Current progress of Pt and Pt-based electrocatalysts used for fuel cells
Received
8th July 2019
, Accepted 21st September 2019
First published on 23rd September 2019
Abstract
Due to the growing demand for energy and impending environmental issues, fuel cells have attracted significant attention as an alternative to conventional energy technologies. As cost is the main inhibitor of this technology, low cost catalysts with high activity and stable catalytic performance are the key to large scale application of fuel cells. The development and recent advancements of Pt and Pt-based electrocatalysts are discussed in this review. Discussion is mainly focused on the structure and composition of Pt and Pt-based electrocatalysts, which significantly affect the catalytic activities and durability of fuel cell catalysts. The electrocatalysts including Pt single metal, Pt-based alloys (including noble alloys, non-noble alloys, metal oxide alloys, and non-metal alloys), and structure-controlled alloys (nanopolyhedra, nanodendrites, and hollow and core–shell structures) were discussed. The activity, stability, and efficiency of Pt and Pt-based catalysts for both the oxygen reduction reaction (ORR) and methanol oxidation reaction (MOR) as well as the correlation between the catalytic performance, structure optimization, and composition modulation of catalysts are also discussed.
1 Introduction
As the population grows, the demand for energy also grows. Environmental pollution has caused serious problems such as the greenhouse effect, acid rain, and holes in the ozone. Therefore, in the development of clean energy, the relationship between energy and the environment is a common concern and it is important to explore efficient, clean, and sustainable energy technologies.
Fuel cells have emerged as a promising technological device to supply energy to automobiles, and portable and stationary applications. Compared to combustion engines, they are environmentally friendly, steady, noise-free, and efficient when active catalysts are used on both electrodes.1 Currently, the major types of fuel cells include alkaline fuel cells (AFCs), polymer electrolyte membrane fuel cells (PEMFCs), direct methanol fuel cells (DMFCs), phosphoric acid fuel cells (PAFCs), and solid oxide fuel cells (SOFC).2,3 The major fuel cell technologies and potential applications are summarized in Fig. 1. Electrocatalysts accelerate the electrochemical reaction kinetics at the electrode–electrolyte interface of fuel cells, affecting the performance and service life of fuel cells. Therefore, tremendous efforts were made to research electrocatalysts for fuel cells (Scheme 1).
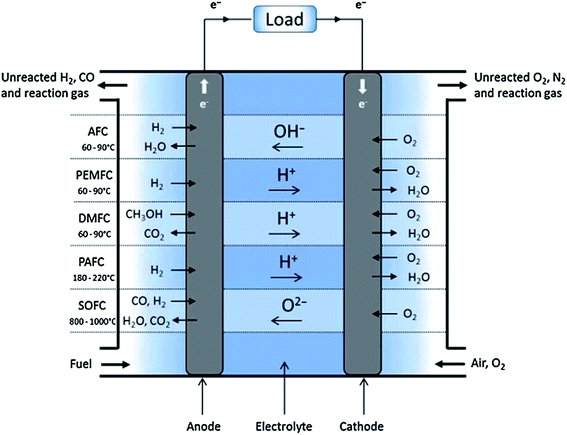 |
| Fig. 1 Schematic overview of different types of fuel cells.3 | |
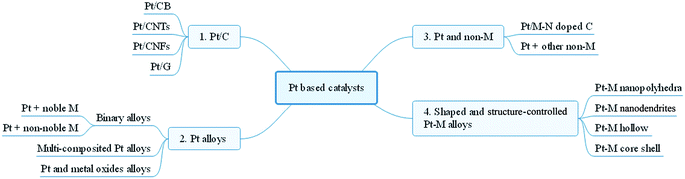 |
| Scheme 1 Current representative classes of Pt based catalysts for fuel cells. | |
Although developing non-precious metal-based electrocatalysts (such as M-N-C, M = Co, Fe, Ni, Mn, etc.) has attracted extensive attention, more detailed information about the active site formation mechanisms and structures of M-N-C electrocatalysts is still inadequate according to the current research.4
Generally, platinum nanoparticles (NPs) loaded on a carbon support (Pt/C) are most widely used as a fuel cell catalyst in scientific research and commercial cases, mostly benefiting from its higher catalytic activity and better stability than other noble metals in strongly acidic electrolytes.
However, the commercial application of Pt/C is still seriously hampered by several problems, particularly because Pt is a relatively expensive metal and is easily dissolved and aggregated under poor operating conditions, resulting in an expensive catalyst with poor electrocatalytic activity and stability.5–7 In addition, common carbon support materials for Pt and Pt-alloy catalysts suffer from corrosion due to electrochemical oxidation on the surface, which may cause the dissolution and aggregation of platinum nanoparticles.8,9 To overcome these intrinsic limitations arising from both the metal catalyst and carbon support, careful design of electrocatalysts still remains an important challenge.10
It is an effective approach to control Pt nanostructures with multi-dimensional morphologies such as core–shell, hollow, nanotube (NT),11 nanowire (NW),12 nanodendrite (ND),13 and porous structures.14,15 Each morphology can significantly increase the density of exposed Pt active sites and/or improve the stability of the catalysts by mitigating dissolution and aggregation, effectively enhancing the catalytic durability and/or activity.12,16–18 In addition, a lot of research focuses on introducing earth-abundant transition (Fe, Co, Ni, and Cu) metals with low cost and good catalytic performance to Pt to form Pt-based alloys. The synergistic effect of geometric, ligand, and electronic effects between the different components is utilized to reduce the amount of Pt and the occurrence of side reactions, ultimately improving the catalytic performance of the Pt-based alloy for fuel cells.19
This review focuses on the exciting research progress of Pt monometallic and Pt-based bimetallic catalysts in the past few years, mainly the aspects of changing the composition, structure, and morphology of Pt and Pt-based alloys to achieve excellent catalytic performance as well as long-term stability. An overview will be given on the forthcoming challenges and possible opportunities to further improve the performance of Pt and Pt-based electrocatalysts. All the efforts have contributed to the early realization of the commercial viability of fuel cells, and early realization of green energy-saving society ultimately.
2 Platinum catalysts
In 1839, William Grove,20 using platinum wire as the electrode, sulfuric acid solution as the electrolyte, and hydrogen and oxygen as raw materials, carried out the first traditional reverse reaction of electrolyzed water, producing current and successfully producing the first fuel cell model. Since then, many scientists have worked tirelessly on the application of Pt catalysts in fuel cells.
In the early stage of fuel cells' development, only black Pt NPs were used as catalysts for the anode and cathode. Pt catalysts show higher activity and durability than any other type of single noble metal catalyst for fuel cells, although a high loading of Pt catalyst is required to achieve high power density because of the large over potential of the Pt catalyst.21 However, without support materials, the increased concentration of Pt leads to agglomeration of Pt NPs and, therefore, a decrease in the surface area, hindering the catalytic performance.22
Consequently, using carbon support materials (Table 1), such as carbon black (CB), carbon nanotubes (CNTs), carbon nanofibers (CNFs), carbon nanohorns, and graphene, allows for a reduction in the amount of Pt used while enhancing the activity of electrocatalysts. Carbon materials feature high surface areas, suitable porosity, high corrosion resistance, and excellent electrical conductivity, allowing for a high dispersion of Pt NPs and accelerated electron transfer at the electrode–electrolyte interface.23 Meanwhile, oxidizing materials were also good candidates to support Pt NPs due to their improved corrosion resistance over carbon materials.24,25 Since the 1990s, CB materials have been widely used as catalyst supports for Pt and Pt-alloy catalysts in PEMFCs because of their large surface areas, excellent electrical conductivities, porous structures, and low cost, making CB one of the most widely used support materials for catalysts.26 Watanabe et al.27 prepared Pt NPs with a series of target mean-diameters and sharp size-distribution supported on graphitized carbon black (GCB). They found that GCB supports could increase the corrosion resistance of Pt/GCB at high potentials. In addition, Yu et al.28 successfully prepared Pt-nanoflowers on CB in aqueous solution, and the nanoflowers they prepared showed uniform distribution with an average particle size of 27.0 nm, contributing to remarkable area-specific and mass-specific activities for the ORR. Under normal conditions, CNTs share the same superiority with CB, but the onset corrosion potential for multi-walled CNTs is higher than that of CB, minimizing the agglomeration of Pt NPs.26 For instance, Shao et al.29 found that Pt/CNTs are more stable than Pt/C for PEMFCs, mostly attributed to the higher resistance of CNTs to electrochemical oxidation. Zhang et al.30 recently proposed a convenient and scalable approach to achieve ultrafine Pt NPs on graphene oxide-dispersed pristine carbon nanotube (G-PCNT) supports, presenting several structural advantages such as a cross-linked porous framework, large specific surface area, uniform Pt dispersion, and excellent electron conductivity. The results showed that Pt/G-PCNT has exceptional catalytic activity, good electrochemical stability, and strong poison tolerance, superior to both conventional Pt/CB and Pt/CNTs. After multi-walled CNTs were activated with KOH, a good dispersion of Pt NPs was obtained and CNTs were adopted as the support material for Pt NPs.31
Table 1 Several carbon support materials and their typical characteristicsa
Carbon support |
Surface area (m2 g−1) |
Particle size (nm) |
Conductivity (S cm−1) |
Reference |
FB = oil-furnace black.
|
Carbon black, Vulcan XC-72R FB |
254 |
30 |
2.77 |
32
|
Carbon nanotubes |
50–1000 |
1–5 μm |
0.3–400 |
33–35
|
Carbon nanofibers |
50–1000 |
30–100 μm |
200–900 |
34, 36 and 37
|
Carbon nanohorns |
300–400 |
40–50 nm |
4.95–7.07 |
38
|
Graphene |
>2000 |
10–20 nm |
103 to 104 |
37 and 39
|
Graphene shows great promise as a support material in the preparation of high performance catalysts for fuel cells because of their unique ultrathin sheet structure, ultra-high specific surface area, good electrical conductivity, and stable physical properties in comparison to other sp2 bonded carbon materials.40 Saravanan et al.41 reported that Pt NPs could be synthesized on the surface of reduced graphite oxide (RGO) through electrodeposition. The measurement results showed that RGO acts as an excellent support to anchor the Pt NPs, enhancing current density. Compared to CB and CNTs, graphene possesses a larger surface area.42 Zhou et al.43 synthesized holey graphene nanosheets (HGNs) with a meso–macroporous structure and high surface area and prepared Pt/HGN-900 with higher catalytic activity and higher stability than Pt/XC-72 and Pt/CRGO-900.
Besides choosing appropriate support materials to enhance the catalytic activity and durability, it is also important to control Pt nanostructures with multi-dimensional morphologies (such as NTs, NWs, NDs, and porous structures), which can vastly increase the exposed Pt active sites and improve the stability of the catalysts. Hoque et al.12 synthesized S-CNT supported 1D Pt nanowires (Pt NW/S-CNTs) which exhibited excellent activity, durability, and high specific activity due to the unique peculiarity of slowing down the ripening process and avoiding the dissolution/aggregation of NWs.
