The interplay between individual reflection and collaborative learning – seven essential features for designing fruitful classroom practices that develop students’ individual conceptions
Received
9th August 2019
, Accepted 20th March 2020
First published on 25th March 2020
Abstract
Teaching and learning chemistry or science, in general, could be described as building upon learners' existing conceptions. In order to support individual conceptual development, teachers should create opportunities for students to become aware of their thoughts. As this is very demanding in chemistry classroom practice with twenty-five or more individuals, pedagogical approaches and instructional support are needed. We argue for a collaborative learning practice that focuses on sharing and discussing individual conceptions on a chemistry-based phenomenon to build up a joint conceptual understanding. We state seven essential features for integrating this collaborative pedagogy successfully in classroom practice: (1) Becoming aware of one's own conceptions; (2) externalizing individual ideas; (3) initiating comparable situation models; (4) ensuring active involvement of all; (5) offering each learner opportunities to reflect on each other's conceptual understanding; (6) integrating decision-making processes; (7) offering the teacher measures to monitor the learning process. This paper is structured in three parts. Part I gives theoretical evidence to these essential features. Part II introduces the peer-interaction-method (PIM) as one of the possible collaborative learning approaches. The PIM is a pre-structured two-step collaborative learning method with instructional measures to meet the seven features. Part III reports a study with 136 students (grade 8 and 9), learning with the PIM in the context of combustion. The results give evidence to the features as being promising to foster individuals’ conceptual development in science learning. The paper concludes with a discussion, limitations and an outlook for further studies and pedagogies in the context of developing conceptual understanding in collaborative settings.
Introduction
Teaching and learning chemistry or science, in general, could also be described as building upon learners' existing conceptions. Rather than labelling learners' initial ideas as incorrect or as obstacles for subsequent learning processes, they should be regarded as a starting point for meaningful learning situations as they provide potential opportunities for reorganizing or constructing (new) knowledge (Taber, 2001b; Duit et al., 2012). Referring to this, traditionally designed classroom practice is less suitable for adequate support of students’ conceptional development (e.g.Wandersee et al., 1994; Özmen and Ayas, 2003) due to its non-constructivist orientated perspective (Duit et al., 2013). Instead, conceptual change-oriented teaching methods are needed as they explicitly focus on students' individual conceptions (Duit et al., 2013). In order to support individual conceptual development, teachers should create opportunities for students to become aware of their thoughts (e.g.Thomaz et al., 1995; Van Boxtel et al., 2000), to externalize them in a variety of ways (e.g.Treagust et al., 2003) as well as to share and develop them discursively with peers (Tao and Gunstone, 1999). As it is very demanding for teachers to provide this individual assistance extensively, pedagogical approaches and instructional support are needed (see also Knox et al., 2019). In this regard, we argue for a collaborative learning practice that meets the following seven essential features:
F1. Becoming aware of one's own conceptions: successful collaborative processes require measures that allow each learner to become aware of his or her own conceptions.
F2. Externalizing individual ideas: providing several ways to create external representations allow learners to externalize different conceptions comprehensively.
F3. Initiating comparable situation models: a comparable situation model among all learners is conducive to develop shared understanding.
F4. Ensuring active involvement for all: each group member needs to play an active role in a collaborative setting.
F5. Offering each learner opportunities to reflect on each other's conceptual understanding: being aware of different perspectives on a concept will enhance the scope to reflect on the respective concept.
F6. Integrating decision-making processes: building up a common knowledge base as one important goal of collaboration could be supported through negotiation processes.
F7. Offering the teacher measures to monitor the learning process: group results and uncovered varying students’ conceptions give the teacher feedback of his or her teaching and provide opportunities for individual support and further classroom practice.
This paper is structured in three parts. Part I gives evidence to these features, based on research in the relevant fields of chemistry education and collaborative learning. Part II gives a short overview of learning approaches that use the first-individual-than-collaborative principle and introduces the peer-interaction-method (PIM), a comparable collaborative learning method with instructional measures to meet the seven features and contrast it against the other approaches. Part III reports a study with 136 students (grade 8 to 9, 13–15 year), learning with the PIM in the context of combustion. The results give evidence that the PIM is a promising approach to foster individuals’ conceptual development in science learning. It also sheds light on the potential of each feature which will also be discussed for their contribution to other collaborative learning approaches. The paper concludes with limitations and an outlook for further studies in the context of developing conceptual understanding in collaborative settings.
Part I: seven essential features for developing conceptual understanding in collaborative settings
Theoretical background
Before elucidating the seven features (F1–F7) based on literature, we define our understanding of learner conceptions and collaborative learning.
Learner conceptions
In the last decades, the existence and origins of students' conceptions of scientific phenomena is widely discussed in research literature (e.g.Driver, 1981; Garnett et al., 1995; Duit et al., 1996; Treagust et al., 2000; Taber, 2001a; Morrison and Lederman, 2003; Özdemir and Clark, 2007). In this article, we define all conceptions learners, students and even teachers or experts can have as learner conceptions; whether they are formed spontaneously (e.g.DiSessa, 2008; Amin, 2009) or can be regarded as consistent (e.g.Vosniadou, 2013) and also independent of their scientific accuracy. In many cases, learner conceptions are based on everyday life observations. Even if these everyday life conceptions are incongruent compared to scientific explanations, they often represent fruitful explanations in their daily contexts (Wandersee et al., 1994; Duit et al., 2012). Additionally, chemistry lessons themselves can serve as an origin for building up scientifically inadequate conceptions (Taber, 2001b). In every case, scientific accepted (also canonical) and alternative conceptions should be considered to build up a sustainable knowledge base (e.g.Duit et al., 2012).
Collaborative learning
Following Dillenbourg (1999) or Hathorn and Ingram (2002), collaboration describes the interdependence of group participants as they share unique ideas and experiences. All group members work on a problem and the individual contributions of the participants often cannot be separated in the final project. In contrast to cooperation that allows and encourages distributed knowledge, effective collaboration leads to shared knowledge as learners have the same concepts as their learning partners (Weinberger et al., 2007).
According to our understanding of learner conceptions, classroom practice should provide each learner opportunities to build upon his or her understanding of the chemical concepts. In particular in the first years of chemistry learning students should learn and be aware of how close scientific concepts like a solution, dissolution, diffusion or combustion are connected to everyday life experiences; that both perspectives, the scientific and the everyday-life, share a terminology but may differ in their meaning (e.g.Taber, 2019). The understanding of chemical concepts is based on the understanding of the particular nature of matter. Classroom practice has the goal to develop this concept and take care that it will not interfere with macroscopic entities. In the following we describe seven features, each to be fruitful for supporting such learning objectives and essential for conceptual development in a collaborative learning practice.
Essential feature 1: becoming aware of one's own conceptions
Learning science requires both, an individual process of knowledge construction or meaning-making as well as social interactions (e.g.Driver et al., 1994; Morrison and Lederman, 2003). Van Boxtel et al. (2000) argue that an individual reflection process of one's understanding is a basic requirement for collaborative exchange. Following their results, it is considered that this individual phase must be long enough for students to become aware of their conceptions sufficiently. Allowing every single student to reflect on his or her conceptual knowledge and adding or linking new ideas to existing ones is one promising means to build up a fundamental understanding of scientific concepts (Donovan et al., 1999; Davis, 2003; Land and Zembal-Saul, 2003).
