DOI:
10.1039/D0SC03458D
(Edge Article)
Chem. Sci., 2020,
11, 9994-9999
Synthesis and structures of anionic rhenium polyhydride complexes of boron–hydride ligands and their application in catalysis†‡
Received
22nd June 2020
, Accepted 2nd September 2020
First published on 9th September 2020
Abstract
The rhenium complex, [K(DME)(18-c-6)][ReH4(Bpin)(η2-HBpin)(κ2-H2Bpin)] 1, comprising hydride and boron ligands only, has been synthesized by exhaustive deoxygenation of the commercially available perrhenate anion (ReO4−) with pinacol borane (HBpin). The structure of 1 was analysed by X-ray crystallography, NMR spectroscopy, and DFT calculations. While no hydrides were located in the X-ray crystal structure, it revealed a trigonal arrangement of pinacol boron ligands. Variable-temperature NMR spectroscopy supported the presence of seven hydride ligands but further insight was hindered by the fluxionality of both hydride and boron ligands at low temperature. Further evaluation of the structure by Ab Initio Random Structure Searching (AIRSS) identified the presence of hydride, boryl, σ-borane, and dihydroborate ligands. This complex, either isolated or prepared in situ, is a catalyst for the 1,4-hydroboration of N-heteroaromatic substrates under simple operating procedures. It also acts as a reagent for the stoichiometric C–H borylation of toluene, displaying high meta regioselectivity in the borylated products. Reaction of 1 with 9-BBN resulted in HBpin substitution to form the new anionic tetra(dihydroborate) complex [K(DME)(18-c-6)][Re(κ2-H-9-BBN)4] 4 for which the hydride positions were clearly identified by X-ray crystallography. The method used to generate these isolable yet reactive boron–hydride complexes is direct and straightforward and has potential utility for the exploitation of other metal oxo compounds in operationally simple catalytic reactions.
Introduction
The activation of a B–H bond by a transition-metal complex is a key step in catalytic processes such as the borylation of organic molecules1 and the dehydrocoupling of ammonia-borane.2 As such, the synthesis and study of boron–hydride complexes is an area of growing interest.3 A number of studies have shown that hydroboranes can adopt different coordination geometries at a metal centre, a feature which is largely determined by the Lewis acidity of the borane and the Lewis basicity of the metal (Scheme 1A). At one extreme of the bonding continuum are metal boryl hydrides resulting from oxidative addition of the B–H bond to the metal centre, exemplified in Rh,4 Ir,4a,5 Os,6 Ru,4d Nb,7 Ta7a and W complexes.7b,8 At the other extreme, the boron ligand coordinates as a dihydroborate, and which is typically observed for more Lewis-acidic boranes such as 9-borabicyclo[3.3.1]nonane (9-BBN).6,9 Lying between these two extremes are a number of potential σ(B–H) complexes.5b,9g,10
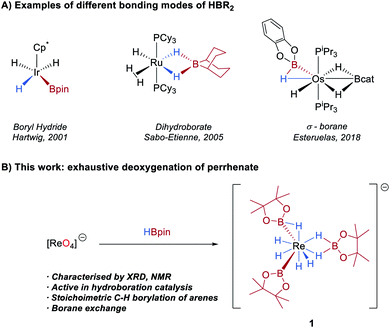 |
| Scheme 1 (A) Selected examples of complexes with boron-centred ligands. (B) This work: synthesis of a rhenium boron–polyhydride anion from HBpin and KReO4. | |
High oxidation-state metal-oxo complexes have been studied in the activation of X–H bonds (X = B, Si, P) for application in catalytic hydrofunctionalisation reactions of unsaturated organic substrates and in deoxydehydration reactions, both of which represent a role-reversal from their traditional use in oxidation chemistry.11 Recently, we reported that [N(hexyl)4][ReO4] can act as a catalyst for the reduction of organic carbonyls and carbon dioxide to the corresponding alcohols by activation of hydrosilanes.12 Similarly, the reduction of sulfoxides by pinacol borane (HBpin) and various Re-oxo catalysts has been reported to proceed by addition of the B–H bond across a Re
O bond to form a boroxy–hydride complex.13