In summary, for fuel cells, Pt catalysts show a very high catalytic activity and stability due to their high resistance to corrosion in acid environments. Although other metals (such as Pd,44 Fe,45 Co,46,47 and Ru48) are also used as catalysts for fuel cells, their catalytic performance and corrosion resistance are inferior to those of Pt catalysts. Many detailed studies on electrocatalytic oxidation on Pt catalyst surfaces have been conducted. Research aimed at optimizing support materials and the structure for Pt NPs has been a growing field of interest in recent years.
3 Platinum alloy catalysts
Regarding the cathodes and anodes of fuel cells, pure Pt NPs are no longer state-of-the-art materials as they cannot provide sufficient catalytic performance at practical loadings in addition to their high cost. When Pt is used as a single catalytic unit, the electrocatalytic oxidation of intermediates, such as CO, can occur on the surface of Pt to occupy catalytically active sites, reducing the efficiency of Pt catalysts (Table 2). The addition of a second metal can significantly enhance its desorption as well as the oxidation of adsorbed CO, re-exposing the active sites and greatly improving the reaction kinetics.49 The catalytic activity and CO tolerance of Pt can be improved significantly by electronic or synergistic effects between other catalyst components. More recently, Pt–M (M = Au,50 Ru,51 Co,52 Fe,53 Cu,54 or Ni55) catalysts have received attention due to their open, three-dimensional structure, which exposes more active sites by reducing the number of unutilized noble metal atoms.56
Table 2 Summary of representative Pt-based catalysts for the MOR and ORR compared to commercial Pt/C
|
Representative |
Co tolerance |
Stability |
MOR |
ORR |
Reference |
Support materials |
Pt/G-PCNTs |
Strong anti-toxic ability |
Long-term stability |
|
1.7 times |
30
|
Pt/HGN-900 |
|
High stability |
2.8 times |
|
126
|
Pt alloys |
Pt-noble metals |
PtRu/Ru |
Better CO tolerance |
Long-term stability |
1.9 times |
|
59
|
Pt-non-noble metals |
PtNi |
|
Excellent stability |
|
2.5 times |
74
|
Multi-composited Pt alloys |
Au-doped PtCo/C |
Better CO tolerance |
Superior stability |
|
1.4 times |
85
|
Pt and metal oxide alloys |
PtNi(OH)2 |
|
High stability |
|
6.0 times |
96
|
Pt and non-M |
Pt/M-N doped C |
Pt/FeNC |
|
|
|
5.6 times |
97
|
Pt + other non-M |
PtNW/S-CNT |
|
Long-term stability |
|
6.7 times |
12
|
Shaped and structured Pt–M alloys |
Pt–M nanopolyhedra |
Ga–PtNi octahedra |
|
Superior stability |
|
11.7 times |
115
|
Pt–M nanodendrites |
PtPdCu nanodendrites |
Better CO tolerance |
Enhanced stability |
1.8 times |
|
109
|
Pt–M hollow |
Hollow PdPt |
|
Long-term stability |
6.2 times |
|
121
|
Pt–M core–shell |
Au@Pt |
|
Long-term stability |
3.9 times |
|
111
|
3.1 Binary alloys
Even with reduced usage of Pt and improved catalytic performance, Pt NPs still do not meet the requirements for commercialization in fuel cells. Consequently, the production of cheap and highly electro-active catalysts for fuel cells is a major challenge. Alloying Pt with other metals and controlling the structure and shape of Pt-based NPs are the two main approaches for reducing the usage of Pt and improving the catalytic performance.57
3.1.1 Platinum and noble metals.
Traditionally, PtRu electrocatalysts are used at the anode of direct methanol fuel cells (DMFCs) because Ru can promote the CO anti-poisoning properties of the nearby Pt atom.58 Bai59 synthesized PtRu/Ru catalysts with excellent catalytic activity for methanol electrooxidation by changing the amount of Ru precursor, and proved that Ru NPs led to the formation of hydroxyl species (Fig. 2).
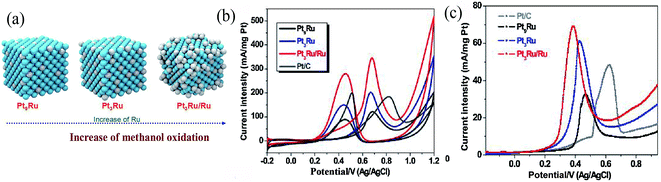 |
| Fig. 2 (a) Structural illustration of PtRu nanocrystals. (b) Cyclic voltammetry of methanol on Pt9Ru, Pt3Ru, Pt3Ru/Ru, and Pt/C electrodes in 0.5 M H2SO4 + 1 M CH3OH solution; scan rate: 0.05 V s−1. (c) CO stripping voltammetry of commercial Pt/C, Pt9Ru, Pt3Ru, and Pt3Ru/Ru.59 | |
Au has high catalytic activity for many reactions, such as CO oxidation, at the nanoscale.60 PtAu nanocatalysts exhibit synergistic catalytic effects, which alter the structure of the Pt electronic bands by changing the surface adsorption force. The synergistic catalytic effects lead to a significant enhancement in both electrocatalytic activity and stability that could improve the CO tolerance of Pt catalysts. Synthesized by Feng et al.,61 a Pt1.0Au/RGO electrocatalyst showed higher catalytic activity and stability compared with pure Pt, suggesting that the addition of Au could greatly improve the catalytic properties. The improved electrocatalytic properties of Pt1.0Au/RGO were mainly related to the suppressed CO formation, rather than the facile CO removal, on the surface of Pt. Deng et al.62 recently synthesized PtAu/TiO2 NW catalysts with different Pt/Au/Ti ratios, and they found that the Pt1Au1/(TiO2)1 NWs exhibited excellent mass activity and specific activity compared to commercial Pt/C, owing partly to the synergistic effect of TiO2, and exhibited increased stability, attributed to the Au and C skin (Fig. 3).
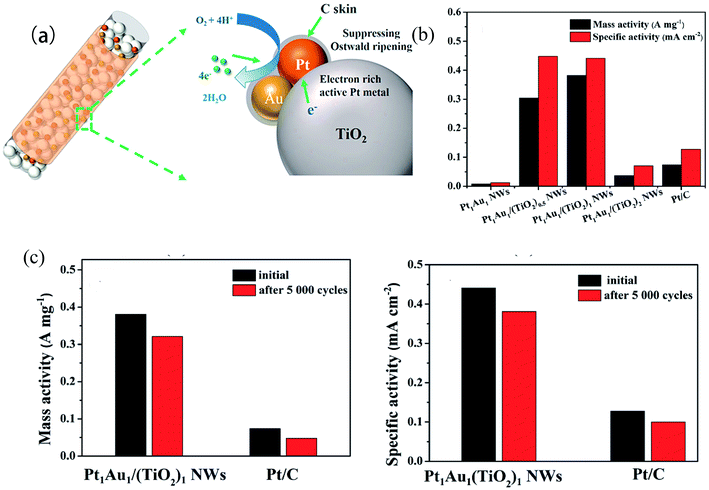 |
| Fig. 3 (a) Structural illustration of PtAu/(TiO2) NWs. (b) Mass activities and specific activity of 20% Pt/C and PtAu/TiO2NWs. (c) Mass and specific activities of 20% Pt/C and Pt1Au1/(TiO2)1 NW catalysts before and after the durability test.62 | |
One of the most effective candidates for alloying with Pt is Pd due to its identical facets and similar lattice constant, allowing for bimetallic nanocrystals with single crystallinity.63 Cho et al.10 recently proposed a new structure of bimetallic catalysts by a facile wet chemical method, realizing synergistic effects on the ORR performance through effective integration of the fine-sized (2–5 nm) Pt NPs (Fig. 4). PdPt bimetallic nanocrystals exhibit substantially enhanced ORR activity and durability in comparison to commercial Pt/C.
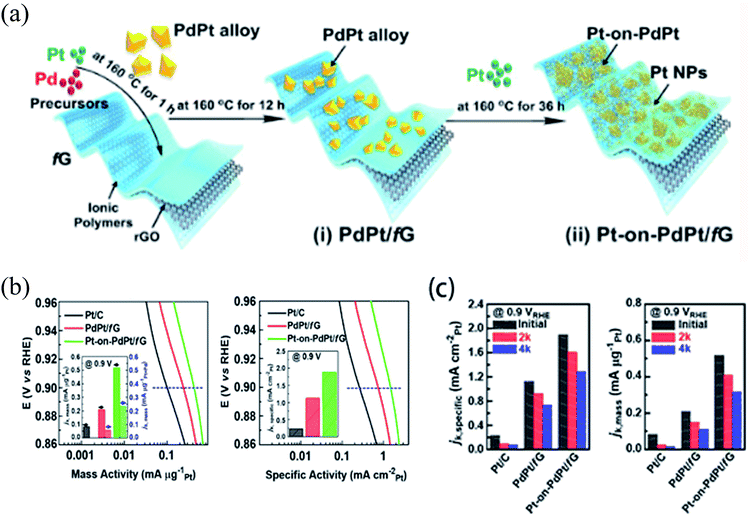 |
| Fig. 4 (a) Schematic depiction of the synthesis of (i) PdPt/fG and (ii) Pt-on-PdPt/fG. (b) Tafel plots of the mass activity and specific activity of Pt/C, PdPt/fG, and Pt-on-PdPt/fG (inset: mass and specific activities at 0.9 VRHE, which are given as kinetic current (ik) normalized by the loading mass of Pt and the ECSA, respectively). (c) Specific activity and mass activity of Pt/C, PdPt/fG, and Pt-on-PdPt/fG. The durability tests were performed by cycling between 0.6 and 1.2 VRHE at a scan rate of 50 mV s−1 at room temperature in an O2-saturated 0.1 M HClO4 solution.10 | |
These non-Pt noble metals, Ru, Au, and Pd, are added to modify Pt catalysts, proving that the addition of a second noble metal can cut down the amount of Pt while maintaining high electrocatalytic activities and durability as well as suppressing CO poisoning.
3.1.2 Platinum and non-noble metals.
The combination of non-noble metals with Pt can increase its catalytic efficiency and reduce the cost of the catalyst. In addition, the integration of other metals can sharply increase the utilization efficiency of Pt atoms.19 At the moment, a number of different non-noble metals are studied by researchers for Pt-based electrocatalysts, including Sc, Ti, V, Cr, Mn, Fe, Co, Ni, Cu, Zn, etc.45,64–71 However, Pt–Ni, Pt–Cu, Pt–Co, and Pt–Fe, which have lower cost and a more outstanding performance the above non-noble metals, show enhanced catalytic activities in comparison with their monometallic counterparts. Hence four representative non-noble metals are studied in this paper.
