the presence of catechol groups in neurotransmitters. Catechol groups can easily release one or two electrons during the oxidation, and the released electrons can be utilized to reduce Au+ ions of Au NCLs into neutral Au NPs, as shown in Scheme 2.41–43 However, the formation of Au NPs is not possible for non-catecholamine neurotransmitters such as GABA and H, and they can only produce Au NCLs.
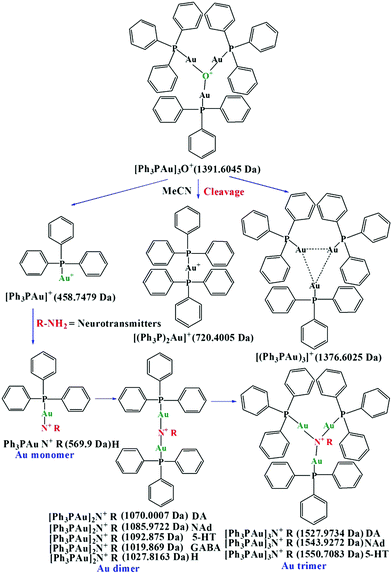 |
| Scheme 1 Mechanism for the cleavage of [Ph3PAu]3O+BF4−, and for the formation of Au monomer, dimer and trimer. | |
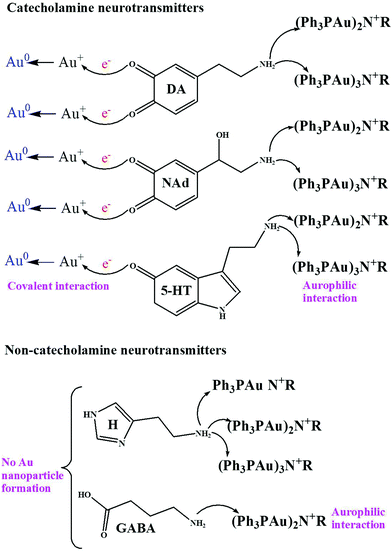 |
| Scheme 2 Mechanism for the recognition of various neurotransmitters by the [Ph3PAu]+ adduct. | |
Results and discussion
Characterization of Au NCLs and Au NPs
The formation of Au NCLs upon adding various neurotransmitters into [Ph3PAu]3O+BF4− has been confirmed by the MALDI-TOF-MS results, as shown in S4a–f (ESI†). The samples were prepared by adding 100 μM of various neurotransmitters separately into a series of vials containing 100 μM [Ph3PAu]3O+BF4−. Based on the obtained results, [Ph3PAu]3O+BF4− produced a mixture of Au dimers and trimers with DA, NAd and 5-HT. The amount of dimer was higher than that of trimer, and no monomer was found. In the case of H, monomer, dimer and trimer were observed, and the amount of monomer was higher than that of dimer and trimer. Unfortunately, only dimer was obtained for GABA.44,45 This shows that the formation of a monomer, dimer and trimer is related to the presence of not only primary amine groups, but also catechol groups of neurotransmitters. The neurotransmitters with catechol groups, called catecholamine neurotransmitters, such as DA, NAd and 5-HT can achieve dual molecular recognition with [Ph3PAu]+ by interacting with both catechol and primary amine groups. Due to the dual molecular interaction, catecholamines can form larger Au NCLs than non-catecholamines such as H and GABA.41,42
In addition, the catechol groups of neurotransmitters also contribute as reducing agents for the conversion of Au NCLs into wine-red coloured Au NPs. There is some strong evidence available in the literature that supports the fact that the catechol groups of neurotransmitters can easily auto-oxidize in open air to form intermediate semiquinones and/or quinones by releasing one or two electrons. The released electrons can reduce Au+ ions in Au NCLs to form neutral Au NPs, as shown in Scheme 2.41–43 To confirm this hypothesis, Au NP formation has been observed only for catecholamine neurotransmitters such as DA, NAd and 5-HT, while it was not found for non-catecholamine neurotransmitters such as H and GABA. The formation of Au NPs was confirmed by the UV-vis absorbance bands at 527 nm and 300 nm, as shown in Fig. 1a. The absorbance band at 527 nm indicates the occurrence of metallic Au NPs, while the absorbance band at 300 nm shows the phenylphosphine capping layer from the [Ph3PAu]3O+BF4− matrix over the Au surface. Noticeably, the absorbance band at 527 nm for Au NPs was not detected, and only the phenylphosphine matrix peak was retained for non-catecholamines (GABA & H) to confirm that the non-catecholamines without electron rich catechol groups cannot produce Au NPs. The obtained spherical Au NPs are displayed in the TEM images in Fig. 1b and c.42,46 The SEM and EDS results of obtained Au NPs are shown in S5 (ESI†).