We became interested in exploring the use of the perrhenate anion [ReO4]−, the most commonly traded form of Re,14 as a synthetic precursor for rhenium boron–hydride complexes through the activation of the B–H bonds of common boranes, e.g. HBpin (pin = pinacol). This contrasts with the normal synthetic routes to these types of complexes, which typically involve salt metathesis reactions with an electrophilic boron reagent15 or by reaction of a nucleophilic boryl with a rhenium halide;16 these methods require the use of pre-functionalized and air-sensitive reagents and are limited to low oxidation-state Re centres. Furthermore, rhenium (poly)hydrides are typically synthesised from Re oxides under forcing conditions such as sodium in EtOH17 or by the reaction of a rhenium (oxo)halide with a main group metal hydride such as LiAlH4.18
Herein, we report the operationally simple synthesis of [K(DME)(18-c-6)][ReH4(Bpin)(η2-HBpin)(κ2-H2Bpin)] 1 through the exhaustive deoxygenation of KReO4 by HBpin (Scheme 1B). This complex comprises hydride, boryl, σ-borane, and dihydroborate ligands and is an active catalyst for the 1,4-hydroboration of N-heteroaromatics. A protocol has been developed such that the simple, air-stable alklyammonium complexes [NR4][ReO4] can be exploited as pre-catalysts for hydroboration reactions. We also show that 1 can be further functionalized through borane exchange and is a reagent for stoichiometric and regioselective C–H borylation of arenes.
Results and discussion
Synthesis and structural characterisation of 1
The reaction between KReO4 and HBpin in the presence of 18-crown-6 (18-c-6) forms the new boron polyhydride complex [K(18-c-6)2][ReH7(Bpin)3], O(Bpin)2, and H2 as a result of the complete deoxygenation of KReO4. Recrystallization of the reaction mixture from toluene/DME allows isolation of the product [K(DME)(18-c-6)][ReH4(Bpin)(η2-HBpin)(κ2-H2Bpin)] 1 in 56% yield as colourless needles (Scheme 2). The same anionic moiety is observed by 1H and 11B NMR spectroscopy when perrhenates of alkylammonium countercations, e.g. NBu4+ or N(hexyl)4+ are used instead of potassium (Fig. S8 and S9‡); however, in these former cases the isolation of the deoxygenated product is more challenging due to their enhanced solubility in common organic solvents. The 1H NMR spectrum of 1 in d8-toluene exhibits a singlet hydride resonance at −7.22 ppm integrating to 7 hydrogens which remains sharp on cooling to 173 K. The 11B NMR spectrum shows a single broad resonance at 45.6 ppm which, similarly to the 1H NMR resonances, is unchanged at low temperature. The IR spectrum of 1 shows weak and broad absorptions that are attributable to Re–H stretches between 2000 and 1750 cm−1. Single-crystal X-ray diffraction was carried out on a two-domain aggregate crystal of 1 from which diffraction images were integrated for both domains using two orientation matrices. The solid-state structure shows that two of the Bpin ligands (B1, B1′) are related by a C2-symmetry axis and, along with B2 adopt a pseudo-trigonal arrangement at the Re atom (B1–Re1–B2 = 118.6(2)°, B1–Re1–B1′ = 122.9(5)°) with similar Re1–B1 (2.198(10) Å) and Re1–B2 (2.174(14) Å) distances (Scheme 2, left); these distances compare well with other high oxidation-state boron–hydride complexes.4a–c,5a,7b,19
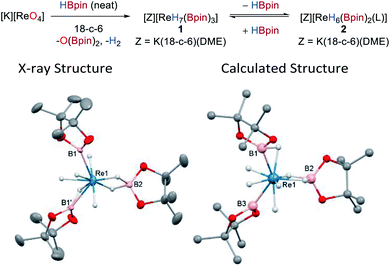 |
| Scheme 2 Synthesis of 1 from [K][ReO4] (L = THF) and the solid-state (left) and calculated (right) structures of 1. For clarity, the cation [K(DME)(18-c-6)]+ and all hydrogen atoms except for the Re hydrides are omitted from the X-ray crystal structure; displacement ellipsoids are drawn at 50% probability. | |
