DOI:
10.1039/D0SC03223A
(Edge Article)
Chem. Sci., 2020,
11, 8145-8150
An atropisomeric M2L4 cage mixture displaying guest-induced convergence and strong guest emission in water†
Received
10th June 2020
, Accepted 17th July 2020
First published on 20th July 2020
Abstract
Introduction of atropisomeric axes into a bent bispyridine ligand leads to the quantitative formation of a complex mixture of atropisomeric M2L4 cages upon treatment with metal ions. Whereas the isomer ratio of the obtained cage mixture, consisting of up to 42 isomers, is insensitive to temperature and solvent, the quantitative convergence from the mixture to a single isomer is accomplished upon encapsulation of a large spherical guest, namely fullerene C60. The observed isomerization with other guests depends largely on their size and shape (e.g., <10 and 82% convergence with planar triphenylene and bowl-shaped corannulene guests, respectively). Besides the unusual guest-induced convergence, the present cage mixture displays the strongest guest emission (ΦF = 68%) among previously reported MnLm cages and capsules, upon encapsulation of a BODIPY dye in water.
Introduction
Biological assemblies such as viral capsids and ferritins display well-organized, spherical nanoarchitectures yet consist of unsymmetrical protein subunits bearing flexible moieties before assembly (Fig. 1a).1 On the other hand, synthetic chemists are apt to design highly symmetrical subunits with sufficient conformational rigidity to precisely construct spherical nanostructures through hydrogen bonding2 and coordination-driven self-assemblies,3e.g., a spherical M2L4 cage (Fig. 1b).4 The cavity size and properties of such artificial assemblies are also highly controllable by the use of the well-designed components.5 In contrast, desymmetrized, synthetic subunits with one or more isomerizable moieties give rise to a complex mixture of product isomers via self-assembly (e.g., Fig. 1c). So far, no rational method has been reported to converge such complex, artificial mixtures (so-called dynamic combinatorial libraries)6 into a single, three-dimensional isomer.
 |
| Fig. 1 Schematic representation of the self-assemblies of (a) a biological nanostructure, (b) an M2L4 cage from symmetrical ligands and metal ions, and (c) a complex mixture of M2L4 cage isomers from desymmetrized ligands and metal ions studied herein. | |
Herein we report the design and synthesis of bent bispyridine ligand 1 with two atropisomeric axes (Fig. 2a). Treatment of the isomerizable and desymmetrized ligands with metal ions leads to the quantitative formation of a complex mixture of atropisomeric M2L4 cages 2 (Fig. 2b). Although the isomer ratio of the resultant cages is insensitive to temperature and solvent, the selective and quantitative convergences from the complex mixture to a single isomer are demonstrated upon encapsulation of spherical corannulene dimer and fullerene C60, respectively. In addition, the present cage mixture displays the strongest guest emission (ΦF = 68%), as compared to previously reported MnLm cages and capsules,7 upon encapsulation of a fluorescent BODIPY dye.
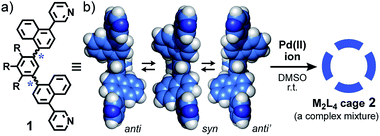 |
| Fig. 2 Schematic representation of (a) bispyridine ligand 1 (R = –OCH2CH2OCH3) and, (b) the optimized structures of the atropisomers in equilibrium (DFT calculation, B3LYP/6-31* level, R = –OCH3) and the formation of M2L4 cage 2 as a complex isomeric mixture. | |
As the simplest model, we chose a highly symmetrical M2L4 structure to examine its desymmetrization and convergence. It has been established that bent bispyridine ligands with a virtual C2v symmetry generate M2L4 cages or capsules as a single isomer upon complexation with metal ions (Fig. 1b).4 Their host abilities have been widely investigated by many groups so far.8,9 Thus we introduced atropisomeric axes10 into the ligand and designed 1,4-naphthylene-embedded, bent ligand 1 (Fig. 2a). The new bispyridine ligand adopts three atropisomers owing to hindered rotation around the phenyl–naphthyl bonds so that the corresponding atropisomeric M2L4 cage, possessing a spherical hydrophobic cavity (∼1 nm in diameter), is anticipated to be present as an equilibrium mixture of up to 42 isomers (Fig. 3).