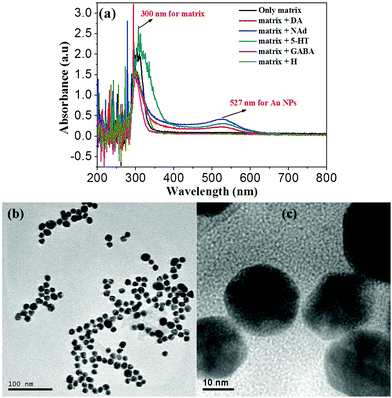 |
| Fig. 1 (a) UV-vis absorption spectra of the [Ph3PAu]3O+BF4− matrix in the presence of various neurotransmitters; (b and c) TEM images of as formed Au NPs during the interaction of [Ph3PAu]+ with neurotransmitter DA. | |
A number of other aurating agents is also available for the introduction of [Ph3PAu]+ units on a given substrate, including phosphine gold fluoroborates, halides, triflates, perchlorates and acetylacetates.43 For the present purpose, we have chosen [Ph3PAu]3O+BF4−, due to the high affinity of the oxonium ion being a useful driving force for the formation of Au NCLs with ammonium derivatives. The oxonium ion in [Ph3PAu]3O+BF4− also acts as a template for the formation of ammonium derivatives. Both oxonium and Au+ ions in [Ph3PAu]3O+BF4− play an equal role in the formation of ammonium derivatives with the primary amine groups of neurotransmitters. The Au+ ion increases the bond strength of ammonium derivatives by providing a strong Au–N–Au linkage, while the oxonium ion provides a template for such a derivatization. The Ph3P ligand in [Ph3PAu]3O+BF4− suppresses the aggregation tendency of Au+ ions, and provides a stable structure of Au clusters and nanoparticles.40,44,45 To investigate this hypothesis, chloro(triphenylphosphine)gold(I) ([Ph3PAu]+Cl−) without the oxonium ion (but having the [Ph3PAu]+ adduct) and trimethyloxonium tetrafluoroborate ([CH3]3O+BF4−) without the Au+ ion (but having the oxonium ion) were used in place of [Ph3PAu]3O+BF4−, as shown in S6a–c (ESI†).38,44,47 Unfortunately, no products were found for the neurotransmitter DA with [Ph3PAu]+Cl− and [CH3]3O+BF4−. This is due to the absence of the oxonium template in [Ph3PAu]+Cl− and the absence of Au+ ions in [CH3]3O+BF4−. It was also found that [CH3]3O+BF4− without Au+ is not stable at room temperature, since no characteristic peaks for [CH3]3O+BF4− were observed in mass spectra.
Simultaneous detection of neurotransmitters
The simultaneous detection of various neurotransmitters such as DA, NAd, 5-HT, GABA and H has been investigated by obtaining MALDI-TOF-MS spectra, as shown in S7 (ESI†). The sample for simultaneous detection was prepared by mixing 50 μM solutions of various neurotransmitters into the same 100 μM solution of [Ph3PAu]3O+BF4−. Based on the obtained results, the products DA, NAd, 5-HT, GABA and H with [Ph3PAu]3O+BF4− have been observed in the same MALDI-TOF-MS spectrum.21–23 Even though [Ph3PAu]3O+BF4− forms Au monomers, dimers and trimers with neurotransmitters, Au dimers were commonly obtained for all the neurotransmitters. Hence, we have used only Au dimers for the simultaneous detection of various neurotransmitters. There were effective peak separations obtained between GABA–H, H–DA, DA–NAd and NAd–5-HT to confirm the selective and simultaneous detection of DA, NAd, 5-HT, GABA and H.21–25
Response time measurement
The response time for the formation of Au NPs was further investigated by measuring UV-vis absorbance with respect to time at 527 nm wavelength, as shown in Fig. 2a.46 According to the UV-vis results, the absorbance was constant from 0.5 min to 3 min and gradually increased 3 min after adding catecholamine neurotransmitters. This suggests that an Au0 layer was formed during the first 3 min after adding DA, NAd and 5-HT, which is known as the nucleation process. After 3 min, the nucleated Au0 layer acted as the seed layer for the propagation of Au NPs, and this step is known as the growth process. The sudden decrease in the first 0.5 min after adding neurotransmitters is probably due to the decrease in [Ph3PAu]+ concentration due to dilution. The obtained results also suggest that NAd with three hydroxyl groups has a faster response than DA with two hydroxyl groups, while 5-HT with solely one hydroxyl group has an extremely slow response. This shows that the response time is directly proportional to the number of hydroxyl groups in neurotransmitters, which act as electron donors. The catecholamine neurotransmitter with more hydroxyl groups releases more electrons during oxidation and increases the electron population for the reduction of Au+ ions into Au NPs.