The hydrides in 1 could not be located with confidence from difference maps. As such, possible hydride positions were identified using ab initio random structure searching (AIRSS, see ESI‡ for details) with the positions of the heavier atoms derived from the crystal structure and constraints on prospective hydride positions to ensure neither H2 was formed nor unwanted K–H interactions occurred.20 This approach generated a series of structures with various combinations of hydride, boryl, σ-borane, and hydroborate ligands (Fig. S36‡). Higher energy structures featuring two boryl or three dihydroborate ligands were discounted, leaving a series of closely related, low-energy structures (within 5 kJ mol−1) comprising three different binding modes for the boron ligands, a dihydroborate (B2), a σ-borane (B1), and a boryl (B3), along with four terminal hydrides (Scheme 2, right). This model was then used to place the hydrides in the crystal structure refinement (Scheme 2, left, see ESI‡ for details); while refinement is successful there is only a very minor enhancement to its quality. The geometry optimized and crystal structures show significant differences in the Re1–B2 distances (calcd 2.334 Å cf. X-ray 2.175 Å) and the B1–Re1–B1′/B3 angles (calcd 130.2° cf. X-ray 122.9°) which might arise from disorder in the crystal structure of the anion such that its geometrical parameters are averages (see ESI‡ for details). Also, the calculated structure portrays a formal Re(V) oxidation state, whereas the short Re–B distances in the crystal structure and the 11B NMR data are consistent with more boryl character and suggest a Re(VII) oxidation state. It is therefore evident that rapid hydride/boron ligand rearrangement occurs as seen with other transition metal hydrides,5a,c potentially by a σ-CAM mechanism,21 resulting in a range of energetically similar structures.22 This uncertainty renders the formulation of 1 with respect to the hydride positions as tentative.
Dissolution of 1 in d8-THF results in a different 1H NMR spectrum to that seen in d8-toluene, showing a major signal at −7.74 ppm and a minor signal at −7.32 ppm that slightly increases in intensity over the course of 72 h (Fig. S4 and S5‡). This feature is concomitant with the formation of free HBpin and the growth of a second signal at 41.9 ppm in the 11B NMR spectrum. These spectra suggest that an equivalent of HBpin dissociates upon dissolution of 1 in donor solvents to form the related rhenium boron–polyhydride, [K(DME)(18-c-6)][ReH6(Bpin)2(L)] 2, where L in this instance is THF; decomposition of the two Re species to an insoluble material also occurs over time (see below). This ease of ligand exchange may be important in terms of the catalytic activity of 1.
Stoichiometric reactivity of 1
Complex 1 is indefinitely stable in the solid-state when stored under N2 at −20 °C but decomposes with loss of H2 and HBpin over 3–5 days in ethereal solvents at room temperature to give an insoluble and unidentified dark-brown solid. In contrast, 1 reacts cleanly with aromatic solvents such as C6H6 and toluene over 3 days at room temperature to form the aryl boronic esters 3 and an insoluble, dark-brown rhenium species. Toluene undergoes C–H borylation with high regioselectivity for the meta C–H over the para C–H (81:19) (Scheme 3). To the best of our knowledge, this is the highest reported meta selectivity observed for the stoichiometric or catalytic C–H borylation of toluene.23 This reaction is likely to proceed by a similar mechanism reported for [Ir(Bpin)3(L)2] complexes (where L is a bisphosphine, bipyridine or phenanthroline ligand) which undergo σ-CAM activation of the arene C–H bond.24 This is the first example of a high oxidation-state rhenium complex mediating C–H borylation, contrasting with previous examples that exploit complexes of lower oxidation-states.25
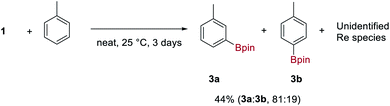 |
| Scheme 3 Stoichiometric borylation of toluene by 1. Regioselectivity and yields were determined by 1H NMR spectroscopy from the crude reaction mixture using trimethoxybenzene as an internal standard and averaged over 3 runs. | |