In recent years, many researchers have considered introducing Ni to form Pt–Ni alloys with Pt NPs. Pt–Ni alloy electrocatalysts are thought to have high performance and good stability for the following two reasons.72,73 First, the electronic characteristics of the surface Pt atoms can be modified with Ni atoms because of the shift in the electron transfer from Ni to Pt. Second, the synergistic effect of Pt and Ni is present on the surfaces of the catalysts. Henning et al.74 successfully synthesized unsupported Pt–Ni aerogels as the ORR catalyst in a PEMFC cathode. The catalyst was optimized by adding K2CO3 into the catalyst ink, which was later removed by acid washing of the catalyst coated membrane, achieving high catalytic performance and excellent stability. Beermann et al.75 prepared shape-controlled octahedral Pt–Ni alloy NPs via thermal annealing, and this special structure exhibited remarkably high activity for the ORR (about 25 times that of commercial Pt/C).
Wang et al.76 recently prepared a novel Pt/Cu-zeolite A/graphene catalyst via adopting a conductive and porous dual template Cu-ZEA/RGO as a support for depositing Pt NPs. The prepared composite catalyst exhibited obviously enhanced current and higher electrocatalytic activity. Maya-Cornejo et al.77 synthesized a PtCu/C catalyst by using a galvanic displacement route, obtaining fine-sized NPs with a semi-spherical morphology. The presence of Cu promotes ethanol oxidation by hindering the Pt–H adsorption at low over-potentials. However, catalysts without carbon material supports have been highlighted recently due to avoiding negative influences of carbon materials on the stability, such as carbon corrosion. Kim et al.54 recently reported a support-less three-dimensional foam of Cu electrodeposited on a micro-porous layer/carbon paper, using hydrogen bubbles as a template and then displaced with Pt by simple immersion. The obtained PtCu alloy catalyst exhibited superior durability.
In addition, Orazi et al.78 used Density Functional Theory (DFT) calculations to investigate methanol adsorption on the PtCo (111) surface. They proved that the most favorable site for CH3OH adsorption is on top of the Co atom. The C–H bonds are strengthened whereas the C–O and O–H bonds are weakened. Consequently, the PtCo electrocatalyst is theoretically proven to be a better choice to avoid CO poisoning. Sorsa et al.79 adopted a potentiostatic electrodeposition method to prepare PtCo, PtNi and PtCu catalysts for the ORR in PEMFCs, which all presented higher mass activities towards the ORR than commercial Pt/C, with PtCo being the most active (Fig. 5).
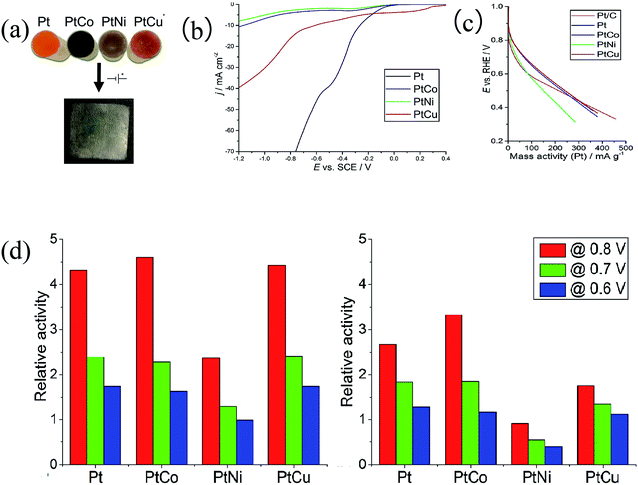 |
| Fig. 5 (a) Schematic depiction of the synthesis of Pt, PtCo, PtNi, and PtCu. (b) Linear sweep voltammograms for liquid crystalline solutions containing Pt, PtCo, PtNi and PtCu with a scan rate of 100 mV s−1. (c) ORR activities indicated in sweep voltammograms (5 mV s−1) of the commercial reference material (Pt/C) and the different electrodeposited catalysts in a three-electrode cell. (d) The relative ORR activities of the electrodeposited catalysts normalized with respect to the commercial reference at different potentials vs. RHE before and after 3000 cycles.79 | |
Furthermore, the development of Pt alloy catalysts relies on the design of architectures with highly active surfaces and optimized utilization of the expensive element. A Pt-based alloy with cheap transition metals normally could be designed by controlling the atomic ratio of metals, structure, and morphology via various synthesis methods to obtain better catalytic performance and excellent durability.
Becknell et al.80 tuned the three dimensional Pt anisotropy of Pt–Ni rhombic dodecahedra by controlling the ratio, obtaining a completely hollow nanoframe or a new architecture nanoframe with about 10 times higher specific and 6 times higher mass activity for the ORR than Pt/C. Flórez-Montaño et al.81 synthesized Ni@Pt/RGO nanodisks and proved that the electronic interaction between Pt and Ni can significantly increase the CO tolerance of the catalyst. Yue et al.82 reported the preparation of Pt@Cu NPs with a low Pt loading via an electrochemical method, which exhibited excellent catalytic performance as well as excellent stability and reusability.
Therefore, the Pt-based modified binary catalyst is fundamentally necessary to optimize the microstructure of Pt, lower the Pt loading, and meet the catalytic performance and stability requirements of fuel cells.
3.2 Multi-composited platinum alloys
The Pt-based binary catalyst reduces the poisoning of the catalyst by the reaction intermediates to improve the catalytic efficiency to a certain extent, but the ability to promote the catalytic activity of Pt metal species is limited. However, the composite single metal in a strongly acidic or strongly alkaline medium is easily dissolved, leading to lower overall efficiency of the binary catalyst. Adding a non-precious metal into the nanostructure to synthesize trimetallic nanocrystal catalysts, such as PtPd–Cu,83 Pd@AuCu,84 Au-doped PtCo,85 and Pt–Au–Si,86 successfully increases the activity and stability.87 Through the combination of screening, a relatively large variety of multi-composited Pt alloy catalyst systems were obtained. Due to the synergistic effect of the metals, the combination of bi-functional, electronic, and hydrogen overflow effects allows some catalysts to exhibit superior catalytic performance for the ORR.
Research has shown that the improvement of catalytic activity on Pt-based electrocatalysts occurs when a secondary metal, Ru, Pd, Au, Rh, Fe, Cu, or Ni, is added to modify the Pt NPs by geometric and electronic interactions. Other researchers have found that ternary catalytic metal materials, such as ternary Pt–Rh–SnO2/C catalysts synthesized by employing a modified polyol method, which shows the highest capability to break the C–C bond of the ethanol molecule efficiently, have a maximum activity obtained at a composition ratio of Pt
:
Rh
:
Sn = 3
:
1
:
4.88 Ternary materials of Pt–Sn–M (M = Fe, Ni, Pd, and Ru) were synthesized by Almeida et al., and evaluated by fluorescence to optimize the catalytic activity, and it was found that Fe-containing catalysts exhibited the highest activity.89
3.3 Platinum and metal oxides
Numerous studies have highlighted the function of Pt catalysts via the preparation of Pt-based binary or ternary catalysts, including metal alloys or metal oxides, for catalyzing the oxidation or reduction process.24 However, carbon corrosion would cause serious Pt loss and cell performance decay, an issue which must be addressed.90 In order to adopt a more stable support material, alternative materials such as metal oxides (TiO2,90 WO3, Fe2O3, and SnO2 (ref. 91)) have been used to take advantage of their inertness in harsh oxidative environments, whereas Ni(OH)2 is more likely to rely on the features of Ni, not emphasizing Ni(OH)2 as a support material.
Among these oxide materials, TiO2 possesses great mechanical strength and high stability in acidic and oxidizing environments which is beneficial to the deposition and dispersion of Pt NPs. Titanium is generally used as a catalyst support in the form of nano-materials, such as nanotubes, nanowires, and nanosheets, to make a regular arrangement. Wu et al.92 designed a carbon–TiO2 composite as a Pt support, with mesoporous TiO2 as the core and partially coated carbon as the shell. Electrochemical measurements showed that Pt–C/TiO2 can enhance the activity and stability for the methanol oxidation reaction, which were 3.0 and 1.5 times higher than those of Pt/TiO2 and Pt–C. Meanwhile, Pt–C/TiO2 was 6.7 times more stable than Pt–C after 2000 cycles.
Tungsten oxide, WO3, has been considered the most promising catalyst support material for fuel cells.93 Pt/WO3-G catalysts for the ORR were studied by Jin et al.,94 in which WO3 modified graphene (WO3-G) is adopted as the carrier. The measurement results indicate that the Pt/WO3-G catalyst with the coexistence of Pt particles and WO3 has narrower distribution of Pt particles with an average particle size of 3.9 nm. The Pt/WO3-G catalysts exhibit better electrocatalytic activity with higher current densities at lower over-potentials and a faster kinetics process compared to the Pt/G catalyst. Jin et al.94 have proved that WO3–Pt/C shows higher kinetic activity for the ORR in a single cell than other conventional Pt/C catalysts. Furthermore, the electrocatalytic activity was also enhanced greatly due to the interaction between Pt and WO3, in which the WO3 nanostructures can remove oxygen atoms from platinum and increase hydrogen spillover.
A large effort has been made to find abundant and cheap metals or metal oxides to substitute for noble metals. Pt-based catalysts containing iron and iron oxides with a high surface area, porosity, and electro-positivity as a co-catalyst or conductive support are promising catalysts for the oxygen evolution reaction.95 Almeida et al.1 recently reported that iron oxides provide outstanding activity during the oxidation of ethanol, demonstrating that transition metal oxides may be a promising alternative for the oxidation of fuels. Iron oxide–C was prepared by thermal decomposition of polymeric precursors, and then the subsequent deposition of Pt and PtSn onto the γ-Fe2O3–C substrate was carried out by a microwave-assisted method. The application of γ-Fe2O3–C can decrease the cost of the catalyst and provide a good platform to double the DEFC activity of PtSn, which is now considered to be one of the best electrocatalysts for the electrooxidation of ethanol. Yang et al.24 successfully synthesized a commercial Pt black catalyst modified with PbO2 NPs with a mean size of 4.59 nm as well as high catalytic activity and long term durability. A Pt + SnO2/C electrocatalyst containing cubic Pt NPs with preferential (100) orientation25 was prepared by an alcohol-reduction process. Ni(OH)2 NPs surrounded by ultra-low Pt content supported on functionalized carbon were prepared by a scalable synthesis method. The correlation of strain and d-vacancies with ORR activity and stability is revealed to be a volcano-type correlation. The catalyst had about 6 times higher surface area and mass-normalized activity compared to Pt/C as well as improved durability.96
4 Platinum and non-metals
Due to high cost and low reserves of Pt, many researchers have turned their attention to utilizing inexpensive and easily mass-produced materials such as carbon materials, metal-nitrogen doped carbon catalysts, non-metallic heteroatom-doped carbon catalysts, nitrides, transition metal chalcogenides, and carbon layer-coated non-noble metal compounds to composite with Pt. Support materials play an important role in developing high performance catalysts, since the structure and surface properties of the support greatly affect the size and dispersion quality of metal nanoparticles.92 Among these Pt and non-metal catalysts, metal-nitrogen-doped carbon catalysts and heteroatom-doped carbon catalysts have attracted the most attention because of their high activity and stability.