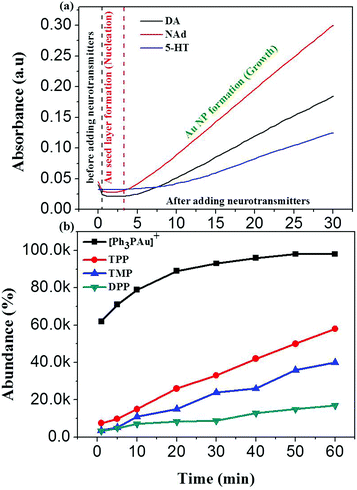 |
| Fig. 2 (a) UV-vis absorbance spectra of Au nanoparticles obtained at different times at 527 nm and (b) calibration plot showing the relationship between time and product abundance with different matrices. | |
In addition, the response time of [Ph3PAu]3O+BF4− was also compared with that of pyrylium salts, such as 2,4,5-triphenylpyrylium tetrafluoroborate (TPP), 2,4,6-trimethylpyrylium tetrafluoroborate (TMP) and 2,6-diphenylpyrylium tetrafluoroborate (DPP), as shown in Fig. 2b. The results show that the response time of [Ph3PAu]3O+BF4− is 10-fold faster than that for the pyrylium counterparts.
The limits of detection (LOD) for DA, NAd, 5-HT, GABA and H were measured in the range of several nanomoles by using the formula 3S/N, since they are sensitive enough for the detection of neurotransmitters at both cellular and tissue levels.46 The obtained MALDI-TOF-MS peak separation between GABA–H, H–DA, DA–NAd and NAd–5-HT was also satisfactory for the selective and simultaneous detection of DA, NAd, 5-HT, GABA and H. The response time was also calculated to be 30 s during the formation of Au NPs, which is fast enough for the rapid detection of neurotransmitters. The obtained results suggest that our developed [Ph3PAu]3O+BF4− can be successfully applied as a matrix for MALDI-TOF-MS detection of neurotransmitters using mice brain extracts.
Analysis of neurotransmitters in mice brain tissue extracts
To investigate the reliability of our [Ph3PAu]3O+BF4− matrix, we have also performed the MALDI-TOF-MS detection of neurotransmitters in the extracts from different regions of mice brain samples. The MALDI-TOF-MS results are shown in S8 (ESI†). The extracts from striatum, hippocampus and prefrontal cortex have been used for the analysis of neurotransmitters. The samples for MALDI-TOF-MS were prepared by adding different brain extracts into 100 μM [Ph3PAu]3O+BF4− solutions. According to Fig. 3, neurotransmitters such as DA, NAd, 5-HT, GABA and tyramine (TY) have been successfully detected in all the brain areas, while H could not be detected in any area. It was also confirmed that a higher abundance of DA, 5-HT and GABA can be found in the hippocampus, while NAd was found in higher concentrations in the prefrontal cortex and the striatum. Moreover, TY was found with high abundance in all samples (shown in S8, ESI†). This shows that the concentration of neurotransmitters in the brain is region-dependent.28,29
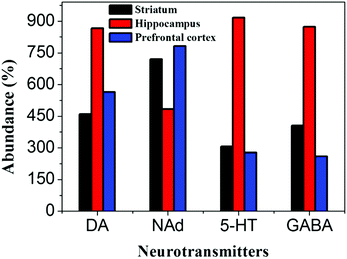 |
| Fig. 3 Calibration plot drawn between various neurotransmitters and their relative abundance in the different regions of mice brains. | |
Conclusion
An organometallic salt [Ph3PAu]3O+BF4− has been successfully applied as a matrix for the MALDI-TOF-MS detection of neurotransmitters in mice brain tissue extracts. [Ph3PAu]3O+BF4− has enabled the rapid and simultaneous detection of neurotransmitters such as DA, NAd, 5-HT, GABA, H and TY in tissue levels. [Ph3PAu]3O+BF4− also enabled the visual differentiation of catecholamine neurotransmitters such as DA, NAd and 5-HT from non-catecholamine neurotransmitters such as GABA and H through a colour change. In addition, the concentration of the neurotransmitters varied from different areas of the brain such as striatum, prefrontal cortex and hippocampus, which confirms that the concentration of neurotransmitters in the brain is completely region-dependent.