In addition to C–H functionalization, 1 also undergoes borane exchange. Reaction of 1 with 2 equivalents of 9-BBN dimer (9-BBN = 9-borabicyclo[3.3.1]nonane, HBR2) forms [K(DME)(18-c-6)][Re(κ2-H-9-BBN)4] 4, along with three equivalents of HBpin (Scheme 4). The solid-state structure of 4 reveals dihydroborate ligands in a tetrahedral geometry at the Re atom (average B–Re–B = 109.1°). The hydrides were located in the difference Fourier map and display an average B–H bond length of 1.284(2) Å, with the average Re–B distance of 2.296(2) Å similar to those seen in the optimized structure of 1 and to related dihydroborate complexes.9f,19,26 The IR spectrum of 4 does not display any absorptions attributable to Re–H stretches which further supports a borohydride bonding motif. Complex 4 is insoluble in aromatic and alkane solvents. On dissolution in d8-THF, the 1H NMR spectrum of 4 shows two hydride signals at −9.02 ppm (s, br) and −9.80 ppm (s) which have a combined integration of 8 protons. As free 9-BBN monomer is seen in the 11B NMR spectrum, it is evident that substitution of 9-BBN by THF occurs to form a mixture of 4 and presumably the THF-adduct [K(DME)(18-c-6)][ReH(κ2-H-9-BBN)3(THF)] 5. Upon addition of 0.5 equivalents of 9-BBN dimer to a solution of crystalline 4, the ratio of 4 to 5 increases from 61
:
39 to 97
:
3 (Fig. S12‡). A coinciding decrease occurs in the signal at 52.7 ppm and an increase in the signal at 29.4 ppm in the 11B NMR spectrum (Fig. S13‡). In contrast, addition of 2 equivalents of DABCO to a solution of 4 results in 5 as the only hydridic species in the 1H NMR spectrum (Fig. S14‡), with the only signals in the 11B NMR spectrum at 52.7 ppm and the DABCO·9-BBN adduct at 2.38 ppm (Fig. S15‡). A minor unknown species is also formed upon dissolution of 4 in d8-THF that displays a sharp singlet at 57.4 ppm in the 11B NMR spectrum that diminishes upon addition of either DABCO or 9-BBN.
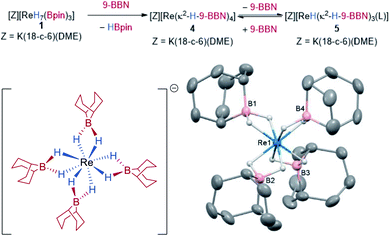 |
| Scheme 4 Synthesis of 4 from 1 by borane exchange and the solid-state structure of 4. For clarity, the cation [K(DME)(18-c-6)]+ and all hydrogen atoms except for the Re hydrides are omitted; displacement ellipsoids are drawn at 50% probability. | |
Catalytic hydroboration of N-heteroaromatics
The simple synthesis of 1 by exhaustive deoxygenation of [ReO4]− and the presence of both hydride and boron-based ligands should favor its exploitation in catalytic hydroboration chemistry. Indeed, using [N(hexyl)4][ReO4] (2.5 mol%) as a bench-stable pre-catalyst with HBpin (1.5 or 2.0 equiv.) results in the catalytic hydroboration of pyridine to give the 1,4-dihydropyridine (1,4-DHP) product in >95% yield after 16 h at room temperature (Scheme 5).27 This represents one of the first examples of the hydroboration of N-heteroaromatics using a high oxidation-state, transition metal catalyst.28 The hydroboration of benzannulated substrates proceeds in good yield and regioselectivity, with quinoline, isoquinoline and acridine 6b–6d forming the hydroborated products. 3-Methylpyridine 6e, 3-phenylpyridine 6f and 2,6-lutidine 6g react in good yields and regioselectivities. N-Methylbenzimidazole 6h is hydroborated to the corresponding 1,4-DHP product in high yield. Substrates bearing an electron-withdrawing group such as methyl-3-pyridinecarboxylate 6i are tolerated in the reaction with no observable reduction of the ester group and relatively short reaction times. 3-Methoxypyridine 6j is reduced with poor regioselectivity and requires an extended reaction time. Only 1,2-, 1,4- and 1,6-DHP regioisomers are observed in this catalytic system. Interestingly, in the presence of 1 equivalent of HBpin, 3-acetylpyridine 6k is selectively reduced to the corresponding boroxy ether with no formation of the DHP product observed.