4.1 Pt/metal-nitrogen doped carbon
The nitrogen atom has a similar atomic radius to the carbon atom, and the electronegativity of nitrogen is greater than that of carbon. When nitrogen is incorporated into a carbon material, the electrons are enriched around the carbon atoms due to the strong electronegativity of the nitrogen atoms, so that the adjacent carbon atoms are positively charged, which facilitates the adsorption of oxygen molecules and further accelerates the ORR. Recently, Pt/metal-nitrogen doped carbon (Pt/M-N-C) has drawn the attention of researchers due to its excellent catalytic activity and stability.97,98
Li et al.99 prepared Pt NPs loaded on nitrogen-doped carbon/Ni nanofibers (Ni-NCNFs-Pt) as an efficient HER electrocatalyst by electrospinning combined with calcination and chemical reduction processes. The introduction of the conductive NCNF substrate facilitated fast electron transport (Fig. 6). Luo et al.100 designed a highly dispersed HD-Pt/TiN material, which exhibits excellent selectivity toward hydrogen oxidation and greater ORR activity than commercial 20 wt% Pt/C. They provided a new strategy to design selective catalysts toward hydrogen oxidation and the ORR, using an oxygen-tolerant anode catalyst to improve the stability during startup and shutdown, making PEMFCs more affordable and practical (Fig. 6(a)). Cui et al.52 fabricated Co NP doped fluffy N-doped mesoporous carbon (Co/mCN) by a facile one-pot carbonization method. Furthermore, the Pt@Co/mCN nanocatalyst was prepared through a facile spontaneous displacement reaction by using the Co/mCN material and H2PtCl6. The Pt@Co/mCN nanocatalyst showed excellent catalytic activity and reusability, probably due to the isolated Pt on the surface of the Co NPs and the highly dispersed Pt@Co NPs on the surface of the fluffy mCN support (Fig. 6(c)).
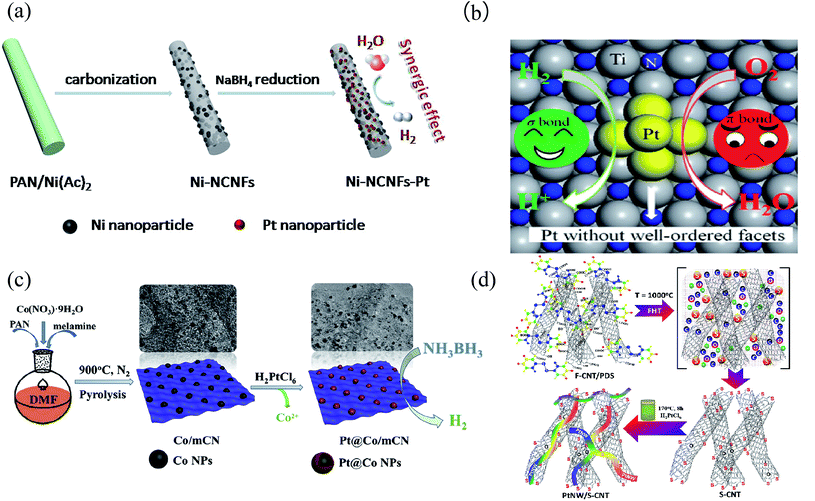 |
| Fig. 6 (a) Synthesis of Pt NPs supported on nitrogen-doped carbon/Ni nanofibers via an electrospinning, calcination, and chemical reduction process.99 (b) Structure illustration of the distinct structure sensitivity between the σ-type bond in H2 and the π-type bond in O2 of HD-Pt/TiN.100 (c) Fabrication procedure and the application of the Pt@Co/mCN nanocatalyst.52 (d) Schematic of the materials produced at different stages of S-CNT and PtNW/S-CNT synthesis.12 | |
4.2 Pt and other non-metals
Non-metallic heteroatom-doped carbon nanocatalysts, such as nitrogen,101 sulphur,102 and boron,103 are also considered to be a class of highly promising fuel cell catalysts due to their high catalytic activity and outstanding resistance to fuel permeation and CO poisoning.
Chen et al.103 recently synthesized N-doped graphene quantum dots (NGQDs) by hydrothermal treatment of citric acid and urea, which is used as a support for platinum NPs. The shift in Pt 4f binding energy suggests that charge transfer from Pt to NGQDs is facilitated by the more electronegative N dopants.
The electronegativity of sulfur (2.58) is very close to the electronegativity of carbon and its atomic radius is greater than the atomic radius of carbon, indicating that incorporating sulfur into the carbon skeleton will strain and stress the carbon skeleton and, therefore, alter the carbon matrix charge distribution and geometry. Hoque et al.12 reported that PtNW/S-CNT synthesized by a modified solvothermal method with a unique web-like 3D architecture is beneficial for the ORR. The PtNW/S-CNT catalyst showed very high specific activity (1.61 mA cm−2) in comparison to Pt/C (0.24 mA cm−2) (Fig. 6(d)).
In addition, boron (B) is also considered to be a promising heteroatom for doping of carbon materials to modify the chemically inert sp2 carbon structure and thus activate the abundant free-flowing π electrons.104,105
5 Shape and structure-controlled Pt–M alloy catalysts
In recent years, in order to further improve the electrocatalytic activity of Pt alloy catalysts for fuel cells, many researchers have made great progress by precisely controlling the synthesis of Pt based alloys to tailor their shapes and structures while enhancing the catalytic activity, reducing the amount of Pt, and avoiding CO poisoning. Pt–M alloys with different shapes and structures (nanopolyhedra,80 nanodendrites,13 hollow,106,107 and core–shell structure108) have become a popular area of research.
5.1 Pt–M nanopolyhedra
Present research on nanopolyhedra is mainly conducted by controlling the shape and surface structure to improve ORR activity and stability.112 In general, smaller sized NPs result in a larger surface area which results in higher electrocatalytic activity. The alloys of Pt and other precious metals in the nanopolyhedra form tend to converge on the edges or vertices to enhance the catalytic performance.
Beermann et al.75 succeeded in synthesizing shape-controlled octahedral Pt–Ni alloy NPs, using a wet-chemical approach via thermal annealing treatments, exhibiting remarkably high activities for the ORR compared to Pt/C. Tian et al.113 recently synthesized a sandwich-structured, highly uniform icosahedral Pt2.1Ni catalyst enclosed by Pt (111) facets via a hot injection method, also having superior ORR activity and especially stability over commercial Pt/C. Li et al.114 reported that Cu-rich PtCu octahedral alloys, prepared by a composition-driven shape evolution route, exhibited remarkable bifunctional performance including enhanced activity and stability toward the MOR and ORR compared to commercial Pt or Pt/C. Using Pt–M alloyed nanopolyhedra has been deemed one of the most promising strategies to maximize electrocatalytic activity.
In Pt–Ni based catalysts, Ni atoms are easily dissolved in the electrolyte, resulting in degradation of the catalyst. Lim et al.115 elucidated that Ga-doping in Pt–Ni octahedral NPs decreases the dissolution rate, resulting in an 11.7-fold improvement in the mass activity (1.24 A mgPt−1), a 17.3-fold enhancement in the specific activity (2.53 mA cm−2), and superior stability to PtNi/C and Pt/C. Huang et al.116 compared various transition metal surface-doped Pt3Ni octahedra supported on carbon and found that Mo–Pt3Ni/C showed the best ORR performance. It had a super high specific activity of 10.3 mA cm−2 and a super high mass activity of 6.98 A mgPt−1 as well as substantially improved stability.
5.2 Pt–M nanodendrites
Pt–M nanodendritic structures have also attracted attention due to their excellent electrocatalytic performance.70,117 These granular nanoparticles are small in size and agglomerate easily, so they need to be loaded onto a carrier during electrocatalytic performance measurement. The branches of the dendritic structure are separated from each other and have a large specific surface area. Dendritic NPs are generally larger in size, and thus, don't require a carrier for the electrocatalytic performance test. As a kind of fuel cell catalyst, dendritic NP electrocatalysts also have a high specific surface area and orientation growth, drawing the attention of many researchers.
Chang et al.109 prepared hierarchical PtPdCu nanodendrites (NDs) via a two-step synthetic route including an electrodeposition and wet-chemical method for the MOR. The PtPdCu NDs showed superior MOR mass activity and specific activity, which were about 1.78 times and 2.14 times higher than those of commercial Pt/C, respectively, along with excellent stability and better CO tolerance (Fig. 7(a)–(c)).
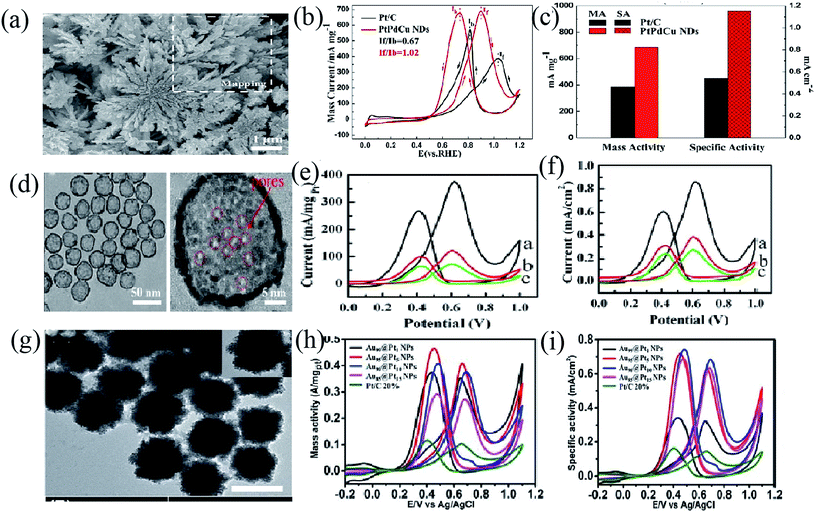 |
| Fig. 7 (a) The SEM image of the hierarchical PtPdCu NDs achieved after being etched in 0.1 M HNO3 with a Cu atomic content of ∼21.51 at%. (b) The CVs of PtPdCu NDs and commercial Pt/C obtained in 0.5 M H2SO4 + 1.0 M CH3OH at 50 mV s−1. (c) The mass activity and specific activity of the PtPdCu NDs and commercial Pt/C.109 (d) TEM images of the prepared HMPNNs. (e) and (f) CVs for the oxidation of 0.5 M methanol in H2SO4 solution (0.5 M) (HMPNNs a, solid Pt–Ni nanoparticles b, and commercial Pt catalyst c, respectively).110 (g) TEM image of Au90@Pt10 NPs. (h) Mass activity CVs and (i) specific activity CVs of the four types of Au@Pt NP catalysts and the commercial Pt/C catalyst in 0.5 M H2SO4 + 0.5 M CH3OH.111 | |
The membrane-electrode-assembly (MEA) test suggested that the 3-D interconnected dendrite structures of Pt–Pd nanodendrites have superiority over Pt/C in reducing the mass transport and ohmic polarization, both of which benefit the ORR in the cathode of PEMFCs.118
5.3 Pt–M hollow structures
The activity of Pt-based catalysts relies on their surface areas and shapes to a great extent.119 Hollow structures have received much attention due to the high surface to volume ratios, low densities, elevated utilization of Pt, and abundant active sites, thereby enhancing the catalytic performance of catalysts and reducing the amount of Pt.106 Recently, Hu et al.120 synthesized a PtNi/G catalyst through the galvanic replacement method, exhibiting high activity toward the MOR on account of its unique hollow structure and the synergy between Pt and Ni (Fig. 7(d)–(f)). More recently, Fang and co-workers demonstrated excellent catalytic activity and stability of hollow PtPd structures, which have high active surface areas, toward the MOR. They prepared hollow PtPd alloy nanostructures by adjusting the reduction rate difference.121
Nanoframe structures, a class of hollow nanostructures, have attracted increasing attention in the catalyst field. The nanoframe structure enlarges the specific surface area of metal particles and their confinement effect improves the collision probability between catalyst molecules and the catalyst surface, thus enhancing the catalytic performance.107 Aram et al.122 designed a fortified Pt-rich skeletal PtNi alloy nanoframe catalyst by etching to selectively remove the Ni-rich phase, which could enhance the catalytic activity significantly for the ORR compared to commercial Pt/C catalysts. The stability of Pt-based nanoframe catalysts can be structurally fortified by introducing an inner-positioned metal nanoparticle support. For example, Kwon et al.123 synthesized a PtCuCo nanoframe (Co-PtCu RNF) with a reinforced vertex structure by in situ doping of Co into the PtCu alloy nanoframe, exhibiting superior excellent activity and durability after 1000 cycles for the ORR and MOR. Park et al.123 reported a robust PtCu@PtCuNi dendrite@frame nanocatalyst, whose structure was strengthened with inner PtCu dendrites and its catalytic activity and stability are much higher than those of commercial Pt/C for the ORR.