Essential feature 2: externalizing individual ideas
Being aware of one's conceptions is a necessary but often not sufficient feature for reflecting on these. When confronted with other views, the learner needs to judge explicitly on whether and how a view differs or resembles his or her understanding. Therefore, for all kinds of individual or collaborative reflection processes students need tools or methods that help them to make their thinking visible and improvable in further learning processes as external representations (Donovan et al., 1999; Linn, 2000). These external representations support all communication processes as learners can reflect and talk about what they see. Appropriate tools could be text- or picture-based. Due to the different nature of textual and pictorial representations, Tversky (2001) argues that both, writings and drawings, are representations of cognitive processes. Written representations often describe scientific phenomena in a string linear way whereas e.g. static or dynamic pictorial models help to understand spatial attributes that cannot be described in texts the same way (Gobert, 2005). In chemistry, text and drawings can be used to explain conceptions on two distinctive conceptual levels (e.g.Johnstone, 1991; Taber, 2013), the macroscopic (e.g. liquid or solid-state), the submicroscopic level (e.g. structure of molecules) or as a hybrid of both levels (Devetak et al., 2009; Akaygun and Jones, 2014). The submicroscopic level is based on existing mental models and abstractions. The process of conceptualization does not occur exclusively by means of subject-specific expressions but can include all kinds of symbolic representations like formulas. According to Taber (2009), element symbols and chemical reaction equations can act as a bridge between the macroscopic and the submicroscopic level.
In many classroom situations, however, learners are neither forced to create drawings by themselves (Ainsworth et al., 2011; Wu and Rau, 2019), nor are they used to reflect on their understanding collaboratively based on external representations at all. Usually, drawings or diagrams are given to students as a tool for interpreting specific phenomena or for solving specific tasks (Davidowitz et al., 2010). When students create drawings on their own, they could gain another and sometimes deeper insight into the understanding of the underlying conceptions compared to creating texts. Rotbain et al. (2005) showed an interrelationship between the integration of drawing-tasks into a traditional learning process and the development of a deeper conceptual understanding. Therefore, both types of external representations, textual and pictorial, help students to self-reflect their understanding and becoming aware of conceptual interrelations and inconsistencies when describing chemical processes in dependence on the different levels of representation. In summary, allowing students to document their conceptions of chemical phenomena multimodal as texts and drawings are necessary, to support their understanding holistically (Rennie and Jarvis, 1995; Treagust et al., 2003; Köse, 2008; Nyachwaya et al., 2011; Akaygun and Jones, 2014).
Essential feature 3: eliciting common situation models
From a cognitive perspective of learning, learners’ conceptions could be highly fragmented and represented differently when acquired in different contexts (e.g.Boujaoude, 1991; Taber, 2000). Accordingly, being in a situation that is similar to a former learning situation may more likely activate parts of the concept that are connected to that former situation than other parts. For instance, observing a burning candle might activate other fragments of the concept “combustion” than presenting a small piece of charcoal or diamond glowing and disappearing in an oxygen atmosphere. We call this process of activating context-specific knowledge eliciting a situation model (e.g.Glaser, 1991, see also Caravita and Halldén, 1994). The situative perspective of learning considers these situation models as different aspects of learning practice in a socially organised activity (e.g.Anderson et al., 2000). The group members’ prior individual conceptions may differ but should all refer to a common frame of reference for discussing, judging, or interpreting individual ideas against the background of this framework, just to minimize the potential of misunderstanding.
Essential feature 4: ensuring active involvement of all
In successful collaborative processes, all members of the group are involved in the achievement of the common goal equally and at any time (Van Boxtel et al., 2000). Also, several studies showed that the students’ perception of how well their work is represented in the final result has a big influence on collaboration within the group (Webb, 1989; Lehtinen et al., 1999; Le et al., 2018). In some cases, learners are not used to collaborative learning situations as it is still not common in classroom practice. Collaborative processes, therefore, can be very demanding for students and teachers as well (Saab et al., 2007). The process can benefit from instruction that guides the communication and interaction processes (see also Kollar et al., 2006).
Essential feature 5: offering each learner opportunities to reflect on each other's conceptual understanding
According to studies of Roschelle (e.g.Roschelle, 1992, 1996; Roschelle and Teasley, 1995), convergence plays a crucial part in collaborative settings. The learners’ activities have an impact on those of their partners. Knowledge acquisition also depends on what the learning partners do and how they present their knowledge. One promoting aspect of this social interaction is the awareness of what the learning partners know. (Content-specific) Visualisation tools are supportive of these processes (see Fischer et al., 2002). Stoyanova and Kommers (2002) argue that presenting and explaining individual externalisations (e.g. concept maps) will force each group member to reflect on other perceptions of the learning object and also to reflect one's conceptual understanding which leads to a “distributed cognition” (p. 112). Also, Jeong and Chi (1997) showed in an exploratory study that creating a common product (e.g. a drawing) can help students to develop their conceptions in a more scientifically appropriate way.
Essential feature 6: integrating decision-making processes
As soon as learners become aware that there are several possible perspectives on the learning object (F5), the need to decide on a common solution arises. Successful discursive processes are characterised by a well-grounded decision for one solution and against inherent alternatives. As this is still unusual in classroom practice and could put additional cognitive effort on students (e.g.Repice et al., 2016), it needs instructional support. Mercer et al. (1999) define the idea of exploratory talk as a measure to engage partners critically but constructively in a process to reflect each other's ideas. Based on their former research, e.g.Mercer (1995) and Wegerif and Mercer (1997) specify 7 ground rules for generating exploratory talk: “1. all relevant information is shared; 2. the group seeks to reach agreement; 3. the group takes responsibility for decisions; 4. reasons are expected; 5. challenges are accepted; 6. alternatives are discussed before a decision is taken; and 7. all in the group are encouraged to speak by other group members” (Mercer et al., 1999, 98f.).
Essential feature 7: offering the teacher measures to monitor the learning process
As mentioned before, the students' conceptions are seen as a starting point for planning and monitoring classroom practice as they can have a significant impact on further learning processes (Driver et al., 1994; Treagust, 2006). Common methods for diagnosing students' conceptions, including interviews, open questions, single- or multiple-choice tests (Gurel et al., 2015), are often time-consuming. Taber (2001a, 2001b) points out that these methods are, therefore, less suitable for common classroom practice. Also, teachers need elaborated methodological knowledge for some of these methods (such as interviews) (Taber, 2001a, 2001b). These should be easy to use, efficient concerning the time to use and analyse the data and reliable and valid for the collected data. Gurel et al. (2015) report that diagnostic methods for learners’ conceptions usually focus on verbal or numerical data, whereas the combination of drawings and written explanations is said to be useful to identify conceptions holistically (Rennie and Jarvis, 1995; Köse, 2008; Nyachwaya et al., 2011; Akaygun and Jones, 2014). The monitoring process should serve to explore the stability of student conceptions as well as conditions that lead to their change (e.g.Özmen and Ayas, 2003).