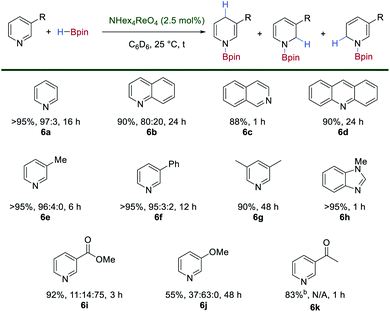 |
| Scheme 5 Catalytic hydroboration of N-heteroaromatics using [N(hexyl)4][ReO4] as a pre-catalyst and HBpin. For 6k reduction is observed exclusively at the carbonyl when 1 equivalent of HBpin is used. | |
The reactions of para- and ortho-substituted pyridines are very slow under these conditions with only trace amounts of DHPs observed after extended reaction times. 3-Nitropyridine gives a complex mixture, presumably due to competitive reduction of the nitro group. Similarly, complex mixtures of products are observed for substrates bearing alkynyl, alkenyl and Boc-protected amines. No conversion of iodo-, bromo- and chloro-substituted pyridines to DHPs is seen under these reaction conditions.
The isolated complex 1 is also a competent, albeit sluggish, catalyst for this reaction giving comparable yields and regioselectivity to the catalyst generated in situ, a feature which may be due to its reduced solubility. However, when 1 was dissolved in d5-pyridine both 1 and 2 are observed in the 1H and 11B NMR spectra. When monitored over 96 h, the gradual consumption of 1 and 2 is observed along with the formation of multiple new hydride signals in the 1H NMR spectrum which eventually resolve into a singlet at δ −2.63 ppm (Fig. S6‡). In the 11B NMR spectrum a signal at δ 23.9 ppm is observed to increase in intensity over time and is indicative of N–Bpin bond formation (Fig. S7‡). These observations suggest that 1 serves as a reservoir for the more reactive, bis(boron) hydride complex 2 through substitution of HBpin by the pyridine substrate. Subsequent boration is likely to proceed by an inner-sphere mechanism of hydride transfer to the activated pyridine substrate, although the 1,4-selectivity seen is unusual for a transition-metal hydride catalyst.29
Conclusions
An operationally simple procedure for the synthesis of the reactive rhenium boron–polyhydride anion 1 from bench-stable and commercially available starting materials has been developed. This is a rare of example of a high oxidation-state, transition metal complex of a boron–hydride ligand. Anion 1 exhibits diverse reactivity including stoichiometric C–H borylation, borane ligand substitution, and the catalytic hydroboration of N-heteroaromatics. The methods described could provide a general route to a range of reactive boron–hydride complexes from other, commercially available, metal oxo anions such as RuO4−, WO42− and MoO42−. These complexes may have wider implications in the catalytic hydroboration of unsaturated organic molecules and in the catalytic C–H borylation of arenes in the presence of stabilising ligands. These aspects are currently under further investigation in our laboratory.
Conflicts of interest
There are no conflicts to declare.
Acknowledgements
We thank the University of Edinburgh, the EPSRC (UK), and the EPSRC CRITICAT Centre for Doctoral Training (PhD studentship to L. J. D.; Grant EP/L016419/1) for financial support. S. P. T. thanks The Royal Society for a University Research Fellowship. We thank the University of Edinburgh's ECDF and the EaStCHEM Research computing facility for hardware and software provision.
Notes and references
- Representative reviews on C–H borylation and C–B bond functionalization:
(a) L. Dang, Z. Lin and T. B. Marder, Chem. Commun., 2009, 3987–3995 RSC
;
(b) J. W. B. Fyfe and A. J. B. Watson, Chem, 2017, 3, 31–55 CrossRef CAS
;
(c) I. A. I. Mkhalid, J. H. Barnard, T. B. Marder, J. M. Murphy and J. F. Hartwig, Chem. Rev., 2010, 110, 890–931 CrossRef CAS
.