Furthermore, the design of Pt-based catalysts with hollow structures has economic advantages, especially considering the high cost and limited reserves of Pt metal. From the above studies, hollow nanostructures not only reduce Pt loadings used in fuel cell electrodes compared to other normal structures, but also enhance the catalytic performance towards the MOR. However, the stability of the hollow structure, including the nanoframe structure, could become much worse due to their intrinsically infirm stability during long-term electro-cycling. Therefore, further studies on the structural fortification of hollow structures to enhance the durability of Pt-based electrocatalysts are a current research target.
5.4 Pt–M core–shell structures
The primary goal of designing core–shell Pt-based alloys is to significantly reduce the Pt loading in fuel cell systems and overcome the limitations of both catalytic activities and stabilities of Pt based catalysts. For core–shell structures, single-metal or alloy NPs have been prepared as the core to adjust the electronic structure and lattice parameters of thin Pt shells.22
First of all, choosing core materials is very important for core–shell Pt-based catalysts. Pd is regarded as the most stable metal as a core material under acidic conditions. In addition, the nanoparticle size of Pd is easily controlled, making it an ideal core metal.22 More recently, Tan et al.111 successfully prepared Au@Pt NPs with outstanding electrochemical performances by the seed-mediated growth method. This core–shell structure is helpful for avoiding agglomeration, allowing for better stability towards the MOR by efficiently removing intermediates (Fig. 7(g) and (h)). Alternatively, Ni, a kind of cheap 3d transition metal, was used as the core material to avoid the use of other expensive core materials. Fetohi et al.124 were very interested in the effects of Ni on the electrocatalytic activity of Pt based core–shell catalysts, and found that when the atomic ratio of Pt
:
Ni was 1
:
3, Ni@Pt/C exhibited a high oxidation current density and stability.
Secondly, shell thickness may be an important factor for improving the activity and long-term stability. Chepkasov and co-workers125 investigated the structural and electronic properties of PtPd NPs by using molecular dynamics simulations and DFT calculations, and they found that the Pd@Pt NPs with a monolayer thickness of the Pt shell turned to be the most stable. Based on the conclusions of DFT calculations and the experimental studies, Jang et al.45 synthesized core–shell Fex@Pt NPs, via one-pot ultrasound-assisted polyol synthesis (UPS) reactions, and found that PtFe1.2/C with a monolayer thick Pt shell and 55% Fe content showed the highest activity for the ORR with excellent durability.
6 Conclusions and prospects
Pt-based catalysts are the most effective catalysts for fuel cells, and the modification of Pt-based catalysts to improve their catalytic efficiency and reduce cost is the key factor to the commercialization of fuel cells. Different compositions, structures, and morphologies of the catalyst can promote the exposure of active sites to increase the catalytic activity and enhance the long-term stability. The optimization of the high specific surface area and the electronic effect can be combined to promote the catalytic efficiency. However, in the progress of development of Pt-based catalysts, there is still some difficulty, such as the development of support materials, non-noble metal catalyst research and development, high-index crystal synthesis, and high-precision control of particle size. Based on the study of Pt-based catalyst modification, we believe that with continuous advancements in technology and characterization of materials combined with continuous theoretical investigation, the application of fuel cells to new energy systems has a bright future.
Conflicts of interest
There are no conflicts to declare.
Acknowledgements
Support of the National Natural Science Foundation of China (21908017 and 21902021), the Fundamental Research Funds for the Central Universities (DUT18LK21 and DUT18LK15), the Natural Science Foundation of Liaoning Province (20180510020), and the Supercomputing Center of Dalian University of Technology for this work is gratefully acknowledged.
References
- T. S. Almeida, C. Garbim, R. G. Silva and A. R. De Andrade, Addition of iron oxide to Pt-based catalyst to enhance the catalytic activity of ethanol electrooxidation, J. Electroanal. Chem., 2017, 796, 49–56 CrossRef CAS.
- C. Zhang, X. Shen, Y. Pan and Z. Peng, A review of Pt-based electrocatalysts for oxygen reduction reaction, Front. Energy, 2017, 11(3), 268–285 CrossRef.
- N. Seselj, C. Engelbrekt and J. Zhang, Graphene-supported platinum catalysts for fuel cells, Sci. Bull., 2015, 60(9), 864–876 CrossRef.
- Y. Nie, L. Li and Z. Wei, Recent advancements in Pt and Pt-free catalysts for oxygen reduction reaction, Chem. Soc. Rev., 2015, 44(8), 2168–2201 RSC.
- D. Li, C. Wang, D. S. Strmcnik, D. V. Tripkovic, X. Sun, Y. Kang, M. Chi, J. D. Snyder, D. van der Vliet, Y. Tsai, V. R. Stamenkovic, S. Sun and N. M. Markovic, Functional links between Pt single crystal morphology and nanoparticles with different size and shape: the oxygen reduction reaction case, Energy Environ. Sci., 2014, 7(12), 4061–4069 RSC.
- G. Andreadis and P. Tsiakaras, Ethanol crossover and direct ethanol PEM fuel cell performance modeling and experimental validation, Chem. Eng. Sci., 2006, 61(22), 7497–7508 CrossRef CAS.
- Y. Hao, X. Wang, J. Shen, J. Yuan, A. J. Wang, L. Niu and S. Huang, One-pot synthesis of single-crystal Pt nanoplates uniformly deposited on reduced graphene oxide, and their high activity and stability on the electrocatalytic oxidation of methanol, Nanotechnology, 2016, 27(14), 145602 CrossRef PubMed.
- E. Antolini, Composite materials: an emerging class of fuel cell catalyst supports, Appl. Catal., B, 2010, 100(3–4), 413–426 CrossRef CAS.
- H. Lv and S. Mu, Nano-ceramic support materials for low temperature fuel cell catalysts, Nanoscale, 2014, 6(10), 5063–5074 RSC.
- K. Y. Cho, Y. S. Yeom, H. Y. Seo, A. S. Lee, X. Huy Do, J. P. Hong, H.-K. Jeong, K.-Y. Baek and H. G. Yoon, Fine-sized Pt nanoparticles dispersed on PdPt bimetallic nanocrystals with non-covalently functionalized graphene toward synergistic effects on the oxygen reduction reaction, Electrochim. Acta, 2017, 257, 412–422 CrossRef CAS.
- L. X. Ding, A. L. Wang, G. R. Li, Z. Q. Liu, W. X. Zhao, C. Y. Su and Y. X. Tong, Porous Pt-Ni-P composite nanotube arrays: highly electroactive and durable catalysts for methanol electrooxidation, J. Am. Chem. Soc., 2012, 134(13), 5730–5733 CrossRef CAS PubMed.
- M. A. Hoque, F. M. Hassan, A. M. Jauhar, G. Jiang, M. Pritzker, J.-Y. Choi, S. Knights, S. Ye and Z. Chen, Web-like 3D Architecture of Pt Nanowires and Sulfur-Doped Carbon Nanotube with Superior Electrocatalytic Performance, ACS Sustainable Chem. Eng., 2017, 6(1), 93–98 CrossRef.
- L. B. Venarusso, C. V. Boone, J. Bettini and G. Maia, Carbon-supported metal nanodendrites as efficient, stable catalysts for the oxygen reduction reaction, J. Mater. Chem. A, 2018, 6(4), 1714–1726 RSC.
- S. Mi, N. Cheng, H. Jiang, C. Li and H. Jiang, Porous Pt3Ni with enhanced activity and durability towards oxygen reduction reaction, RSC Adv., 2018, 8(28), 15344–15351 RSC.
- V. Grozovski, H. Kasuk, J. Nerut, E. Härk, R. Jäger, I. Tallo and E. Lust, Oxygen Reduction at Shape-Controlled Platinum Nanoparticles and Composite Catalysts Based on (100)Pt Nanocubes on Microporous-Mesoporous Carbon Supports, ChemElectroChem, 2015, 2(6), 847–851 CrossRef CAS.
- Z. Yao, R. Yue, C. Zhai, F. Jiang, H. Wang, Y. Du, C. Wang and P. Yang, Electrochemical layer-by-layer fabrication of a novel three-dimensional Pt/graphene/carbon fiber electrode and its improved catalytic performance for methanol electrooxidation in alkaline medium, Int. J. Hydrogen Energy, 2013, 38(15), 6368–6376 CrossRef CAS.
- J. J. Pei, J. J. Mao, X. Liang, Z. B. Zhuang, C. Chen, Q. Peng, D. S. Wang and Y. D. Li, Ultrathin Pt-Zn Nanowires: High-Performance Catalysts for Electrooxidation of Methanol and Formic Acid, ACS Sustainable Chem. Eng., 2018, 6(1), 77–81 CrossRef CAS.
- H. Lv, J. Wang, Z. Yan, B. Li, D. Yang and C. Zhang, Carbon-Supported Pt-Co Nanowires as a Novel Cathode Catalyst for Proton Exchange Membrane Fuel Cells, Fuel Cells, 2017, 17(5), 635–642 CrossRef CAS.
- X. L. Tian, L. Wang, P. Deng, Y. Chen and B. Y. Xia, Research advances in unsupported Pt-based catalysts for electrochemical methanol oxidation, J. Energy Chem., 2017, 26(6), 1067–1076 CrossRef.
- A. J. Appleby, From Sir William Grove to today-fuel-cells and the future, J. Power Sources, 1990, 29(1–2), 3–11 CrossRef CAS.