 |
| Fig. 3 Schematic representation of the possible 42 isomers of M2L4 cage 2. These isomers are in equilibrium in solution. | |
Results and discussion
Formation of a complex mixture of M2L4 cages
First we observed the quantitative formation of a complex mixture of M2L4 cage 2 isomers by mixing atropisomeric ligand 1, PdCl2(DMSO)2, and AgNO3 (in a 2
:
1
:
2 ratio) in DMSO-d6 for 1 h at room temperature (Fig. 2).11,12a As expected, the 1H NMR spectrum of the product mixture showed complicated and broadened, aromatic signals in the range of 9.7 to 6.6 ppm, in contrast to the simple and sharp signals observed for free ligand 1 (Fig. 4a and b). The 1H DOSY NMR spectrum revealed that these proton signals have the same diffusion constant (D = 1.2 × 10−10 m2 s−1; Fig. 4c),12b indicating the existence of Pd(II)-linked cage 2 as an isomeric mixture in equilibrium. The exclusive formation of M2L4 assemblies 2 was unambiguously confirmed by ESI-TOF MS analysis: prominent peaks were observed at m/z 759.9, 1033.9, and 1582.3, assignable to the [2 – n·NO3−]n+ species (n = 4, 3, and 2, respectively; Fig. 4d). In a manner similar to 2, Pt(II)-analogue 2′ was formed quantitatively and its M2L4 structure was confirmed by NMR and MS analyses.11 The existence of various isomers of 2′ was also established by the complicated and broadened 1H NMR signals (Fig. S23†).
 |
| Fig. 4
1H NMR spectra (500 MHz, DMSO-d6, r.t.) of (a) ligand 1 and (b) a complex mixture of cage isomers 2. (c) 1H DOSY NMR (500 MHz, DMSO-d6, 298 K) and (d) ESI-TOF MS (DMSO, r.t.) spectra of isomers 2. Optimized structures of 2: (e) an all-syn isomer and (f and g) an all-anti isomer (substituents and counterions are omitted for clarity). | |
The theoretical calculation of cage 2 indicated that differences in energy are relatively small among representative isomers (ΔE < 1.8 kcal mol−1; e.g., Fig. 4e, f and S22†),11 supporting the complex signals observed in the 1H NMR spectrum (Fig. 4b). The optimized structure of 2 (e.g., an all-anti isomer, Fig. 4g) clarified that the spherical cavity (∼580 Å3) is surrounded by eight naphthalene panels and the cavity diameter is 1.3 nm. Although the cavity shape and size are almost identical to those of anthracene-based M2L4 capsule 3 reported previously (Fig. S1†),9,11 the bent polyaromatic ligands of 2 are proximate to each other yet unstacked.
Guest-induced convergence to a single isomer
Whereas the isomer ratio of cage 2 remained intact even upon changing the solvent (e.g., CD3CN, CD3OD, and D2O) and temperature (e.g., −10 to 110 °C), guest encapsulation allowed the complex mixture to convert into a single isomer in water. When a simple planar and hydrophobic guest, triphenylene (Tp; excess), was mixed with a D2O solution of 2 (0.6 mM) at room temperature for 1 h (Fig. 5a), the 1H NMR signals of 2 changed from completely broad13 to slightly sharp (Fig. 5b and c), due to the quantitative incorporation of the guests (2 equiv.) into the host cavity through the hydrophobic effect.14 The NMR signals were unchanged even at elevated temperature (e.g., 80 °C). The ESI-TOF MS analysis of the product clearly supported the formation of 1
:
2 host–guest complex 2·(Tp)2 (Fig. S27c†). This result indicated the possibility of wide-ranging host abilities of 2 in water and selective isomerization of 2 through an appropriate guest stimulus.15
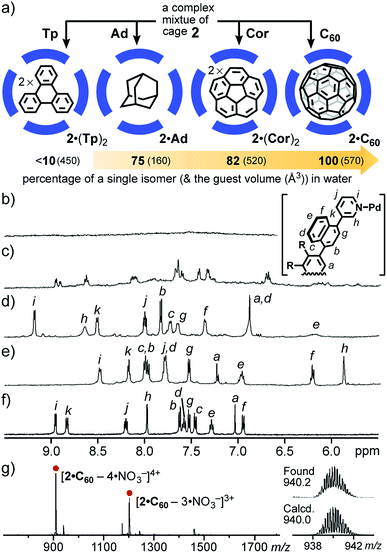 |
| Fig. 5 (a) Schematic representation of the guest-induced convergence of a complex mixture of 2. 1H NMR spectra (500 MHz, D2O, r.t.) of (b) cage 2, (c) 2·(Tp)2, (d) 2·Ad, (e) 2·(Cor)2, and (f) 2·C60. (g) ESI-TOF MS spectrum (H2O) of 2·C60 and the expansion and simulation of the [2·C60 – 4·NO3−]4+ signals. | |