Part II: bringing the seven essential features into classroom practice – the peer-interaction-method (PIM)
Collaborative learning is discussed as an effective student directed learning method that initiate fruitful interaction, argumentation, and negotiation processes (e.g.Slavin, 1996; Dillenbourg, 1999; Schraw et al., 2006). Research results also indicate that implementing collaboration into classroom practice does not always proceed as intended and also does not guarantee expected learning gains per se (Olsen et al., 2019). Referring to conceptual development as one learning gain, this could be drawn back to the fact that one or more of the seven essential features (F1–7) were neglected. Nevertheless, more research is needed even to demonstrate the importance of these features for successful collaborative learning in classroom practice.
We developed the Peer-Interaction-Method (PIM) as a structured and scaffolded collaborative setting. It is just as well designed for students to share and reconstruct their ideas at a peer level as for teachers to efficiently monitor students' conceptional development on a specific topic. The PIM consists of two phases (Fig. 1) an individual (IP) and a collaboration phase (CP).
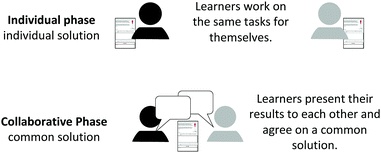 |
| Fig. 1 Two phases of the PIM here illustrated for dyads. | |
Combining individual and group learning phases is very common in classroom practice. In the following section we give a short overview of three documented approaches (Think-Pair-Share, Jigsaw classroom and Team-based-Learning) and report empirical results that illustrate the potential of the approaches, focussing on science learning. We complete Part II with a detailed description of the PIM.
Think-pair-share (TPS)
The method Think-Pair-Share (TPS) firstly proposed by Lyman (1981), see also Raba (2017) describes a basic structure to provide students to think and reflect on an answer for a given task (think), then to practice stating the thoughts with a peer (pair), before presenting the answer to a whole learning group (share). The method is described as easy to prepare and not time-consuming. However, variations like Think-Write-Pair-Share (e.g.Bauer et al., 2013) integrate phases that do not only rely on spoken communication.
Research on integrating TPS in classroom practice report positive effects in different disciplines e.g. on improving students’ oral communicative skills (Raba (2017), university level, English for nonnative speakers), critical thinking skills (Kaddoura (2013), baccalaureate nursing students) higher quality cognitive and problem-solving skills (Bamiro (2015), secondary school, chemistry guided discovery learning), or on conceptual learning and epistemological beliefs (Gok (2018), first-year university physics). Overall students felt more comfortable when sharing ideas on a peer level and felt more responsibility towards learning in the pair situation (e.g.Raba 2017, Gok, 2018).
Jigsaw classroom
The jigsaw classroom (Aronson, 1978) is a script that combines individual phases with cooperative (expert groups) and collaborative (home or jigsaw groups) learning phases. The idea is to bring fragments of knowledge from individual experts into a group and connect them all to reach a common goal.
Studies that compare the jigsaw classroom to traditional teaching report positive changes in the students’ attitudes towards the learning content (Artut and Tarim, 2007, Gambari and Yusuf, 2017), their ability to teach concepts (Crone and Portillo 2013), or even an increase in conceptual understanding (Doymus et al., 2010). Other studies focus on general affective changes due to the peer teaching situation when the individual experts return to their home groups and are in charge to teach the other group members. They report an increase in feeling competency (Berger and Hänze, 2009), in students’ engagement (Perkins and Tagler, 2011) or motivation (Hidi et al.,1998; Berger and Hänze 2009). Further studies use the jigsaw classroom to create a collaborative learning situation and rely on the potential of the method reported, e.g. in Aronson (1978), without even systematically verifying it against alternatives (e.g.Eilks, 2005).
Team-based learning (TBL)
Team-Based Learning (TBL) (e.g.Michaelsen and Sweet, 2011) is a practice that strategically organises students in individual and group learning activities to ensure both, conceptual and procedural knowledge. One TBL sequence has five to seven instructional units from individual study over diagnostic- and feedback-phases to the application-oriented activities and usually lasts several hours.
Michaelsen and Sweet (2008) describe four essential elements for learners to ensure cohesive learning teams: (1) Groups: groups must be properly formed and managed, (2) Accountability: students must be accountable for the quality of their individual and group work, (3) Feedback: students must receive frequent and timely feedback, and (4) Assignment design: group assignments must promote both learning and team development. With the structure and also with these four elements TPS explicitly address essential features. E.g., in the individual study activity students get the chance to become aware of their own (conceptual) understanding (F1) and elicit a common situation model (F3). The group work ensures active involvement (F4), offers each learner opportunities to reflect on each other's (conceptual) understanding (F5) and integrates decision-making processes (F6).
Research on TBL mainly focusses on higher education levels (e.g. in chemistry Wiegant et al., 2012; Evans et al., 2016; Alvarez-Bell et al., 2017). Reviews report that TBL improves students’ end of course grades, test performance, and classroom engagement (Swanson et al., 2019). However, in their meta-analysis Swanson et al. (2019) argue that these effects are mainly based on the assessment components of TBL. Students are motivated to perform well in the individual quizzes to be well prepared for the knowledge application activities as well as they want to perform well in front of their peers in the team quizzes. Nevertheless, this meta-analysis reports also a social condition effect of TBL in favour of small group sizes. Like in other collaborative learning practices a key feature is the co-construction of meaning through discursive processes that are better supported in groups smaller than five learners.
Peer-interaction-method (PIM)
The PIM has its pedagogical roots in the TPS. Similar to the TBL, it provides definite instructional measures in the individual and in the collaborative phase, supported by specially designed worksheets. In contrast to the jigsaw classroom, it has no phase that creates distributed knowledge but uses the individual phase to bring expertise into the collaborative learning phase as each learner is an expert of his or her externalisations.
Individual phase (IP) of the PIM
Structure of the worksheets and implementation.
The sheets consist of at least three different components (preliminary paragraph, written tasks, drawing tasks – see Appendix 1). The preliminary paragraph aims at building up a common situation model (F3) and unambiguous technical terminology. The preliminary paragraph is followed by a variable number of different tasks mostly (but not necessarily) in a two-tier-format (e.g.Treagust, 1988). The first tier is either closed or open-ended. Distractors of closed questions (e.g. multiple select) should authentically address learner conceptions. In our tasks, the distractors are derived from a literature review and address common learner conceptions. The second tier usually asks for an explanation of the student's choice through short sentences. These initial questions are intended to activate learners’ conceptions about the underlying phenomena (F1) which will be important for the knowledge exchange processes in the second phase of PIM. The first or second tier can also address a symbolic representation, e.g., by asking for a reaction equation. As the same terms used by different learners do not necessarily refer to the same mental model, the students should always be asked to create and explain a drawing (preferably representing the submicroscopic level) in another task. Using these different modes of representation serves to enable the students to externalise a comprehensive concept (F2).