- Representative reviews on dehydropolymerization of amine-borane adducts:
(a) D. Han, F. Anke, M. Trose and T. Beweries, Coord. Chem. Rev., 2019, 380, 260–286 CrossRef CAS
;
(b) C. W. Hamilton, R. T. Baker, A. Staubitz and I. Manners, Chem. Soc. Rev., 2009, 38, 279–293 RSC
;
(c) A. Rossin and M. Peruzzini, Chem. Rev., 2016, 116, 8848–8872 CrossRef CAS
;
(d) A. Staubitz, A. P. M. Robertson, M. E. Sloan and I. Manners, Chem. Rev., 2010, 110, 4023–4078 CrossRef CAS
.
- Representative reviews on transition metal complexes with boron-centred ligands:
(a) G. J. Irvine, M. J. G. Lesley, T. B. Marder, N. C. Norman, C. R. Rice, E. G. Robins, W. R. Roper, G. R. Whittell and L. J. Wright, Chem. Rev., 1998, 98, 2685–2722 CrossRef CAS
;
(b) H. Braunschweig, R. D. Dewhurst and A. Schneider, Chem. Rev., 2010, 110, 3924–3957 CrossRef CAS
;
(c) Q. Zhao, R. D. Dewhurst, H. Braunschweig and X. Chen, Angew. Chem., Int. Ed., 2019, 58, 3268–3278 CrossRef CAS
;
(d) H. Braunschweig, C. Kollann and D. Rais, Angew. Chem., Int. Ed., 2006, 45, 5254–5274 CrossRef CAS
.
-
(a) N. Zhang, R. S. Sherbo, G. S. Bindra, D. Zhu and P. H. M. Budzelaar, Organometallics, 2017, 36, 4123–4135 CrossRef CAS
;
(b) K. S. Cook, C. D. Incarvito, C. E. Webster, Y. Fan, M. B. Hall and J. F. Hartwig, Angew. Chem., Int. Ed., 2004, 43, 5474–5477 CrossRef CAS
;
(c) J. F. Hartwig, K. S. Cook, M. Hapke, C. D. Incarvito, Y. Fan, C. E. Webster and M. B. Hall, J. Am. Chem. Soc., 2005, 127, 2538–2552 CrossRef CAS
;
(d) P. L. Callaghan, R. Fernández-Pacheco, N. Jasim, S. Lachaize, T. B. Marder, R. N. Perutz, E. Rivalta and S. Sabo-Etienne, Chem. Commun., 2004, 242–243 RSC
.
-
(a) K. Kawamura and J. F. Hartwig, J. Am. Chem. Soc., 2001, 123, 8422–8423 CrossRef CAS
;
(b) L. P. Press, A. J. Kosanovich, B. J. McCulloch and O. V. Ozerov, J. Am. Chem. Soc., 2016, 138, 9487–9497 CrossRef CAS
;
(c) B. Ghaffari, B. A. Vanchura II, G. A. Chotana, R. J. Staples, D. Holmes, R. E. Maleczka Jr and M. R. Smith III, Organometallics, 2015, 34, 4732–4740 CrossRef CAS
.
- M. A. Esteruelas, A. M. López, M. Mora and E. Oñate, Organometallics, 2015, 34, 941–946 CrossRef CAS
.
-
(a) D. R. Lantero, S. L. Miller, J.-Y. Cho, D. L. Ward and M. R. Smith, Organometallics, 1999, 18, 235–247 CrossRef CAS
;
(b) J. F. Hartwig and S. R. De Gala, J. Am. Chem. Soc., 1994, 116, 3661–3662 CrossRef CAS
.
- J. F. Hartwig and X. He, Organometallics, 1996, 15, 5350–5358 CrossRef CAS
.