- X. Yu and S. Ye, Recent advances in activity and durability enhancement of Pt/C catalytic cathode in PEMFC, J. Power Sources, 2007, 172(1), 133–144 CrossRef CAS.
- N. Jung, D. Y. Chung, J. Ryu, S. J. Yoo and Y.-E. Sung, Pt-based nanoarchitecture and catalyst design for fuel cell applications, Nano Today, 2014, 9(4), 433–456 CrossRef CAS.
- E. Antolini, Carbon supports for low-temperature fuel cell catalysts, Appl. Catal., B, 2009, 88(1–2), 1–24 CAS.
- W.-H. Yang, Q.-H. Zhang, H.-H. Wang, Z.-Y. Zhou and S.-G. Sun, Preparation and utilization of a sub-5 nm PbO2 colloid as an excellent co-catalyst for Pt-based catalysts toward ethanol electro-oxidation, New J. Chem., 2017, 41(20), 12123–12130 RSC.
- R. M. Antoniassi, J. C. M. Silva, A. Oliveira Neto and E. V. Spinacé, Synthesis of Pt+SnO2/C electrocatalysts containing Pt nanoparticles with preferential (100) orientation for direct ethanol fuel cell, Appl. Catal., B, 2017, 218, 91–100 CrossRef CAS.
- Y. Luo and N. Alonso-Vante, The Effect of Support on Advanced Pt-based Cathodes towards the Oxygen Reduction Reaction. State of the Art, Electrochim. Acta, 2015, 179, 108–118 CrossRef CAS.
- M. Watanabe, H. Yano, H. Uchida and D. A. Tryk, Achievement of distinctively high durability at nanosized Pt catalysts supported on carbon black for fuel cell cathodes, J. Electroanal. Chem., 2018, 819, 359–364 CrossRef CAS.
- S. Yu, F. Li, H. Yang, G. Li, G. Zhu, J. Li, L. Zhang and Y. Li, Pt-nanoflower as high performance electrocatalyst for fuel cell vehicle, Int. J. Hydrogen Energy, 2017, 42(50), 29971–29976 CrossRef CAS.
- Y. Y. Shao, G. P. Yin, Y. Z. Gao and P. F. Shi, Durability study of Pt/C and Pt/CNTs catalysts under simulated PEM fuel cell conditions, J. Electrochem. Soc., 2006, 153(6), A1093–A1097 CrossRef CAS.
- X. Zhang, J. Zhang, H. Huang, Q. Jiang and Y. Wu, Platinum nanoparticles anchored on graphene oxide-dispersed pristine carbon nanotube supports: high-performance electrocatalysts toward methanol electrooxidation, Electrochim. Acta, 2017, 258, 919–926 CrossRef CAS.
- C. He, S. Song, J. Liu, V. Maragou and P. Tsiakaras, KOH-activated multi-walled carbon nanotubes as platinum supports for oxygen reduction reaction, J. Power Sources, 2010, 195(21), 7409–7414 CrossRef CAS.
- S. Tang, G. Sun, J. Qi, S. Sun, J. Guo, Q. Xin and G. M. Haarberg, Review of New Carbon Materials as Catalyst Supports in Direct Alcohol Fuel Cells, Chin. J. Catal., 2010, 31(1), 12–17 CrossRef CAS.
- A. Bharti and G. Cheruvally, Influence of various carbon nano-forms as supports for Pt catalyst on proton exchange membrane fuel cell performance, J. Power Sources, 2017, 360, 196–205 CrossRef CAS.
- S. L. Knupp, W. Li, O. Paschos, T. M. Murray, J. Snyder and P. Haldar, The effect of experimental parameters on the synthesis of carbon nanotube/nanofiber supported platinum by polyol processing techniques, Carbon, 2008, 46(10), 1276–1284 CrossRef CAS.
- B. Escobar, R. Barbosa, M. Miki Yoshida and Y. Verde Gomez, Carbon nanotubes as support of well dispersed platinum nanoparticles via colloidal synthesis, J. Power Sources, 2013, 243, 88–94 CrossRef CAS.
- T. Yamada, H. Yabutani, T. Saito and C. Y. Yang, Temperature dependence of carbon nanofiber resistance, Nanotechnology, 2010, 21(26), 265707 CrossRef PubMed.
- J. Lee, K. Kim, W. I. Park, B. H. Kim, J. H. Park, T. H. Kim, S. Bong, C. H. Kim, G. Chae, M. Jun, Y. Hwang, Y. S. Jung and S. Jeon, Uniform graphene quantum dots patterned from self-assembled silica nanodots, Nano Lett., 2012, 12(12), 6078–6083 CrossRef CAS PubMed.
- C. Zhu, D. Liu, Z. Chen, L. Li and T. You, Superior catalytic activity of Pt/carbon nanohorns nanocomposites toward methanol and formic acid oxidation reactions, J. Colloid Interface Sci., 2018, 511, 77–83 CrossRef CAS PubMed.
- L. Li, L. Hu, J. Li and Z. Wei, Enhanced stability of Pt nanoparticle electrocatalysts for fuel cells, Nano Res., 2015, 8(2), 418–440 CrossRef CAS.
- Y. Sun, Q. Wu and G. Shi, Graphene based new energy materials, Energy Environ. Sci., 2011, 4(4), 1113–1132 RSC.
- G. Saravanan and S. Mohan, Pt nanoparticles embedded on reduced graphite oxide with excellent electrocatalytic properties, Appl. Surf. Sci., 2016, 386, 96–102 CrossRef CAS.
- E. Antolini, Graphene as a new carbon support for low-temperature fuel cell catalysts, Appl. Catal., B, 2012, 123–124, 52–68 CrossRef CAS.
- L. Zhou, Y. Wang, J. Tang, J. Li, S. Wang and Y. Wang, Facile synthesis of holey graphene-supported Pt catalysts for direct methanol electro-oxidation, Microporous Mesoporous Mater., 2017, 247, 116–123 CrossRef CAS.
- A. Ghosh, P. Chandran and S. Ramaprabhu, Palladium-nitrogen coordinated cobalt alloy towards hydrogen oxidation and oxygen reduction reactions with high catalytic activity in renewable energy generations of proton exchange membrane fuel cell, Appl. Energy, 2017, 208, 37–48 CrossRef CAS.
- J. H. Jang, E. Lee, J. Park, G. Kim, S. Hong and Y. U. Kwon, Rational syntheses of core-shell Fex@Pt nanoparticles for the study of electrocatalytic oxygen reduction reaction, Sci. Rep., 2013, 3, 2872 CrossRef PubMed.
- F. Papiya, A. Nandy, S. Mondal and P. P. Kundu, Co/Al2O3-rGO nanocomposite as cathode electrocatalyst for superior oxygen reduction in microbial fuel cell applications: The effect of nanocomposite composition, Electrochim. Acta, 2017, 254, 1–13 CrossRef CAS.
- X. Zhang, P. Lu, C. Zhang, X. Cui, Y. Xu, H. Qu and J. Shi, Towards understanding ORR activity and electron-transfer pathway of M-Nx/C electro-catalyst in acidic media, J. Catal., 2017, 356, 229–236 CrossRef CAS.
- H. Teller and A. Schechter, On the synthesis of RuSe oxygen reduction nano-catalysts for direct methanol fuel cells, J. Solid State Chem., 2017, 21(11), 3103–3111 CAS.
- K. Bhunia, S. Khilari and D. Pradhan, Trimetallic PtAuNi alloy nanoparticles as an efficient electrocatalyst for the methanol electrooxidation reaction, Dalton Trans., 2017, 46(44), 15558–15566 RSC.
- L. Lu, Y. Nie, Y. Wang, G. Wu, L. Li, J. Li, X. Qi and Z. Wei, Preparation of highly dispersed carbon supported AuPt nanoparticles via a capping agent-free route for efficient methanol oxidation, J. Mater. Chem. A, 2018, 6(1), 104–109 RSC.
- L. Huang, X. Zhang, Q. Wang, Y. Han, Y. Fang and S. Dong, Shape-Control of Pt-Ru Nanocrystals: Tuning Surface Structure for Enhanced Electrocatalytic Methanol Oxidation, J. Am. Chem. Soc., 2018, 140(3), 1142–1147 CrossRef CAS PubMed.
- X. Cui, H. Li, G. Yu, M. Yuan, J. Yang, D. Xu, Y. Hou and Z. Dong, Pt coated Co nanoparticles supported on N-doped mesoporous carbon as highly efficient, magnetically recyclable and reusable catalyst for hydrogen generation from ammonia borane, Int. J. Hydrogen Energy, 2017, 42(44), 27055–27065 CrossRef CAS.
- Y. Han, P. Li, J. Liu, S. Wu, Y. Ye, Z. Tian and C. Liang, Strong Fe3+-O(H)-Pt Interfacial Interaction Induced Excellent Stability of Pt/NiFe-LDH/rGO Electrocatalysts, Sci. Rep., 2018, 8(1), 1359 CrossRef PubMed.
- Y. Kim, H. Lee, T. Lim, H.-J. Kim and O. J. Kwon, Non-conventional Pt-Cu alloy/carbon paper electrochemical catalyst formed by electrodeposition using hydrogen bubble as template, J. Power Sources, 2017, 364, 16–22 CrossRef CAS.
- Y. Wu, D. Wang, Z. Niu, P. Chen, G. Zhou and Y. Li, A strategy for designing a concave Pt-Ni alloy through controllable chemical etching, Angew. Chem., 2012, 51(50), 12524–12528 CrossRef CAS PubMed.
- X. Peng, S. Zhao, T. J. Omasta, J. M. Roller and W. E. Mustain, Activity and durability of Pt-Ni nanocage electrocatalysts in proton exchange membrane fuel cells, Appl. Catal., B, 2017, 203, 927–935 CrossRef CAS.
- R. Lin, X. Cai, H. Zeng and Z. Yu, Stability of High-Performance Pt-Based Catalysts for Oxygen Reduction Reactions, Adv. Mater., 2018, 30(17), e1705332 CrossRef PubMed.
- X. Yu, Q. Zhang, Y. Ling, Z. Yang and H. Cheng, Promoted stability and electrocatalytic activity of PtRu electrocatalyst derived from coating by cerium oxide with high oxygen storage capacity, Appl. Surf. Sci., 2018, 455, 815–820 CrossRef CAS.
- L. Bai, Synthesis of PtRu/Ru heterostructure for efficient methanol electrooxidation: the role of extra Ru, Appl. Surf. Sci., 2018, 433, 279–284 CrossRef CAS.
- T. Ishida and M. Haruta, Gold catalysts: towards sustainable chemistry, Angew. Chem., 2007, 46(38), 7154–7156 CrossRef CAS PubMed.
- Y.-Y. Feng, G.-H. Song, Q. Zhang, H.-S. Hu, M.-Y. Feng, J.-Y. Wang and D.-S. Kong, Catalytic performance of non-alloyed bimetallic PtAu electrocatalysts for methanol oxidation reaction, Int. J. Hydrogen Energy, 2017, 42(51), 30109–30118 CrossRef CAS.