Collaborative phase (CP) of the PIM
Structure of the exercise sheets and implementation.
The collaboration part should take place in small groups from two up to four students. Van Boxtel et al. (2000) argue that collaborative processes in larger groups can lead to asynchronous participation. Also, cognitive overload is possible due to the number of different students' conceptions (Van Boxtel et al., 2000). The group gets one single new worksheet consisting of the same IP tasks. In addition, the worksheet asks in every task each student to present and explain his/her individual solution to the peers (F4). As part of this exchange process, students need to be actively engaged in terms of understanding, questioning or arguing for the solutions. The group then has to agree upon one joint solution for the collaborative worksheet (F5–6). The students are explicitly free to choose a solution of one group member, a combination of single solutions, or to create an entirely new answer. It is important that this answer has to be supported and accepted by each single group member (Tao and Gunstone, 1999).
This exchange process is scaffolded by a collaboration script on the worksheet (Kollar, et al., 2006; Fischer et al., 2007; Rau et al., 2017; Wang and Mu, 2017), short enough to leave room for the constructive process of collaborative learning and to reduce the risk of over-scripting (Dillenbourg, 2002) to a minimum (see Appendix 2).
Part III – the PIM and its potential of fostering conceptual understanding
We collaborated with teachers that used PIM tasks in classroom practice and gave us feedback to improve the tasks and instructions in an iterative cycle over three years (Schanze and Busse, 2015). In the following, we report results from one study that was designed to illustrate the PIM and how successful collaborative learning could be drawn back to the seven features. Furthermore, we will also bring up open questions for future research. To structure the process of discussing the potential of the PIM according to the seven features, we formulate two research questions. The first addresses the aspect of revealing students’ conceptions. The second one focusses on the collaborative setting according to examine a change from the individual to the group work results.
Research questions
RQ (1) To what extent does the peer-interaction-method encourage students to externalize their individual conceptions (here about processes of combustion)?
RQ (2) To what extent can differences be identified between the IP and CP worksheet results?
Answering RQ 1 will give us an insight about the potential of how the method will provide students the opportunity to reflect on their own conceptual understanding (F1) in different representational modes (F2) in the IP and also provide a basis for active involvement (F4) and an interactive reflection on different conceptions (F5) in the CP. At least the quality of the externalisations could indicate the potential for the teacher getting an overview of the learners’ conceptual understanding for monitoring future classroom practice (F7). The results from RQ 2 serve to obtain evidence about the process of developing conceptual understanding and sharing a joint knowledge base (F6) that implicitly indicates a quality of the reflective processes (F4–5) based on a common situational model (F3). F3 could be examined more directly by analysing the protocol of the dialogues, which will not be part of this paper. We will come back to this in the limitation part below.
Although, we developed a set of PIM tasks referring to different concepts (e.g. strong and weak acids, solution and diffusion, bonding or dynamic equilibrium), in this study we only use one task about the reaction of carbon (here charcoal) in an oxygen atmosphere. Nevertheless, the other tasks are based on the same PIM framework described in Part II. The study-task addresses the concept of combustion and mass conservation (realizing oxygen as a reactant and gases as substances with a noticeable mass), which will develop an understanding of the chemical reaction on the macroscopic as well as on the submicroscopic level. It builds upon everyday life experiences of combustion of organic substances. For a better understanding of the study, we start with a small paragraph about the current status of known learner conceptions about burning and combustion before reporting the research design and results.
Learners’ conceptions about burning or combustion
Over the last forty years, learners’ conceptions are reported for various chemistry topics (for an overview see Duit, 2009). Some studies deal with the processes of burning or combustion as well as the mass conservation in closed systems (e.g.Meheut Saltiel and Tiberghien, 1985; Andersson, 1990; Stavy, 1990; Boujaoude, 1991; Prieto et al., 1992; Haidar, 1997; Özmen and Ayas, 2003; Lee and She, 2010; Yan and Talanquer, 2015). Findings by Meheut et al. (1985) show two different learner conceptions of combustibility: one describes a process synonymous to the reversible change of physical conditions (e.g. melting of wax), and the other an irreversible and destructive change mostly into a new substance (e.g. burning wood to ashes) without taking gaseous reaction products into account. Both conceptions were closely connected to everyday life observations. These results are also in line with Boujaoude (1991), who used data from interviews and observations in chemistry lessons to examine junior high school students’ understanding. Özmen and Ayas (2003) reported students’ conceptions that “a solid is heavier than a gas” and that “when a chemical combustion happens in a closed system, the total mass decreases” (p. 287).
Everyday conceptions were proven to be fruitful for the students for many years. Therefore, they are stable (e.g.Carey, 1985; Vosniadou and Brewer, 1987; Celikten et al., 2012; Leppävirta, 2012) and resistant to change. In order to develop a scientifically accepted understanding of combustion, Andersson (1990) and Prieto et al. (1992) emphasize that students need a deep understanding of the law of mass conservation as well as the role of gases as part of chemical reactions. In the context of a closed system, the students should learn that the mass of the system remains the same during the chemical reaction although, e.g. we see solid substances like coal disappear. Students should then understand the explanation on the submicroscopic level that atoms neither can be destroyed nor formed during a chemical reaction; while on the macroscopic level we observe educts to vanish and other substances (products) to appear.
Based on previous studies about processes of burning and combustion (e.g.Meheut et al., 1985; Boujaoude, 1991; Prieto et al., 1992; Özmen and Ayas, 2003; Hundertmark and Schanze, 2017), we identified 55 different learner conceptions that could be assigned to five categories potential mass change during the experiment, the process of combustion, potential reactants and products, prerequisites for the combustion, and anthropomorphic and animistic conceptions (see Table 1 with illustrating examples). Three categories (the process of combustion, potential reactants and products as well as prerequisites for the combustion) were developed based on a category system suggested by Watson, Prieto and Dillon (1995). The remaining two categories mass conservation and anthropomorphic and animistic conceptions are added based on further findings like in Hundertmark and Schanze (2017) to capture aspects of the concept that are not mentioned explicitly by Watson, Prieto and Dillon (1995). In coherence with our definition of learner conceptions, we firstly do not judge them to be adequate or not, so these 55 conceptions are a list of scientific accepted as well as everyday conceptions.