Next, the treatment of small spherical adamantane (Ad) and bowl-shaped corannulene (Cor) with cage mixture 2 gave rise to host–guest complexes 2·Ad and 2·(Cor)2 in a quantitative manner, respectively, under the same aqueous conditions (Fig. 5a).15 Interestingly, the resultant 1H NMR spectra revealed further conversion from the complex mixtures to a single isomer upon binding of the sterically demanding guests, whose shapes are similar to the spherical cavity of 2. The percentages of the generated, major host isomer accommodating Ad and (Cor)2 guests were estimated to be 75 and 82, respectively (Fig. 5d and e), on the basis of the reference proton signal of the methoxy groups of 2 around 2.96–2.83 ppm. The aliphatic proton signals of bound Ad appeared around −0.7 ppm, due to the aromatic shielding effect of 2 (Fig. S28a†). The eleven aromatic signals were clearly observed in the 1H NMR spectrum of 2·(Cor)2 (Fig. 5e). Noteworthy, a similar 1H NMR spectrum was obtained from a mixture of cage 2′, which is an analogue of 2 with Pt(II) ions, and excess Cor in D2O at room temperature for 7 h (Fig. S30†).11 The finding revealed that the naphthalene panels embedded in the spherical framework of 2 can flip even at ambient temperature, because the bonds between the Pt(II) hinges and the pyridyl groups are fixed under these conditions.
The quantitative conversion of the mixture into a single isomer was accomplished upon incorporation of spherical fullerene C60 (C60) into cage 2. Mixing of cage 2 with excess C60 in D2O at 100 °C for 24 h yielded 1
:
1 host–guest complex 2·C60 exclusively. Intense ESI-TOF MS peaks at m/z = 940.2 and 1274.2 were assignable to [2·C60 – n·NO3−]n+ species (n = 4 and 3; Fig. 5g). Notably, the 1H NMR spectrum of 2·C60 exhibited only eleven signals in the aromatic region, indicating the presence of a single isomer (∼100% selectivity; Fig. 5f). In the 13C NMR spectrum of 2·C60, a single signal for the C60 guest was observed at 140.1 ppm (Fig. S32†), in a manner similar to that of the previous C60-incorporated M2L4 capsule (141.2 ppm).9a The broadened, new UV-visible absorption bands derived from the guest were also found around 400–730 nm (Fig. S36†).16 In short, these results revealed that the “atropisomeric” dynamic combinatorial library6 of cage 2 can be strictly controlled by its size (volume) and shape complementary guest molecules.
Crystallographic analysis of the single isomer
The direction of the eight naphthalene panels on 2·C60 was eventually determined by X-ray crystallographic and theoretical analyses.11,17,18 As we expected, the crystal structure showed that one molecule of C60 is fully captured by the cage-shaped M2L4 framework of 2 (Fig. 6a). Remarkably, all the bent bispyridine ligands adopted a syn conformation and aligned roundly in the same direction (Fig. 6b), most probably to avoid the steric repulsion between the naphthalene panels of the adjacent ligands. The π-stacking interactions between C60 and the naphthalene panels were observed at eight positions (d = 3.2–3.4 Å in interplanar distance; Fig. S38†). The theoretical calculations of 2·C60 (R = –H) on the basis of the present crystal structure indicated that the energy of the all-syn isomer (Fig. 6a) is 10.6 kcal mol−1 lower than that of an all-anti isomer (Fig. 6c) in the gas phase. The cage framework possesses four small openings (approximately 4 × 5 Å2) between the polyaromatic ligands, in contrast to previous M2L4 capsule 3 with an anthracene-based, closed shell.9 Preliminary host–guest studies showed the selective binding of one molecule of functionalized fullerenes (e.g., diethyl malonate-derivatized C60) by the present cage in water (Fig. S40†).11
 |
| Fig. 6 X-ray crystal structure of 2·C60 (all-syn isomer): (a) space-filling representation for C60 and (b) space-filling representation for the naphthalene panels (the substituents are replaced by H atoms for clarity). (c) Optimized structure of 2·C60 (all-anti isomer, R = –H). | |
Strong emission of an encapsulated BODIPY derivative
Finally, we encountered that naphthalene-based Pt(II)-cage 2′ displays very strong guest emission upon encapsulation of a BODIPY dye in water.19 After stirring a mixture of cage 2′ and water-insoluble, pentamethyl boron-dipyrromethene PMB (9 equiv.) in D2O at 80 °C for 1.5 h (Fig. 7a), the formation of 1