- X. Deng, S. Yin, X. Wu, M. Sun, Z. Xie and Q. Huang, Synthesis of PtAu/TiO2 nanowires with carbon skin as highly active and highly stable electrocatalyst for oxygen reduction reaction, Electrochim. Acta, 2018, 283, 987–996 CrossRef CAS.
- S. Esabattina, V. R. Posa, H. Zhanglian, S. k. Godlaveeti, R. R. Nagi Reddy and A. R. Somala, Fabrication of bimetallic PtPd alloy nanospheres supported on rGO sheets for superior methanol electro-oxidation, Int. J. Hydrogen Energy, 2018, 43(8), 4115–4124 CrossRef CAS.
- H. Feng, J. Ma and Z. Hu, Nitrogen-doped carbon nanotubes functionalized by transition metal atoms: a density functional study, J. Mater. Chem., 2010, 20(9), 1702 RSC.
- M. Xu, Y. Zhao, H. Chen, W. Ni, M. Liu, S. Huo, L. Wu, X. Zang, Z. Yang and Y.-M. Yan, Role of Ultrathin Carbon Shell in Enhancing the Performance of PtZn Intermetallic Nanoparticles as an Anode Electrocatalyst for Direct Formic Acid Fuel Cells, ChemElectroChem, 2019, 6(8), 2316–2323 CrossRef.
- S. Kadkhodaei and A. van de Walle, First-principles calculations of thermal properties of the mechanically unstable phases of the PtTi and NiTi shape memory alloys, Acta Mater., 2018, 147, 296–303 CrossRef CAS.
- M. Filip, S. Todorova, M. Shopska, M. Ciobanu, F. Papa, S. Somacescu, C. Munteanu and V. Parvulescu, Effects of Ti loading on activity and redox behavior of metals in PtCeTi/KIT-6 catalysts for CH4 and CO oxidation, Catal. Today, 2018, 306, 138–144 CrossRef CAS.
- X. Jin, C. Zeng, W. Yan, M. Zhao, P. Bobba, H. Shi, P. S. Thapa, B. Subramaniam and R. V. Chaudhari, Lattice distortion induced electronic coupling results in exceptional enhancement in the activity of bimetallic PtMn nanocatalysts, Appl. Catal., A, 2017, 534, 46–57 CrossRef CAS.
- D. Kaewsai and M. Hunsom, Comparative Study of the ORR Activity and Stability of Pt and PtM (M = Ni, Co, Cr, Pd) Supported on Polyaniline/Carbon Nanotubes in a PEM Fuel Cell, Nanomaterials, 2018, 8(5), 299 CrossRef PubMed.
- L. Lu, S. Chen, S. Thota, X. Wang, Y. Wang, S. Zou, J. Fan and J. Zhao, Composition Controllable Synthesis of PtCu Nanodendrites with Efficient Electrocatalytic Activity for Methanol Oxidation Induced by High Index Surface and Electronic Interaction, J. Phys. Chem. C, 2017, 121(36), 19796–19806 CrossRef CAS.
- N. K. Chaudhari, Y. Hong, B. Kim, S.-I. Choi and K. Lee, Pt–Cu based nanocrystals as promising catalysts for various electrocatalytic reactions, J. Mater. Chem. A, 2019, 7(29), 17183–17203 RSC.
- Q. Jia, Z. Zhao, L. Cao, J. Li, S. Ghoshal, V. Davies, E. Stavitski, K. Attenkofer, Z. Liu, M. Li, X. Duan, S. Mukerjee, T. Mueller and Y. Huang, Roles of Mo Surface Dopants in Enhancing the ORR Performance of Octahedral PtNi Nanoparticles, Nano Lett., 2018, 18(1), 798–804 CrossRef CAS PubMed.
- W. Gong, Z. Jiang, R. Wu, Y. Liu, L. Huang, N. Hu, P. Tsiakaras and P. K. Shen, Cross-double dumbbell-like Pt–Ni nanostructures with enhanced catalytic performance toward the reactions of oxygen reduction and methanol oxidation, Appl. Catal., B, 2019, 246, 277–283 CrossRef CAS.
- S. Henning, H. Ishikawa, L. Kuehn, J. Herranz, E. Mueller, A. Eychmueller and T. J. Schmidt, Unsupported Pt-Ni Aerogels with Enhanced High Current Performance and Durability in Fuel Cell Cathodes, Angew. Chem., Int. Ed., 2017, 56(36), 10707–10710 CrossRef CAS PubMed.
- V. Beermann, M. Gocyla, S. Kuhl, E. Padgett, H. Schmies, M. Goerlin, N. Erini, M. Shviro, M. Heggen, R. E. Dunin-Borkowski, D. A. Muller and P. Strasser, Tuning the Electrocatalytic Oxygen Reduction Reaction Activity and Stability of Shape-Controlled Pt-Ni Nanoparticles by Thermal Annealing - Elucidating the Surface Atomic Structural and Compositional Changes, J. Am. Chem. Soc., 2017, 139(46), 16536–16547 CrossRef CAS PubMed.
- S. Wang, P. He, M. He, F. Dong, H. Liu, H. Lei, X. Zhang and S. He, Enhanced Electrocatalytic Activity of Dual Template Based Pt/Cu-zeolite A/Graphene for Methanol Electrooxidation, Chin. J. Chem., 2018, 36(1), 37–41 CrossRef CAS.
- J. Maya-Cornejo, R. Carrera-Cerritos, D. Sebastian, J. Ledesma-Garcia, L. G. Arriaga, A. S. Arico and V. Baglio, PtCu catalyst for the electro-oxidation of ethanol in an alkaline direct alcohol fuel cell, Int. J. Hydrogen Energy, 2017, 42(46), 27919–27928 CrossRef CAS.
- V. Orazi, P. Bechthold, P. V. Jasen, R. Faccio, M. E. Pronsato and E. A. González, DFT study of methanol adsorption on PtCo(111), Appl. Surf. Sci., 2017, 420, 383–389 CrossRef CAS.
- O. Sorsa, H. Romar, U. Lassi and T. Kallio, Co-electrodeposited Mesoporous PtM (M = Co, Ni, Cu) as an Active Catalyst for Oxygen Reduction Reaction in a Polymer Electrolyte Membrane Fuel Cell, Electrochim. Acta, 2017, 230, 49–57 CrossRef CAS.
- N. Becknell, Y. Son, D. Kim, D. Li, Y. Yu, Z. Niu, T. Lei, B. T. Sneed, K. L. More, N. M. Markovic, V. R. Stamenkovic and P. Yang, Control of Architecture in Rhombic Dodecahedral Pt-Ni Nanoframe Electrocatalysts, J. Am. Chem. Soc., 2017, 139(34), 11678–11681 CrossRef CAS PubMed.
- J. Flórez-Montaño, A. Calderón-Cárdenas, W. Lizcano-Valbuena, J. L. Rodríguez and E. Pastor, Ni@Pt nanodisks with low Pt content supported on reduced graphene oxide for methanol electrooxidation in alkaline media, Int. J. Hydrogen Energy, 2016, 41(43), 19799–19809 CrossRef.
- Y.-N. Yue, D. Wu, S. Zeng, M.-P. Yang, H. Wang and J.-X. Lu, Alkaloid-induced asymmetric hydrogenation on bimetallic Pt@Cu cathodes under electrochemical conditions, New J. Chem., 2017, 41(16), 7853–7856 RSC.
- A. X. Yin, X. Q. Min, W. Zhu, W. C. Liu, Y. W. Zhang and C. H. Yan, Pt-Cu and Pt-Pd-Cu concave nanocubes with high-index facets and superior electrocatalytic activity, Chemistry, 2012, 18(3), 777–782 CrossRef CAS PubMed.
- S. Xie, M. Jin, J. Tao, Y. Wang, Z. Xie, Y. Zhu and Y. Xia, Synthesis and characterization of Pd@M(x)Cu(1-x) (M = Au, Pd, and Pt) nanocages with porous walls and a yolk-shell structure through galvanic replacement reactions, Chemistry, 2012, 18(47), 14974–14980 CrossRef CAS PubMed.
- J. Choi, J. Cho, C.-W. Roh, B.-S. Kim, M. S. Choi, H. Jeong, H. C. Ham and H. Lee, Au-doped PtCo/C catalyst preventing Co leaching for proton exchange membrane fuel cells, Appl. Catal., B, 2019, 247, 142–149 CrossRef CAS.
- B. Jiang, Z. Tang, F. Liao, H. Lin, S. Lu, Y. Li and M. Shao, Powerful synergy: efficient Pt–Au–Si nanocomposites as state-of-the-art catalysts for electrochemical hydrogen evolution, J. Mater. Chem. A, 2017, 5(41), 21903–21908 RSC.
- J.-p. Tao, Q.-s. Ji, G.-f. Shao, Z.-p. Li, T.-d. Liu and Y.-h. Wen, Stable structure optimization of Pt-X-Cu (X = Au, Ag, Pd and Rh) trimetallic nanoparticles, J. Alloys Compd., 2017, 716, 240–250 CrossRef CAS.
- M. Li, A. Kowal, K. Sasaki, N. Marinkovic, D. Su, E. Korach, P. Liu and R. R. Adzic, Ethanol oxidation on the ternary Pt–Rh–SnO2/C electrocatalysts with varied Pt:Rh:Sn ratios, Electrochim. Acta, 2010, 55(14), 4331–4338 CrossRef CAS.
- T. S. Almeida, A. R. Van Wassen, R. B. VanDover, A. R. de Andrade and H. D. Abruña, Combinatorial PtSnM (M = Fe, Ni, Ru and Pd) nanoparticle catalyst library toward ethanol electrooxidation, J. Power Sources, 2015, 284, 623–630 CrossRef CAS.
- M. T. Anwar, X. Yan, S. Shen, N. Husnain, F. Zhu, L. Luo and J. Zhang, Enhanced durability of Pt electrocatalyst with tantalum doped titania as catalyst support, Int. J. Hydrogen Energy, 2017, 42(52), 30750–30759 CrossRef CAS.
- M. Dou, M. Hou and F. Wang, Sb-doped SnO2 supported platinum catalyst with high stability for proton exchange membrane fuel cells, Abstr. Pap. Am. Chem. Soc., 2015, 249, 225 Search PubMed.
- X. Wu, W. Zhuang, L. Lu, L. Li, J. Zhu, L. Mu, W. Li, Y. Zhu and X. Lu, Excellent performance of Pt-C/TiO2 for methanol oxidation: Contribution of mesopores and partially coated carbon, Appl. Surf. Sci., 2017, 426, 890–896 CrossRef CAS.
- L. Timperman, A. Lewera, W. Vogel and N. Alonso-Vante, Nanostructured platinum becomes alloyed at oxide-composite substrate, Electrochem. Commun., 2010, 12(12), 1772–1775 CrossRef CAS.
- Y. X. Jin, D. M. Han, W. P. Jia, F. Li, X. Y. Chen, G. B. Huang and D. Zhang, WO3 Modified Graphene Supported Pt Electrocatalysts with Enhanced Performance for Oxygen Reduction Reaction, Int. J. Electrochem. Sci., 2017, 12(7), 6535–6544 CrossRef CAS.