Table 1 Examples of conceptions about the phenomenon combustion illustrated by students’ statements; in parentheses is given the internal code that refers to one of the 55 conceptions
Conception and reference |
Example from our data |
Gases weigh less than solids (C3) (Özmen and Ayas, 2003) |
|
Translation: “Since there is no coal left in the end, there is only carbon dioxide, which can have weight. But that does not have as much weight as coal” |
Reactants (e.g. coal) evaporate (C11) (Andersson, 1990; Boujaoude, 1991; Watson et al., 1997) |
|
Translation: “solid coal + heat → gaseous coal. Due to the heating, the coal changes its state of aggregation” |
Coal atoms reacts with oxygen molecules to form carbon dioxide molecules/Coal reacts with oxygen to form carbon dioxide (C32) (Wiberg et al., 2001) |
|
Participants and data collection
The study took place in different grammar schools (Gymnasium) in Germany. A total of 136 students (55.1% female, grade 8–9, age 13 to 15 years) participated in this study, grouped in 68 dyads. The students were voluntarily recruited by the respective teachers and free to choose their partner. The instructor had no influence on the individual or the collaborative phase. Furthermore, students did not receive any feedback on their individual or collaborative products during the intervention. For the role of the instructor, it is precisely what we expect from the teacher to do in classroom practice. Thus, the experimental setting is very close to the intended classroom situation, which supports the ecological validity of the study. In accordance with Taber (2014), all students were informed that they were participating in a study. They were told that this participation is voluntary and anonymous and that they were free to withdraw at any time. Students were also informed that none of their answers affected their grades and that their teachers were not informed about their answers. The written permission of parents and students to participate has been obtained. All parents and students agreed on us using their responses and artefacts for publications. We told them that the aim of the study is, to gather information about students’ understanding of chemical phenomena, to improve classroom teaching. The teachers confirmed that their classes were familiar with the topic of the worksheet (Boyle's flask: combustion of carbon in an oxygen atmosphere). The completed worksheets were collected from both phases.
Data analysis
For analysing the worksheets to answer RQ 1 and 2 we transferred data from all worksheets to digital data using Microsoft Excel 2016. Equation schemes and drawings were scanned. Data were analysed in the native language German. We developed a coding manual with four different schemes: 1. Learners’ conceptions, 2. Comparing IP and CP, 3. Scientific accuracy, and 4. Level of representation. Schemes 1–4 were all applied to the IP and CP worksheets.
(1) Learners’ conceptions: we used the above-mentioned synopsis of 55 learner conceptions about combustion to categorise the students’ statements.
(2) Comparing IP and CP: this coding serves to give information about the origin of the CP: if it was based on one of the two IP (with the special case that both IP are identical), a mixture of both IP or a completely novel solution (see Table 2).
Table 2 Coding rule for characterising the CP results based on the IP results
Code |
Coding rule |
One-sided adoption |
All elements of a common solution (A) are identical to an individual solution (A) of one student. |
A + B → A |
Two-sided adoption |
All elements of the common solution (A) are identical to the individual solutions (A, A′) of both students (students’ individual solutions are nearly identical). |
A + A′ → A |
Mixed solution |
The common solution (C) is a mixture of single elements from different individual solutions (A, B). |
A + B → C, C has parts of A and B |
Novel solution |
The common solution (D) consists of at least one element, which does not exist in both individual solutions. |
A + B → D, D differs at least in one part from A and B |
(3) Scientific accuracy: inorganic chemistry textbooks were used by the authors for indicating scientifically adequate conceptions. This process was guided by a prior analysis of the Lower Saxony school curriculum for the corresponding grades (8–9). The curriculum includes, for example, that the students should know the law of mass conservation. Building upon this, the three-level evaluation system inadequate, potentially adequate, and adequate was developed.
• The highest level of accuracy adequate includes only scientifically correct conceptions.
• Potentially adequate answers consist of adequate propositions but do not reason sufficiently.
• The lowest level inadequate explicitly indicates scientific incorrect conceptions.
This category is very strict but goes in line with our view of learner conceptions. As long as the statement of a learner cannot be characterised as clearly correct (referring to a reference system), we should not assign the ownership of an adequate conception to the learner. E.g., the proposition “In a chemical reaction a reactant disappears/is destroyed” is scientifically adequate on a macroscopic level and a necessary condition for assigning a conceptual understanding of the chemical reaction. But it is not sufficient as a chemical reaction also is characterised by the generation of new substances (reaction products). Without the second proposition, the learner could as well have the concept that reactants are destroyed irrecoverable and without any trace – he or she might ignore gaseous products like carbon dioxide because he or she does not recognize them.
We categorized answers from the open-ended questions and drawings into these three levels (examples see Table 3). In this process, at least two experts were involved. We used scientifically accepted conceptions as a reference framework. The coding scheme from inadequate to adequate, therefore, is not depending on the applied worksheet or the topic combustion and could be transferred to every other data about conceptual understanding in science.
Table 3 Coding rules for task 1b to judger the scientific accuracy of students’ statements
Code |
Coding rule |
Example |
Inadequate |
The students … |
|
… do not refer to the solution they have chosen in exercise T1a or |
Translation: “After the experiment, the piece of coal weighs less than in the beginning.” |
… do not address the task or |
… use concepts that are not in line with scientifically adequate conceptions |
Potentially adequate |
The students … |
|
… refer to their answer in T1a and reason their selection. |
Translation: “It remains 200 g, since the coal does not disappear out of the glass.” |
… mention either a closed system or the conservation of atoms/matter |
… mention no inadequate conceptions |
… can mention the emergence of a new product |
Adequate |
The students … |
|
… refer to their answer in T1a and reason their selection. |
Translation: “In a chemical reaction, no atoms or substances are created or disappear (→mass conservation: “The sum of the mass of the reactants is equal to the sum of the mass of the products”). The flask is sealed airtight → gases cannot escape.” |
… mention a closed system and the conservation of atoms/matter |
… mention no inadequate conceptions |
… mention the emergence of a new product in combination with the disappearance of reactants |
(4) Level of representation: based on Johnstone (1991), Taber and Bricheno (2009) and Taber (2013), we categorise the reaction equations (task 2) as a word or symbol equation or a hybrid version of it.
• In a word equation, the written names of the substances are used (e.g. carbon + oxygen → carbon dioxide).
• A symbol equation uses chemical symbols (e.g. C + O2 → CO2).
• A hybrid version contains both words and chemical symbols.
The drawings (task 3) were categorized as
• referring to the macroscopic level or
• referring to the submicroscopic level or
• referring to a mixture of both.
In total all open-ended tasks from 204 worksheets (136 from IP and 68 from CP) were analysed: 1b – the reason for the choice in 1a, 2 – the reaction scheme and its explanation, and 3 – the drawing of the process. All data were coded by two independent persons. The inter-rater reliability (Cohens Kappa) was κ ≥ 0.83 for all different schemes and tasks (Range = 0.83–0.97, M = 0.92, SD = 0.04). Disagreements were discussed and could be resolved in any case for improving the coding quality. To evaluate possible differences between the IP and the CP worksheets regarding the Schemes 1–4, we used the Wilcoxon-Signed-Rank test. For each student, the IP worksheet results were compared to the CP worksheet results task by task (see Fig. 2). For this, the respective levels of the three single Schemes 1–3 were assigned the ranks 0–2 (see Table 3). The group results (CP) then were each compared with the respective individual results (IP). This means that, for example, in the case of Scheme 2 and for one group, the individual result of the first student (e.g. an inadequate individual result, rank 0) as well as the individual result of the second student (e.g. potentially adequate, rank 1) were compared with the group result of both students (e.g. potentially adequate, rank 1).