:
1 host–guest complex 2′·PMB was evidenced by NMR, MS, and UV-visible analyses. The obtained, broad 1H NMR signals indicated the existence of a complex isomeric mixture including the guest dye (Fig. S41a†). The 1
:
1 host–guest ratio of the product was confirmed by the ESI-TOF MS spectrum, which shows clear MS peaks for [2′·PMB – n·NO3−]n+ species (n = 4, 3; Fig. S41b†). The UV-visible spectrum of the pale yellow solution of 2′·PMB in water showed new absorption bands in the range of 410–550 nm, derived from encapsulated PMB (Fig. 7b). The observed bands were slightly red-shifted (Δλ = 10 nm) with respect to those of free PMB in CH3CN and their intensity indicated the encapsulation efficiency being ∼25%. The optimized structure of 2′·PMB (R = –H) suggested that a slightly bent PMB framework is fully accommodated in the polyaromatic shell of 2′ (Fig. 7c).11
 |
| Fig. 7 (a) Schematic representation of the formation of highly emissive host–guest complex 2′·PMB. (b) UV-visible spectra and photograph (H2O, r.t.) of 2′·PMB, 2′, and PMB (in CH3CN). (c) Optimized structure of 2′·PMB (R = –H, all-syn isomer). (d) Fluorescence spectrum (H2O, r.t., 80 μM based on 2′, λex = 500 nm) of 2′·PMB and its photograph (λex = 365 nm). | |
Host–guest complexes featuring high emissivity in water have attracted considerable attention related to biomolecular sensors. However, the majority of coordination cages and capsules disturb guest emission upon encapsulation, owing to the heavy metal effect.3–6 Our previous studies exceptionally demonstrated strong fluorescence (λmax = 535 nm, ΦF = 48%) from a 1
:
1 host–guest complex, composed of anthracene-based capsule 3 and PMB in water.7c Notably, the aqueous solution of 2′·PMB emitted much stronger green fluorescence with a quantum yield of 68% at λmax = 514 nm, upon irradiation at 500 nm (Fig. 7d). The band shape and maximum wavelength are similar to those of free PMB in ethanol (λmax = 522 nm, ΦF = 78%). To the best of our knowledge, this represents the strongest guest emission in coordination host compounds reported so far.7 As compared to capsule 3 with a relatively rigid cavity,9 the flexible naphthalene-based cavity of 2′ is able to adjust to the shape of the bulky PMB guest (Fig. 7c), which reduces the steric host–guest repulsion. Once the cage encapsulates the guest, the flexibility of the naphthalene panels is largely suppressed due to the efficient host–guest interactions. These effects might suppress emission quenching processes, observed in the case of 3·PMB, to a large degree (ΔΦF = +20%).19
Conclusions
We have challenged the perfect conversion of a complex mixture of atropisomeric M2L4 cages into a single isomer by an external stimulus. The cage mixture including up to 42 isomers was generated upon incorporation of two atropisomeric axes into the bent bispyridine ligands of a common and highly symmetrical M2L4 structure. Unusual convergence of the mixed isomers was demonstrated upon encapsulation of guest molecules. Particularly, the encapsulation of a fullerene guest led to the quantitative formation of a single isomer, due to its size and shape complementarity to the spherical host cavity. Thus, the present system can be regarded as a novel dynamic combinatorial library capable of incorporating large guests up to 1 nm. The isomer structure (all-syn) of the M2L4 cage was fully characterized by NMR, MS, UV-visible, X-ray crystallographic, and theoretical analyses. As an additional host function, the cage mixture encapsulated a fluorescent BODIPY dye and the resultant 1
:
1 host–guest complex exhibited intense guest emission (ΦF = ∼70%) in water. We believe that the present study is a small yet first “synthetic” step to approach large and well-organized, biological capsules, composed of multiple unsymmetrical subunits, in vitro.