-
(a) A. Antiñolo, F. Carrillo-Hermosilla, J. Fernández-Baeza, S. García-Yuste, A. Otero, A. M. Rodríguez, J. Sánchez-Prada, E. Villaseñor, R. Gelabert, M. Moreno, J. M. Lluch and A. Lledós, Organometallics, 2000, 19, 3654–3663 CrossRef
;
(b) X. Chen, S. Liu, C. E. Plečnik, F.-C. Liu, G. Fraenkel and S. G. Shore, Organometallics, 2003, 22, 275–283 CrossRef CAS
;
(c) F.-C. Liu, C. E. Plečnik, S. Liu, J. Liu, E. A. Meyers and S. G. Shore, J. Organomet. Chem., 2001, 627, 109–120 CrossRef CAS
;
(d) C. E. Plečnik, F.-C. Liu, S. Liu, J. Liu, E. A. Meyers and S. G. Shore, Organometallics, 2001, 20, 3599–3606 CrossRef
;
(e) X. Chen, S. Lim, C. E. Plečnik, S. Liu, B. Du, E. A. Meyers and S. G. Shore, Inorg. Chem., 2004, 43, 692 CrossRef CAS
;
(f) E. Ding, B. Du, E. A. Meyers, S. G. Shore, M. Yousufuddin, R. Bau and G. J. McIntyre, Inorg. Chem., 2005, 44, 2459–2464 CrossRef CAS
;
(g) S. Lachaize, K. Essalah, V. Montiel-Palma, L. Vendier, B. Chaudret, J.-C. Barthelat and S. Sabo-Etienne, Organometallics, 2005, 24, 2935–2943 CrossRef CAS
;
(h) J. Knizek and H. Nöth, Eur. J. Inorg. Chem., 2011, 12, 1888–1900 CrossRef
;
(i) S. Chakraborty, J. Zhang, Y. J. Patel, J. A. Krause and H. Guan, Inorg. Chem., 2013, 52, 37–47 CrossRef CAS
.
-
(a) G. Alcaraz and S. Sabo-Etienne, Coord. Chem. Rev., 2008, 252, 2395–2409 CrossRef CAS
;
(b) G. Alcaraz, M. Grellier and S. Sabo-Etienne, Acc. Chem. Res., 2009, 42, 1640–1649 CrossRef CAS
;
(c) V. Montiel-Palma, M. Lumbierres, B. Donnadieu, S. Sabo-Etienne and B. Chaudret, J. Am. Chem. Soc., 2002, 124, 5624–5625 CrossRef CAS
;
(d) T. J. Hebden, M. C. Denney, V. Pons, P. M. B. Piccoli, T. F. Koetzle, A. J. Schultz, W. Kaminsky, K. I. Goldberg and D. M. Heinekey, J. Am. Chem. Soc., 2008, 130, 10812–10820 CrossRef CAS
;
(e) M. A. Esteruelas, F. J. Fernández-Alvarez, A. M. López, M. Mora and E. Oñate, J. Am. Chem. Soc., 2010, 132, 5600–5601 CrossRef CAS
;
(f) M. A. Esteruelas, I. Fernández, C. García-Yebra, J. Martín and E. Oñate, Organometallics, 2017, 36, 2298–2307 CrossRef CAS
;
(g) J. C. Babón, M. A. Esteruelas, I. Fernández, A. M. López and E. Oñate, Inorg. Chem., 2018, 57, 4482–4491 CrossRef
.
-
(a) S. C. A. Sousa, I. Cabrita and A. C. Fernandes, Chem. Soc. Rev., 2012, 41, 5641–5653 RSC
;
(b) L. J. Donnelly, S. P. Thomas and J. B. Love, Chem.–Asian
J., 2019, 14, 3782–3790 CrossRef CAS
;
(c) D. S. Morris, K. van Rees, M. Curcio, M. Cokoja, F. E. Kühn, F. Duarte and J. B. Love, Catal. Sci. Technol., 2017, 7, 5644–5649 RSC
.
- D. S. Morris, C. Weetman, J. T. C. Wennmacher, M. Cokoja, M. Drees, F. E. Kühn and J. B. Love, Catal. Sci. Technol., 2017, 7, 2838–2845 RSC
.
-
(a) A. C. Fernandes, J. A. Fernandes, F. A. Almeida Paz and C. C. Romao, Dalton Trans., 2008, 6686–6688 RSC
;
(b) A. C. Fernandes, J. A. Fernandes, C. C. Romão, L. F. Veiros and M. J. Calhorda, Organometallics, 2010, 29, 5517–5525 CrossRef CAS
.
-
United States Geological Survey, https://pubs.er.usgs.gov/publication/pp1802P, May 2020.