Conflicts of interest
The authors have no conflicts to declare.
Acknowledgements
The authors would like to acknowledge CAS-PIFI and MOST2016YFC1306603 for the financial support during this research work.
References
- B. Shi and E. Song, Chemosensors, 2018, 6, 1 CrossRef.
- J. Jankovic, J. Neurol. Neurosurg. Psychiatry, 2008, 79, 368 CrossRef CAS PubMed.
- J. M. Fearnley and A. J. Lees, Brain, 1991, 114, 2283 CrossRef PubMed.
- A. Gröger, R. Kolb, R. Schäfer and U. Klose, PLoS One, 2014, 9, e84081 CrossRef PubMed.
- H. Braak, K. D. Tredici, U. Rüb, R. A. I. de Vos, E. N. H. J. Steur and E. Braak, Neurobiol. Aging, 2003, 24, 197 CrossRef.
- E. Bezard, C. E. Gross and J. M. Brotchie, Trends Neurosci., 2003, 26, 215 CrossRef CAS.
- M. C. Rodriguez-Oroz, M. Jahanshahi, P. Krack, I. Litvan, R. Macias, E. Bezard and J. A. Obeso, Lancet Neurol., 2009, 8, 1128 CrossRef CAS.
- T. Aosaki, M. Miura, T. Suzuki, K. Nishimura and M. Masuda, Geriatr. Gerontol. Int., 2010, 10, S148 CrossRef.
- P. Calabresi, B. Picconi, L. Parnetti and M. Di Filippo, Lancet Neurol., 2006, 5, 974 CrossRef CAS.
- P. DeBoer, M. J. Heeringa and E. D. Abercrombie, Eur. J. Pharmacol., 1996, 317, 257 CrossRef CAS.
- H. Braak, E. Ghebremedhin, U. Rüb, H. Bratzke and K. D. Tredici, Cell Tissue Res., 2004, 318, 121 CrossRef.
- M. J. Millan, Prog. Neurobiol., 2003, 70, 83 CrossRef CAS.
- S. M. Rocha, J. Pires, M. Esteves, B. Graça and L. Bernardino, Front. Cell. Neurosci., 2014, 8, 120 Search PubMed.
- J. O. Rinne, O. V. Anichtchik, K. S. Eriksson, J. Kaslin, L. Tuomisto, H. Kalimo, M. Röyttä and P. Panula, J. Neurochem., 2002, 81, 954 CrossRef CAS.
-
F. Hillenkamp and J. Peter-Katalinić, MALDI MS: A practical guide to instrumentation, methods, and applications, Wiley-VCH, Weinheim, 2007, vol. xvi, p. 345 Search PubMed.
- M. Karas, D. Bachmann and F. Hillenkamp, Anal. Chem, 1985, 17, 2935 CrossRef.
- J. J. van Kampen, P. C. Burgers, R. de Groot, R. A. Gruters and T. M. Luider, Mass Spectrom. Rev., 2011, 30, 101 CrossRef CAS.
- J. Webster and D. Oxley, Methods Mol. Biol., 2012, 800, 227 CrossRef CAS.
- J. R. Yates, J. Mass Spectrom., 1998, 33, 1 CrossRef CAS.
- V. Horneffer, A. Forsmann, K. Strupat, F. Hillenkamp and U. Kubitscheck, Anal. Chem., 2001, 73, 1016 CrossRef CAS.
- M. Shariatgorji, A. Nilsson, R. J. A. Goodwin, P. K. llback, N. Schintu, X. Zhang, A. R. Crossman, E. Bezard, P. Svenningsson and P. E. Andren, Neuron, 2014, 84, 697 CrossRef CAS.