Conflicts of interest
There are no conflicts to declare.
Acknowledgements
This work was supported by JSPS KAKENHI (Grant No. JP18H01990/JP19H04566) and “Support for Tokyotech Advanced Researchers (STAR)”. We thank Prof. Munetaka Akita and Dr Naoki Noto for helpful discussion and L. C. thanks the JSPS and Humboldt Postdoctoral Fellowship.
Notes and references
- M. G. Mateu, Arch. Biochem. Biophys., 2013, 531, 65–79 CrossRef CAS PubMed.
- Representative examples:
(a) R. Meissner, J. d. Mendoza and J. Rebek Jr, Science, 1995, 270, 1485–1488 CrossRef CAS PubMed;
(b) L. R. MacGillivray and J. L. Atwood, Nature, 1997, 389, 469–472 CrossRef CAS;
(c) J. Rebek Jr, Acc. Chem. Res., 1999, 32, 278–286 CrossRef.
- Representative examples:
(a) M. Tominaga, K. Suzuki, M. Kawano, T. Kusukawa, T. Ozeki, S. Sakamoto, K. Yamaguchi and M. Fujita, Angew. Chem., Int. Ed., 2004, 43, 5621–5625 CrossRef CAS PubMed;
(b) Q.-F. Sun, J. Iwasa, D. Ogawa, Y. Ishido, S. Sato, T. Ozeki, Y. Sei, K. Yamaguchi and M. Fujita, Science, 2010, 328, 1144–1147 CrossRef CAS PubMed;
(c) D. Fujita, Y. Ueda, S. Sato, N. Mizuno, T. Kumasaka and M. Fujita, Nature, 2016, 540, 563–566 CrossRef CAS PubMed.
-
(a) N. B. Debata, D. Tripathy and D. K. Chand, Coord. Chem. Rev., 2012, 256, 1831–1945 CrossRef CAS;
(b) A. Schmidt, A. Casini and F. E. Kühn, Coord. Chem. Rev., 2014, 275, 19–36 CrossRef CAS.
- For recent reviews:
(a) K. Harris, D. Fujita and M. Fujita, Chem. Commun., 2013, 49, 6703–6712 RSC;
(b) T. R. Cook and P. J. Stang, Chem. Rev., 2015, 115, 7001–7045 CrossRef CAS PubMed;
(c) C. J. Brown, F. D. Toste, R. G. Bergman and K. N. Raymond, Chem. Rev., 2015, 115, 3012–3035 CrossRef CAS PubMed;
(d) M. Yoshizawa and M. Yamashina, Chem. Lett., 2017, 46, 163–171 CrossRef CAS;
(e) R. A. S. Vasdev, D. Preston and J. D. Crowley, Chem.–Asian J., 2017, 12, 2513–2523 CrossRef CAS;
(f) L.-J. Chen, H.-B. Yang and M. Shionoya, Chem. Soc. Rev., 2017, 46, 2555–2576 RSC;
(g) S. Chakraborty and G. R. Newkome, Chem. Soc. Rev., 2018, 47, 3991–4016 RSC;
(h) I. Sinha and P. S. Mukherjee, Inorg. Chem., 2018, 57, 4205–4221 CrossRef CAS PubMed;
(i) F. J. Rizzuto, L. K. S. von Krbek and J. R. Nitschke, Nat. Rev. Chem., 2019, 3, 204–222 CrossRef;
(j) M. Yoshizawa and L. Catti, Acc. Chem. Res., 2019, 52, 2392–2404 CrossRef CAS PubMed;
(k) H. Sepehrpour, W. Fu, Y. Sun and P. J. Stang, J. Am. Chem. Soc., 2019, 141, 14005–14020 CrossRef CAS PubMed;
(l) J. E. M. Lewis and J. D. Crowley, ChemPlusChem, 2020, 85, 815–827 CrossRef CAS PubMed.
- In contrast to previously reported, dynamic combinatorial libraries, the present library possesses an isolated cavity capable of encapsulating large guests up to 1 nm in water:
(a) J.-M. Lehn, Chem.–Eur. J., 1999, 5, 2455–2463 CrossRef CAS;
(b) G. R. L. Cousins, S. A. Poulsen and J. K. M. Sanders, Curr. Opin. Chem. Biol., 2000, 4, 270–279 CrossRef CAS PubMed;
(c) J.-M. Lehn and A. V. Eliseev, Science, 2001, 291, 2331–2332 CrossRef CAS PubMed.