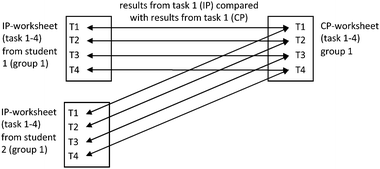 |
| Fig. 2 Schema of group comparisons. | |
Results
RQ 1: PIM and the externalisation of students' diverse conceptions about combustion
In total, the 204 worksheets (IP and CP) reveal 2227 conceptions or graphical representations about combustion that were assigned to 33 out of the 55 known learner conceptions (22 different conceptions in 1b, 24 in 2 and 26 in 3). 24.8 percent of these conceptions were rated as inadequate conceptions, though they were unequally distributed between the three tasks 1b: 55.4%, 2: 7%, and 3: 35.1% (see Table 4).
Table 4 Overview over the 33 diagnosed conceptions in this study. (*) scientific adequate conceptions; in parentheses is given the internal code that refers to one of the 55 conceptions (see Appendix 4 for a complete list of the 55 conceptions)
Category |
Conception |
Task 1b (N = 204) |
Task 2 (N = 204) |
Task 3 (N = 204) |
Total (N = 612) |
N (%) |
N (%) |
N (%) |
N (%) |
Potential mass change during the experiment |
Submicroscopic entities (e.g. atoms) are destroyed/macroscopic entities (e.g. substances) are destroyed without mentioning the emergence of new entities (C1) |
13 (6) |
2 (1) |
7 (3) |
22 (4) |
Gases weigh less than solids (C3) |
33 (16) |
|
|
33 (5) |
Gases weigh nothing (C4) |
7 (3) |
|
|
7 (1) |
Due to the buoyancy of gases, the flask weighs less (C5) |
10 (5) |
2 (1) |
3 (1) |
15 (2) |
Due to another modification of the substance, the flask weighs less (C6) |
10 (5) |
|
|
10 (2) |
Due to another modification of the substance, the flask weighs more (C7) |
3 (1) |
|
|
3 (<1) |
(*) The number of atoms remains the same in a chemical reaction (C9) |
35 (17) |
2 (1) |
10 (5) |
47 (8) |
(*) There is no mass transfer with the environment (C10) |
52 (25) |
|
|
52 (8) |
Process of combustion |
Reactants (e.g. coal) evaporate (C11) |
16 (8) |
12 (6) |
82 (41) |
110 (18) |
Oxygen transform into another product (e.g. soot) (C13) |
1 (<1) |
|
|
1 (<1) |
The appearance or properties of some reactant have changed, the substance (e.g. coal) still exists (C14) |
26 (13) |
11 (5) |
84 (41) |
121 (20) |
Coal atoms are destroyed/Coal is destroyed without mentioning the emergence of new substances (C15a) |
23 (11) |
7 (3) |
3 (1) |
33 (5) |
(*) All carbon atoms are bound forming a new molecule/Coal reacts with mentioning the emergence of a new product (C15b) |
13 (6) |
156 (76) |
99 (49) |
268 (44) |
Oxygen molecules are destroyed/Oxygen is destroyed without mentioning the emergence of new substances (C16a) |
1 (<1) |
2 (1) |
2 (1) |
5 (1) |
(*) All oxygen atoms are bound forming a new molecule/Oxygen reacts with mentioning the emergence of a new product (C16b) |
10 (5) |
156 (76) |
95 (47) |
261 (43) |
Reactants transform into new substances (C18a) |
|
1 (<1) |
|
1 (<1) |
Reactants react into some new substances (C18b) |
9 (4) |
149 (73) |
85 (42) |
243 |
Reactants (e.g. coal) dissolves and there is no substance left (C19) |
8 (4) |
7 (3) |
17 (8) |
32 (5) |
Reactants transform into Energy/light/heat (C21) |
|
1 (<1) |
|
1 (<1) |
Energy/light/heat transforms into a substance, which can transform into other substances afterwards (C22a) |
|
1 (<1) |
1 (<1) |
2 (<1) |
Coal atoms reacts with oxygen molecules to form carbon dioxide molecules/Coal reacts with oxygen to form carbon dioxide (C32) |
6 (3%) |
152 (75) |
93 (46) |
251 (41) |
The product is created by mixing a substance with the oxygen instead of a chemical reaction (C36a) |
|
5 (2) |
14 (7) |
19 (3) |
Substances (e.g. coal) contain the product (e.g. carbon dioxide), that is release (C40) |
2 (1) |
|
1 (<1) |
3 (<1) |
Potential reactants and products |
(*) An oxygen molecule consists of two oxygen atoms (C23) |
|
67 (33) |
45 (22) |
112 (18) |
(*) Coal is made up of carbon atoms (C25) |
|
91 (45) |
42 (21) |
133 (22) |
Ash is a product (C26) |
3 (1) |
2 (1) |
3 (1) |
8 (1) |
Carbon monoxide is the only product (C27) |
|
3 (1) |
11 (5) |
14 (2) |
(*) Carbon dioxide is a product (C28) |
13 (6) |
156 (76) |
93 (46) |
262 (43) |
Carbon trioxide is a product (C29) |
|
1 (<1) |
3 (1) |
4 (1) |
The product is a different gas than carbon dioxide (C30) |
21 (10) |
16 (8) |
73 (36) |
110 (18) |
Prerequisites for the combustion |
The flame/annealing is a prerequisite for the combustion (C41) |
1 (<1) |
|
1 (<1) |
2 (<1) |
(*) The reaction requires (activation) energy (C22b) |
1 (<1) |
36 (18) |
4 (2) |
41 (7) |
Anthropomorphic and animistic conceptions |
Causes are anthropomorphized (C50) |
|
|
1 (<1) |
1 (<1) |
Table 5 Distribution of the CP solutions based on the IP overall and based on no (0) only one (1), two (2) or all three open-ended tasks of the CP could be characterized as one-sided, two-sided adoption; mixed or novel solution
|
|
One-sided adoption % |
Two-sided adoption % |
Mixed adoption % |
Novel solution % |
Overall |
|
43 |
15 |
10 |
33 |
Total number of tasks per group |
0 |
24 |
66 |
76 |
32 |
|
1 |
28 |
15 |
10 |
35 |
|
2 |
34 |
10 |
3 |
22 |
|
3 |
7 |
1 |
3 |
34 |
Table 4 also indicates that 15 conceptions, including scientifically adequate (e.g. C9) were activated in all three tasks in the IP and CP products. From the five categories of conceptions mainly the three potential mass change during the experiment, process of combustion, and potential reactants and products were addressed. Compared to this, fewer conceptions from the other two categories prerequisites for the combustion and anthropomorphic and animistic conceptions were identified.
RQ 2: differences between IP and CP worksheets
The second research question asks for differences between the IP and CP worksheets. We answer this in three steps following the coding Schemes 2–4:
• The IP and CP worksheets of each group are compared using coding Scheme 2 to gain information about the potential origin of the group results (see Table 2).
• The IP worksheets and the corresponding CP worksheets are compared using coding Scheme 3 to gather information about the potential development of the scientific accuracy of the learners' answers (see Table 3).
• The levels of representation in tasks 2 and 3 are compared using coding Scheme 4 again to gather information about the potential development in favour of scientifically adequate representations.