- K. M. Waltz, C. N. Muhoro and J. F. Hartwig, Organometallics, 1999, 18, 3383–3393 CrossRef CAS
.
- R. Frank, J. Howell, R. Tirfoin, D. Dange, C. Jones, D. M. P. Mingos and S. Aldridge, J. Am. Chem. Soc., 2014, 136, 15730–15741 CrossRef CAS
.
- K. Abdur-Rashid, A. J. Lough and R. H. Morris, Can. J. Chem., 2001, 79, 964–976 CrossRef CAS
.
- D. Baudry and M. Ephritikhine, J. Chem. Soc., Chem. Commun., 1980, 249–250 RSC
.
-
(a) X.-Y. Liu, S. Bouherour, H. Jacobsen, H. W. Schmalle and H. Berke, Inorg. Chim. Acta, 2002, 330, 250–267 CrossRef CAS
;
(b) G. Jia, A. J. Lough and R. H. Morris, J. Organomet. Chem., 1993, 461, 147–156 CrossRef CAS
.
-
(a) C. J. Pickard and R. J. Needs, J. Phys.: Condens. Matter, 2011, 23, 053201 CrossRef
;
(b) S. J. Clark, M. D. Segall, C. J. Pickard, P. J. Hasnip, M. J. Probert, K. Refson and M. C. Payne, Z. Kristallogr., 2005, 220, 567–570 CAS
.
- R. N. Perutz and S. Sabo-Etienne, Angew. Chem., Int. Ed., 2007, 46, 2578–2592 CrossRef CAS
.
- N. Seiffert, T. Kendrick, D. Tiana and C. A. Morrison, Dalton Trans., 2015, 44, 4259–4270 RSC
.
- T. Kato, S. Kuriyama, K. Nakajima and Y. Nishibayashi, Chem.–Asian J., 2019, 14, 2097–2101 CrossRef CAS
.
-
(a) T. M. Boller, J. M. Murphy, M. Hapke, T. Ishiyama, N. Miyaura and J. F. Hartwig, J. Am. Chem. Soc., 2005, 127, 14263–14278 CrossRef CAS
;
(b) H. Tamura, H. Yamazaki, H. Sato and S. Sakaki, J. Am. Chem. Soc., 2003, 125, 16114–16126 CrossRef CAS
.
-
(a) H. Chen and J. F. Hartwig, Angew. Chem., Int. Ed., 1999, 38, 3391–3393 CrossRef CAS
;
(b) M. Murai, T. Omura, Y. Kuninobu and K. Takai, Chem. Commun., 2015, 51, 4583–4586 RSC
.
- E. Ding, B. Du, F.-C. Liu, S. Liu, E. A. Meyers and S. G. Shore, Inorg. Chem., 2005, 44, 4871–4878 CrossRef CAS
.
-
(a) S. Park and S. Chang, Angew. Chem., Int. Ed., 2017, 56, 7720–7738 CrossRef CAS
;
(b) B. Rao, C. Chang Chong and R. Kinjo, J. Am. Chem. Soc., 2018, 140, 652–656 CrossRef CAS
;
(c) T. Lundrigan, C. H. Tien and K. N. Robertson, Chem. Commun., 2020, 56, 8027–8030 RSC
;
(d) S. Schnitzler, T. P. Spaniol and J. Okuda, Inorg. Chem., 2016, 55, 12997–13006 CrossRef CAS
.
- Recently, it was shown that [(PPh3)2Re(O)2I] can act as a catalyst for the hydroboration of quinoline, but this reaction proceeds with poor chemo- and regioselectivity: R. Arevalo, C. M. Vogels, G. A. MacNeil, L. Riera, J. Perez and S. A. Westcott, Dalton Trans., 2017, 46, 7750–7777 RSC
.
- S. Park, ChemCatChem, 2020, 12, 1–17 CrossRef
.
Footnotes |
† Dedicated to Prof. Alan Welch on the occasion of his retirement from Heriot-Watt University. |
‡ Electronic supplementary information (ESI) available. CCDC 1979241 and 1979242. For ESI and crystallographic data in CIF or other electronic format see DOI: 10.1039/d0sc03458d |
|
This journal is © The Royal Society of Chemistry 2020 |