- Fluorescent host–guest complexes based on coordination hosts (up to ΦF = 48%):
(a) K. Ono, J. K. Klosterman, M. Yoshizawa, K. Sekiguchi, T. Tahara and M. Fujita, J. Am. Chem. Soc., 2009, 131, 12526–12527 CrossRef CAS PubMed;
(b) P. P. Neelakandan, A. Jiménez and J. R. Nitschke, Chem. Sci., 2014, 5, 908–915 RSC;
(c) M. Yamashina, M. Sartin, Y. Sei, M. Akita, S. Takeuchi, T. Tahara and M. Yoshizawa, J. Am. Chem. Soc., 2015, 137, 9266–9269 CrossRef CAS PubMed;
(d) D. R. Martir, A. Pizzolante, D. Escudero, D. Jacquemin, S. L. Warriner and E. Zysman-Colman, ACS Appl. Energy Mater., 2018, 1, 2971–2978 CrossRef CAS;
(e) T. Tsutsui, S. Kusaba, M. Yamashina, M. Akita and M. Yoshizawa, Chem.–Eur. J., 2019, 25, 4320–4324 CrossRef CAS PubMed;
(f) H. Dobashi, L. Catti, Y. Tanaka, M. Akita and M. Yoshizawa, Angew. Chem., Int. Ed., 2020, 59, 11881–11885 CrossRef CAS.
- Representative examples:
(a) D. A. McMorran and P. J. Steel, Angew. Chem., Int. Ed., 1998, 37, 3295–3297 CrossRef CAS;
(b) L. J. Barbour, G. W. Orr and J. L. Atwood, Nature, 1998, 393, 671–673 CrossRef CAS;
(c) J. E. M. Lewis, E. L. Gavey, S. A. Cameron and J. D. Crowley, Chem. Sci., 2012, 3, 778–784 RSC;
(d) G. H. Clever, W. Kawamura, S. Tashiro, M. Shiro and M. Shionoya, Angew. Chem., Int. Ed., 2012, 51, 2606–2609 CrossRef CAS;
(e) M. Han, R. Michel, B. He, Y.-S. Chen, D. Stalke, M. John and G. H. Clever, Angew. Chem., Int. Ed., 2013, 52, 1319–1323 CrossRef CAS;
(f) D. P. August, G. S. Nichol and P. J. Lusby, Angew. Chem., Int. Ed., 2016, 55, 15022–15026 CrossRef CAS;
(g) V. Martí-Centelles, A. L. Lawrence and P. J. Lusby, J. Am. Chem. Soc., 2018, 140, 2862–2868 CrossRef.
-
(a) N. Kishi, Z. Li, K. Yoza, M. Akita and M. Yoshizawa, J. Am. Chem. Soc., 2011, 133, 11438–11441 CrossRef CAS PubMed;
(b) S. Matsuno, M. Yamashina, Y. Sei, M. Akita, A. Kuzume, K. Yamamoto and M. Yoshizawa, Nat. Commun., 2017, 8, 749 CrossRef PubMed;
(c) M. Yamashina, M. Akita, T. Hasegawa, S. Hayashi and M. Yoshizawa, Sci. Adv., 2017, 3, e1701126 CrossRef PubMed;
(d) M. Yamashina, S. Kusaba, M. Akita, T. Kikuchi and M. Yoshizawa, Nat. Commun., 2018, 9, 4227 CrossRef PubMed;
(e) M. Yamashina, T. Tsutsui, Y. Sei, M. Akita and M. Yoshizawa, Sci. Adv., 2019, 5, eaav3179 CrossRef CAS PubMed;
(f) K. Niki, T. Tsutsui, M. Yamashina, M. Akita and M. Yoshizawa, Angew. Chem., Int. Ed., 2020, 59, 10489–10492 CrossRef CAS PubMed;
(g) N. Kishida, K. Matsumoto, Y. Tanaka, M. Akita, H. Sakurai and M. Yoshizawa, J. Am. Chem. Soc., 2020, 142, 9599–9603 CAS.