Scheme 2 Comparing IP and CP: Using coding Scheme 2, all four categories were identified (see Table 5). Overall, about 43% of the CP was based on an individual solution. A notable amount of 33 percent of the tasks was to be characterized as novel solutions, containing elements that were not found in any of the individual answers. 15% of both IP tasks were similar and did not change in the CP. The table also shows the distribution over the amount of the open-ended tasks (from 0 to 3). Accordingly, only about 7% adopted a complete worksheet from one of the group members.
Table 6 show the distribution between the three tasks. The analysis of possible connections between the type of adoption over the different tasks showed in all cases weak or moderate effect sizes (T1b–T2: Cramér's V = 0.28, p = 0.096; T1b–T3: Cramér's V = 0.35, p = 0.003; T2–T3: Cramér's V = 0.31, p = 0.024).
Table 6 Distribution of the CP solutions based on the IP between the open-ended tasks
Task |
One-sided adoption % |
Two-sided adoption % |
Mixed adoption % |
Novel solution % |
1b |
41 |
14 |
6 |
39 |
2 |
48 |
21 |
9 |
21 |
3 |
39 |
10 |
13 |
37 |
Table 7 Distribution of the scientific accuracy of students’ statements in all tasks and regarding IP and CP. Codes in brackets. For task 1a code 0 means false answer, code 1 correct answer
Tasks |
Increase of mass % (0) |
Constant mass % (1) |
Decrease of mass % (0) |
1a |
IP |
5 |
55 |
40 |
CP |
0 |
69 |
31 |
Tasks |
Inadequate % (0) |
Potentially adequate % (1) |
Adequate % (2) |
1b |
IP |
65 |
30 |
5 |
CP |
62 |
35 |
3 |
2 |
IP |
26 |
65 |
8 |
CP |
13 |
62 |
25 |
3 |
IP |
63 |
13 |
23 |
CP |
50 |
14 |
36 |
Total |
IP |
52 |
35 |
12 |
CP |
42 |
36 |
21 |
Table 8 Distribution of the representational level of students’ externalisations in task 2 and 3, regarding IP and CP. Codes in brackets
Task |
Usage of |
IP % |
CP % |
2 |
Word equation (0) |
60 |
43 |
Mixture of word and symbol equation (1) |
8 |
10 |
Symbol (chemical) equation (2) |
32 |
48 |
3 |
Macroscopic elements (0) |
14 |
6 |
Mixture of macro- and submicroscopic elements (1) |
20 |
14 |
Submicroscopic elements (2) |
67 |
80 |
Scheme 3 Scientific accuracy: The coded answers from IP were compared with those from CP using the Wilcoxon test to analyse a possible development of the scientific accuracy. Table 7 shows the percentage of the coding about the four tasks (the multiple-choice task 1a included). Three of the four tasks (1a, 2 and 3) showed a change in favour of (potentially) adequate answers. Regarding the high percentage of already correct answers in 1a of the IP, it has to be emphasised that the students already knew the context from classroom practice before they answered the worksheets. In contrast to this, the number of inadequate explanations (1b) and also the distribution in all other tasks still revealed uncertainty. They were inadequate or incomplete in a majority even after the CP. In 1b, only 3% of the explanations were adequate after the CP compared to 5.2% in the IP.
In comparison, the students improved on average in three of the four tasks. When tested by Wilcoxon test, the differences proved to be significant and, based on Cohen (2013), showed a large or medium effect size for the improvement ((1a) z = 3,48, p < 0.001, d = 0.62; (2) z = 4.85, p < 0.001, d = 1.01; (3) z = 2.98, p = 0.003, d = 0.54). There was no significant improvement for the task 1b.
Scheme 4 Usage of representational level: Additionally, task 2 and 3 were coded using Scheme 4 (levels of representation). We used a descriptive ranking from a macroscopic level towards a level that more and more integrates symbolic elements resp. refer to a submicroscopic level (Table 8). A Wilcoxon test indicated that in the collaborative phase the students significantly more frequently (a) used symbols in task 2 to describe the chemical reaction (z = 3.56, p < 0.001, d = 0.70) and (b) in the drawing-task 3 referred to the submicroscopic level (z = 3.63, p < 0.001, d = 0.68). But still about 41 percent of the answers in task 2 is based on a word equation. In contrast, a majority of students already used submicroscopic elements in the IP for the drawing task 3.
Table 9 shows the solutions of one dyad, the individual and the collaborative worksheet results. This example illustrates the capability of the individual and the collaborative phase. Both students A and B incorrectly answered task 1 with arguments that supported their decision. Each drawing (task 3) was coherent with the reaction scheme (task 2). The representations of Student A were assigned to the macroscopic level and the ones from Student B to the submicroscopic level, both with deficits. Student A only referred to the visible product, which is consistent in the drawing on a macroscopic level. However, in the reaction scheme the gas as a reaction product should have been considered. Student B indicates carbon[mono]oxide as a product. The collaborative results showed improvements in all tasks: they correctly answered 1a with an improved but still not sufficient explanation. The reaction scheme and the drawing were on a submicroscopic level based on the IP results of Student A but now mostly correct.
Table 9 Individual (IP) and collaborative (CP) worksheet results of one dyad
|
Worksheet answers |
Translation |
IP |
|
“I think it will weigh less because, when coal and Oxygen reacts, another substance will be formed. As it is gaseous it will ‘float’ into the balloon and I think, a gaseous substance weights less.” |
Student A |
Task 1b |
Task 2 |
|
Coal + Oxygen → Ashes |
Task 3 |
|
(left) Before heating: (+Oxygen not visible), Coal |
(right) After heating: (ash particles) |
IP |
|
The flask weights more after the reaction, because by this process the coal binds with the oxygen, resp. oxidized. The substances form a new* substance that weights as much as the sum of both? *(gaseous) |
Student B |
Task 1b |
Task 2 |
|
C + O Heat CO |
Coal Oxygen → Carbonoxide |
Task 3 |
|
(left) Before heating: coal |
(right) After heating: coal, oxygen |
CP |
|
It will weigh the same, because the formed substance is gaseous (CO2) [because the coal reacts with the oxygen and binds resp. oxidized, a new (gaseous) substance is formed] |
Task 1b |
Task 2 |
|
(s)C + O(g) → CO2(g) |
(Carbon) + (Oxygen) Carbon dioxide |
Task 3 |
|
(left) Before heating: Oxygen, Coal |
(right) After heating: carbon dioxide, carbon, oxygen |
Discussion
The theoretical Part I of this paper states seven essential features for fostering conceptual understanding in a collaborative setting. On this basis, we introduce the PIM in Part II as one possible approach that combines individual and collaborative learning activities and designed to meet the essential features. Part III reports a study based on two research questions that serve to give empirical evidence to the assumption made in Part II. In the following final sections, we will discuss the results of the study to argue the potential of the PIM and comparable approaches without disregarding limitations, open questions or indications for further improvements.
RQ 1 asks how the PIM encourages students to externalize their individual conceptions.