- M. Shariatgorji, A. Nilsson, P. Källback, O. Karlsson, X. Zhang, P. Svenningsson and P. E. Andren, J. Am. Soc. Mass Spectrom, 2015, 26, 934 CrossRef CAS.
- Q. Cao, Y. Wang, B. Chen, F. Ma, L. Hao, G. Li, C. Ouyang and L. Li, ACS Chem. Neurosci., 2019, 10, 1222 CrossRef CAS.
- M. Waliczek, M. Kijewska, M. Rudowska, B. Setner, P. Stefanowiczand and Z. Szewczuk, Sci. Rep., 2016, 6, 37720 CrossRef CAS.
- M. Bayer and S. Konig, Electrophoresis, 2016, 37, 2953 CrossRef CAS.
- J. Ye, X. Wang, Y. Gao, L. Yang, Y. Lin and G. Ning, Chin. J. Org. Chem., 2015, 35, 373 CrossRef CAS.
- J. Li, J. Liu, Z. Liu, Y. Tan, X. Liu and F. Wang, Chem. Commun., 2017, 53, 12688 RSC.
- S. Falasca, F. Petruzziello, R. Kretz, G. Rainer and X. Zhang, J. Chromatogr. A, 2012, 1241, 46 CrossRef CAS.
- S. Falasca, V. Ranc, F. Petruzziello, A. Khani, R. Kretz, X. Zhang and G. Rainer, J. Chem. Neuroanat., 2014, 59, 29 CrossRef.
- H. Schmidbaur and A. Schier, Chem. Soc. Rev., 2012, 41, 370 RSC.
- H. Schmidbaur and A. Schier, Chem. Soc. Rev., 2008, 37, 1931 RSC.
- H. Schmidbaur, Gold Bull., 2000, 33, 3 CrossRef CAS.
- A. N. Nesmeyanov, E. G. Perevalova, Yu. T. Struchkov, M. Yu. Antipin, K. I. Grandberg and V. P. Dyadchenko, J. Organomet. Chem., 1980, 201, 343 CrossRef CAS.
- E. Zeller and H. Schmidbaur, J. Chem. Soc., Chem. Commun., 1993, 69 RSC.
- H. Schmidbaur and O. Steigelmann, Z. Naturforsch., B: J. Chem. Sci., 1992, 42, 1721 Search PubMed.
- M. Bardahi and A. Laguna, J. Chem. Educ., 1999, 76, 201 CrossRef.
- H. Schmidbaur, F. Scherbaum, B. Huber and G. Muller, Angew. Chem., Int. Ed. Engl., 1988, 27, 419 CrossRef.
- E. R. T. Tiekink, Acta Crystallogr., Sect. C: Cryst. Struct. Commun., 1989, 45, 1233 CrossRef.
- A. Deak, T. Megyes, G. Tarkanyi, P. Kiraly, L. Biczok, G. Palinkas and P. Stang, J. Am. Chem. Soc., 2006, 128, 12668 CrossRef CAS.
- A. Grohmann, J. Riede and H. Schmidbaur, J. Chem. Soc., Dalton Trans., 1991, 783 RSC.
- Y. Ma, H. Niu, X. Zhang and Y. Cai, Analyst, 2011, 136, 4192 RSC.
- S. Du, Y. Luo, Z. Liao, W. Zhang, X. Li, T. Liang, F. Zuo and K. Ding, J. Colloid Interface Sci., 2018, 523, 27 CrossRef CAS.
- R. Baron, M. Zayats and I. Willner, Anal. Chem., 2005, 77, 1566 CrossRef CAS.
- W. Schneider, K. Angermaier and H. Schmidbaur, Z. Naturforsch., B: J. Chem. Sci., 1996, 51, 801 CAS.
- T. Mathieson, A. Schier and H. Schmidbaur, Z. Naturforsch., B: J. Chem. Sci., 2000, 55, 1000 CAS.
- S. Palanisamy, X. Zhang and T. He, J. Mater. Chem. B, 2015, 3, 6019 RSC.
- J. Kang, Y. Jeong, S. Oh, H. Kim, C. Park and E. R. T. Tiekink, Bull. Korean Chem. Soc., 2010, 31, 2151 CrossRef CAS.
Footnote |
† Electronic supplementary information (ESI) available. See DOI: 10.1039/c9tb01800j |
|
This journal is © The Royal Society of Chemistry 2020 |