- L. Wang, J. Geng, W. Wang, C. Yuan, L. Kuai and B. Geng, Facile synthesis of Fe/Ni bimetallic oxide solid-solution nanoparticles with superior electrocatalytic activity for oxygen evolution reaction, Nano Res., 2015, 8(12), 3815–3822 CrossRef CAS.
- F. Godínez-Salomón, C. P. Rhodes, K. S. Alcantara, Q. Zhu, S. E. Canton, H. A. Calderon, J. L. Reyes-Rodríguez, M. A. Leyva and O. Solorza-Feria, Tuning the Oxygen Reduction Activity and Stability of Ni(OH)2@Pt/C Catalysts through Controlling Pt Surface Composition, Strain, and Electronic Structure, Electrochim. Acta, 2017, 247, 958–969 CrossRef.
- J.-Y. Park, D.-H. Kwak, K.-B. Ma, S.-B. Han, G. S. Chai, S.-K. Kim, D.-H. Peck, C.-S. Kim, A. Kucernak and K.-W. Park, Enhanced oxygen reduction reaction of Pt deposited Fe/N-doped bimodal porous carbon nanostructure catalysts, J. Catal., 2018, 359, 46–54 CrossRef CAS.
- K. A. Kuttiyiel, K. Sasaki, G.-G. Park, M. B. Vukmirovic, L. Wu, Y. Zhu, J. G. Chen and R. R. Adzic, Janus structured Pt-FeNC nanoparticles as a catalyst for the oxygen reduction reaction, Chem. Commun., 2017, 53(10), 1660–1663 RSC.
- M. Li, Y. Zhu, N. Song, C. Wang and X. Lu, Fabrication of Pt nanoparticles on nitrogen-doped carbon/Ni nanofibers for improved hydrogen evolution activity, J. Colloid Interface Sci., 2017, 514, 199–207 CrossRef PubMed.
- J. Luo, H. Tang, X. Tian, S. Hou, X. Li, L. Du and S. Liao, Highly selective TiN-supported high dispersed Pt catalyst: ultra active towards the hydrogen oxidation and inactive towards the oxygen reduction, ACS Appl. Mater. Interfaces, 2018, 10(4), 3530–3537 CrossRef CAS PubMed.
- H. F. Wang, C. Tang and Q. Zhang, Template growth of nitrogen-doped mesoporous graphene on metal oxides and its use as a metal-free bifunctional electrocatalyst for oxygen reduction and evolution reactions, Catal. Today, 2018, 301, 25–31 CrossRef CAS.
- C. X. Wang, F. Yang, C. Xu, Y. Cao, H. L. Zhong and Y. F. Li, Sulfur-doped porous graphene frameworks as an efficient metal-free electrocatalyst for oxygen reduction reaction, Mater. Lett., 2018, 214, 209–212 CrossRef CAS.
- W. Chen, L. Xu, Y. Tian, H. Li and K. Wang, Boron and nitrogen co-doped graphene aerogels: facile preparation, tunable doping contents and bifunctional oxygen electrocatalysis, Carbon, 2018, 137, 458–466 CrossRef CAS.
- F. Guo, P. Yang, Z. Pan, X.-N. Cao, Z. Xie and X. Wang, Carbon doped BN nanosheets for the oxidative dehydrogenation of ethylbenzene, Angew. Chem., Int. Ed., 2017, 56(28), 8231–8235 CrossRef CAS PubMed.
- M. H. Yeh, Y. A. Leu, W. H. Chiang, Y. S. Li, G. L. Chen, T. J. Li, L. Y. Chang, L. Y. Lin, J. J. Lin and K. C. Ho, Boron-doped carbon nanotubes as metal-free electrocatalyst for dye-sensitized solar cells: heteroatom doping level effect on tri-iodide reduction reaction, J. Power Sources, 2018, 375, 29–36 CrossRef CAS.
- H. You, F. Zhang, Z. Liu and J. Fang, Free-Standing Pt–Au Hollow Nanourchins with Enhanced Activity and Stability for Catalytic Methanol Oxidation, ACS Catal., 2014, 4(9), 2829–2835 CrossRef CAS.
-
Y. K. Chen Chen, Z. Huo,Z. Zhu,W. Huang, H. L. Xin,J. D. Snyder,D. Li,J. A. Herron,M. C. Manos Mavrikakis,K. L. More, Y. Li, N. M. Markovic, G. A. Somorjai,P. Yang and V. R. Stamenkovic, Highly Crystalline Multimetallic Nanoframes with Three-Dimensional Electrocatalytic Surfaces. 2014 Search PubMed.
- A. Jackson, A. Strickler, D. Higgins and T. F. Jaramillo, Engineering Ru@Pt Core-Shell Catalysts for Enhanced Electrochemical Oxygen Reduction Mass Activity and Stability, Nanomaterials, 2018, 8, 38 CrossRef PubMed.
- R. Chang, L. Zheng, C. Wang, D. Yang, G. Zhang and S. Sun, Synthesis of hierarchical platinum-palladium-copper nanodendrites for efficient methanol oxidation, Appl. Catal., B, 2017, 211, 205–211 CrossRef CAS.
- Y. Hu, Q. Shao, P. Wu, H. Zhang and C. Cai, Synthesis of hollow mesoporous Pt–Ni nanosphere for highly active electrocatalysis toward the methanol oxidation reaction, Electrochem. Commun., 2012, 18, 96–99 CrossRef CAS.
- C. Tan, Y. Sun, J. Zheng, D. Wang, Z. Li, H. Zeng, J. Guo, L. Jing and L. Jiang, A self-supporting bimetallic Au@Pt core-shell nanoparticle electrocatalyst for the synergistic enhancement of methanol oxidation, Sci. Rep., 2017, 7(1), 6347 CrossRef PubMed.
- N. K. Chaudhari, J. Joo, B. Kim, B. Ruqia, S. I. Choi and K. Lee, Recent advances in electrocatalysts toward the oxygen reduction reaction: the case of PtNi octahedra, Nanoscale, 2018, 10(43), 20073–20088 RSC.
- R. Tian, S. Shen, F. Zhu, L. Luo, X. Yan, G. Wei and J. Zhang, Icosahedral Pt-Ni Nanocrystalline Electrocatalyst: Growth Mechanism and Oxygen Reduction Activity, ChemSusChem, 2018, 11(6), 1015–1019 CrossRef CAS PubMed.
- C. Li, T. Liu, T. He, B. Ni, Q. Yuan and X. Wang, Composition-driven shape evolution to Cu-rich PtCu octahedral alloy nanocrystals as superior bifunctional catalysts for methanol oxidation and oxygen reduction reaction, Nanoscale, 2018, 10(10), 4670–4674 RSC.
- J. Lim, H. Shin, M. Kim, H. Lee, K.-S. Lee, Y. Kwon, D. Song, S. Oh, H. Kim and E. Cho, Ga-doped Pt-Ni Octahedral Nanoparticles as a Highly Active and Durable Electrocatalyst for Oxygen Reduction Reaction, Nano Lett., 2018, 18(4), 2450–2458 CrossRef CAS PubMed.
- X. Q. Huang, Z. P. Zhao, L. Cao, Y. Chen, E. B. Zhu, Z. Y. Lin, M. F. Li, A. M. Yan, A. Zettl, Y. M. Wang, X. F. Duan, T. Mueller and Y. Huang, High-performance transition metal-doped Pt3Ni octahedra for oxygen reduction reaction, Science, 2015, 348(6240), 1230–1234 CrossRef CAS PubMed.
- N. K. Chaudhari, J. Joo, H.-b. Kwon, B. Kim, H. Y. Kim, S. H. Joo and K. Lee, Nanodendrites of platinum-group metals for electrocatalytic applications, Nano Res., 2018, 11(12), 6111–6140 CrossRef CAS.
- X. Xiong, W. Chen, W. Wang, J. Li and S. Chen, Pt-Pd nanodendrites as oxygen reduction catalyst in polymer-electrolyte-membrane fuel cell, Int. J. Hydrogen Energy, 2017, 42(40), 25234–25243 CrossRef CAS.
- T. Asset, N. Job, Y. Busby, A. Crisci, V. Martin, V. Stergiopoulos, C. Bonnaud, A. Serov, P. Atanassov, R. Chattot, L. Dubau and F. Maillard, Porous Hollow PtNi/C Electrocatalysts: Carbon Support Considerations To Meet Performance and Stability Requirements, ACS Catal., 2018, 8(2), 893–903 CrossRef CAS.
- Y. Hu, P. Wu, H. Zhang and C. Cai, Synthesis of graphene-supported hollow Pt–Ni nanocatalysts for highly active electrocatalysis toward the methanol oxidation reaction, Electrochim. Acta, 2012, 85, 314–321 CrossRef CAS.
- C. Fang, J. Zhao, R. Jiang, J. Wang, G. Zhao and B. Geng, Engineering of Hollow PdPt Nanocrystals via Reduction Kinetic Control for Their Superior Electrocatalytic Performances, ACS Appl. Mater. Interfaces, 2018, 10(35), 29543–29551 CrossRef CAS PubMed.
-
H. B. Aram Oh, D. S. Choi, J. Y. Cheon, B. Kim, H. Kim, S. J. Kwon, S. H. Joo, Y. Jung, and K. Lee, Skeletal Octahedral Nanoframe with Cartesian Coordinates via Geometrically Precise Nanoscale Phase Segregation in a Pt@Ni Core Shell Nanocrystal, 2015 Search PubMed.
- T. Kwon, M. Jun, H. Y. Kim, A. Oh, J. Park, H. Baik, S. H. Joo and K. Lee, Vertex-Reinforced PtCuCo Ternary Nanoframes as Efficient and Stable Electrocatalysts for the Oxygen Reduction Reaction and the Methanol Oxidation Reaction, Adv. Funct. Mater., 2018, 28(13), 1706440 CrossRef.
- A. E. Fetohi, R. S. Amin, R. M. A. Hameed and K. M. El-Khatib, Effect of nickel loading in Ni@Pt/C electrocatalysts on their activity for ethanol oxidation in alkaline medium, Electrochim. Acta, 2017, 242, 187–201 CrossRef CAS.
- I. V. Chepkasov, M. A. Visotin, E. A. Kovaleva, A. M. Manakhov, V. S. Baidyshev and Z. I. Popov, Stability and Electronic Properties of PtPd Nanoparticles via MD and DFT Calculations, J. Phys. Chem. C, 2018, 122(31), 18070–18076 CrossRef CAS.
- L. H. Zhou, Y. X. Wang, J. Tang, J. X. Li, S. L. Wang and Y. Wang, Facile synthesis of holey graphene-supported Pt catalysts for direct methanol electro-oxidation, Microporous Mesoporous Mater., 2017, 247, 116–123 CrossRef CAS.
Footnote |
† These authors contributed equally to this work and should be considered co-first authors. |
|
This journal is © The Royal Society of Chemistry 2020 |