- Atropisomeric cages:
(a) T. Kamada, N. Aratani, T. Ikeda, N. Shibata, Y. Higuchi, A. Wakamiya, S. Yamaguchi, K. S. Kim, Z. S. Yoon, D. Kim and A. Osuka, J. Am. Chem. Soc., 2006, 128, 7670–7678 CrossRef CAS PubMed;
(b) S. Arai, D. Niwa, H. Nishide and S. Takeoka, Org. Lett., 2007, 9, 17–20 CrossRef CAS PubMed;
(c) A. Suzuki, K. Kondo, M. Akita and M. Yoshizawa, Angew. Chem., Int. Ed., 2013, 52, 8120–8123 CrossRef CAS PubMed.
- See the ESI.† The geometry optimizations of the cages and host–guest complexes were performed with molecular mechanics (MM) calculations (Forcite module, Materials Studio, version 5.5.3). Particularly, the optimized structures and energies of all-syn/anti2·C60 isomers were calculated on the basis of the crystal structure.
-
(a) Ligand 1 was synthesized in three steps using 3-pyridineboronic acid pinacol ester, 1,4-dibromonaphthalene, and 1,5-dibromo-2,3,4-tri(2-methoxyethoxy)-benzene;11;
(b) The diffusion constant indicates the product size (outer diameter) being approximately 2 nm..
- In the 1H NMR spectrum, the signals of a mixture of 2 in D2O (Fig. 5b) are much broader than those in DMSO-d6 (Fig. 4b), because of strong interactions between the naphthalene-based bispyridine ligands, induced by the hydrophobic effect.
- The ESI-TOF MS analysis of the products elucidated the host–guest ratios (except for the labile 2·Ad complex under MS conditions). In addition, the ratios were also estimated by the 1H NMR integrations of the products after disassembly in DMSO-d6 (at 80 °C for 1 h under high dilution conditions).
- The guest-induced isomerization of coordination cage frameworks is uncommon, whereas there have been several reports on guest-induced structural changes of coordination cages:
(a) M. Scherer, D. L. Caulder, D. W. Johnson and K. N. Raymond, Angew. Chem., Int. Ed., 1999, 38, 1588–1592 CAS;
(b) S. Hiraoka and M. Fujita, J. Am. Chem. Soc., 1999, 121, 10239–10240 CrossRef;
(c) K. Umemoto, K. Yamaguchi and M. Fujita, J. Am. Chem. Soc., 2000, 122, 7150–7151 CrossRef CAS;
(d) D. M. Wood, W. Meng, T. K. Ronson, A. R. Stefankiewicz, J. K. M. Sanders and J. R. Nitschke, Angew. Chem., Int. Ed., 2015, 54, 3988–3992 CrossRef CAS PubMed;
(e) M. Kieffer, R. A. Bilbeisi, J. D. Thoburn, J. K. Clegg and J. R. Nitschke, Angew. Chem., Int. Ed., 2020, 59, 11369–11373 CrossRef CAS PubMed.
- The absorption bands of the electron-deficient C60 guest are sensitive to the polyaromatic host framework due to the efficient host–guest interactions.7f The electron-donating properties of the host framework are decreased by the replacement of the anthracene panels with naphthalene panels so that large blue shifts of the guest absorption bands were observed in 2·C60 as compared with an anthracene-based host–guest analogue.9a.
- The brown block crystals of 2·C60 were obtained by slow concentration of a 2
:
1 acetonitrile/water solution of 2·C60 over 3 weeks at room temperature. Whereas there have been many reports on the successful X-ray crystallographic analysis of M2L4 hosts,4,8,9 reports on host–guest complexes including one C60 guest are still rare.18.
-
(a) C. García-Simón, M. Costas and X. Ribas, Chem. Soc. Rev., 2016, 45, 40–62 RSC;
(b) B. Chen, J. J. Holstein, S. Horiuchi, W. G. Hiller and G. H. Clever, J. Am. Chem. Soc., 2019, 141, 8907–8913 CrossRef CAS PubMed.
- Cage 2′ was chosen for this study due to the pronounced heavy-atom fluorescence quenching effect of the Pd(II)-analogue. A detailed study of the emission dynamics has been performed with 3·PMB, using fluorescence lifetime and femtosecond transient absorption measurements.7c.
Footnotes |
† Electronic supplementary information (ESI) available. CCDC 1907426. For ESI and crystallographic data in CIF or other electronic format see DOI: 10.1039/d0sc03223a |
‡ These authors contributed equally. |
|
This journal is © The Royal Society of Chemistry 2020 |
Click here to see how this site uses Cookies. View our privacy policy here.