• 60% (33 out of 55) of the learner conceptions (scientific adequate included) about combustion that is known by the literature were indicated in the students’ answers. Notably, only a set of three tasks that address one single phenomenon activated this amount of different learner conceptions. Although the 55 different conceptions were taken from studies that deal with the topic of combustion, these studies nevertheless contain different contexts. Since conceptions are highly contextual, it is quite likely that we do not find some of these here. Anyway, it was neither the goal of the study to activate all known 55 learner conceptions nor to find new ones. However, the more conceptions could be identified, the more likely we can say that each learner got the chance to become aware of his or her conceptions. According to this, the RQ1 could be regarded as answered highly satisfactory.
• In regard to the results, the two categories, anthropomorphic and animistic conceptions and especially prerequisites for the combustion, were not activated in the same manner as the other three categories. This lack can be explained with fact, that the task does not bring up the questions of when or why an object burns. For the future, another task could be developed that also activates these categories. Nevertheless, the results show that the task is in accordance to meet F1 (Becoming aware of one's own conceptions).
• In addition to the number of different learner conceptions, the students’ responses also varied trough all levels of representation in the different modes (text, equation and drawing). 15 of the 33 conceptions can be diagnosed in all three tasks. Still, some of the conceptions were only found in one of the tasks individually, which suggests, for this specific topic (combustion), the use of one of the three tasks solely seem to be less suitable for externalising a comprehensive conceptual understanding. As we already stated above it is to be considered that all students previously were taught about Boyle's flask phenomenon. One evidence is the high percentage of (potentially) adequate formulated reaction schemes on the symbolic level (task 2, e.g. see Table 9). However, regarding the more ill-structured students’ explanations of the schemes, it is not sure whether the students had understood the meaning behind the scheme or whether it was instead a reproduction of classroom practice (see also Nyachwaya et al., 2014). Following Nyachwaya et al. (2014), the achievement of teaching goals might rely on memorized algorithms but does not guarantee the development of common conceptual understanding. In conclusion (F2 Externalising individual ideas), the students need opportunities to externalise a conception in different tasks and in different modes of representations. Based on the findings of the study, Boyle's flask task in particular and the multimodal framework of the PIM, in general, meet this requirement.
RQ2 focusses on the effect of collaborative work and on how it is interconnected with the individual phase for conceptual development.
• The findings that only about 7% of the group work was identical to a single solution support the impact of collaboration. More than 90% of the CP worksheets differed in at least one of the tasks compared to the individual works. So, on deciding for a shared solution the externalizations of each group member were considered. And even the adoption of a task from one individual worksheet does not imply the absence of discursive processes. In the example presented in Table 9 the CP results of tasks 2 and 3 could be classified mainly as an adoption of the IP solutions from Student B. The improvements indicate a discursive process. So, given the fact, that the majority of the CP results improved in their accuracy it is likely that the students were actively involved in making a targeted decision for or against one of the two solutions (F4 Ensuring active involvement).
• Remarkably, an overall positive development was measured within three of the four tasks (1a, 2 and 3). The CP results show higher accuracy and an increase in expressing ideas on the submicroscopic level. Overall, these results support the assumption for successful conceptual development through collaboration (see also Slavin, 1996; Schraw et al., 2006) and give evidence that the IP serve as a measure for each learning partner to be aware of alternative solutions, to reflect on them and to decide for or against one of them (F5 Offering each learner opportunities to reflect on each other's conceptual understanding). Additionally, the overall percentage of 43 for mixed or novel solutions is an excellent indicator of decision-making processes (F6 Integrating decision-making processes).
• The congruency of the answers and drawings concerning the expected answers gave hints about the students’ situation model. There is little doubt that the introduction of the worksheet, in particular the picture of Boyles's flask, activated a situation similar to that when the phenomenon was taught in classroom practice (F3 Eliciting common situation models).
• Referring to the last essential feature F7 (Offering the teacher measures to monitor the learning process), the variety of artefacts produced in the individual and collaborative phase serve as a reflection on the success of classroom teaching and getting an insight about remaining possible learner conceptions. However, the teacher could link his or her next intervention to the potentially diverse understandings. The artefacts also could serve as a pool for contrasting different solutions in a further discursive process.
Limitations
The results of the study reported in Part III are based on worksheets data of one single PIM task and so causes limitations we will discuss in this section.
• Salomon and Perkins (1998) and van Boxtel et al. (2000) differ between the product-related impact of collaborative learning and the process-related effects with collaborative learning. Concerning this distinction, our study analysed the effects of collaborative learning based on the exercise sheets of the students' reflection and peer collaboration. We already mentioned at the beginning of Part III that additional insights on the role of the discursive processes for conceptual development and evidence for F3-6 could be achieved by analysing the video- or audio-taped conversations of the students. In the outlook part, we will discuss further the potential of analysing the process data.
• The worksheet has three tasks that ask for different representational modes, which implies being a very pre-structured and guided instruction. Alternatively, the students could simply be prompted to answer the problem in various modes of representation. During the process of developing the worksheets, we had to balance the amount of instructional advice, not to run the risk of over-prompting. In further PIM-situations of one learning group, it might be a measure to provide worksheets with less structure or less instruction, just to fade-out the scaffold and promote long-life learning.
• As already mentioned, activating and developing learner conceptions could be highly context-specific (e.g.Boujaoude, 1991). The conclusions made here are based on a study about the context of combustion in a closed system. Just studying another combustion phenomenon like burning metal in an open system might activate new and/or contradictory conceptions. For indicating conceptual development, a coding manual was developed based on already published inadequate and scientifically adequate conceptions. Appendix 4 gives an overview of the documented conceptions about combustion. Although numerous studies have been searched for possible learners’ conceptions, there is no claim to completeness. However, the 55 conceptions grouped in the five categories advanced further research for the community in capturing the underlying concepts of the phenomenon combustion.
• Furthermore, in this study, we did not control or vary parameters like group composition or the use of the IP for the CP. For the latter, we argue with research evidence that the individual phase is essential for fruitful collaborative learning. Olsen et al. (2019) compared a combined condition to an individual or a collaborative-only learning condition and reported that the combined condition had higher learning gains than the other conditions. The students in collaborative learning situations reported higher situational interest in the activity. Also, the collaborative-only condition outperforms the individual only condition. Based on Olsen et al. (2019), it is still possible that the quality of the instruction on the worksheet allows the group to perform sufficiently even without individual reflection beforehand. Currently, we are running a study in a control condition to get more insights into the effectiveness and relevance of the IP.
Conclusion and outlook
This paper started with describing seven features, each of them essential for designing fruitful classroom practice to develop students’ conceptions as well as to present the Peer-Interaction-Method as a pre-structured pedagogical approach that is designed to meet these features. We will now conclude with pedagogical implications for chemistry classroom practice and then will proceed with an outlook for further research on this topic.
Implications for classroom practice
There are several pedagogical approaches like Think Pair Share (TPS) or Team Based-Learning (TBL) that use most of the seven essential features intuitively or explicitly based on theory or evidence (see Part II). In particular for customizing these approaches for chemistry classroom practice the essential features could serve as a useful framework. The PIM has similarities with the TBL approach (e.g.Michaelsen and Sweet, 2011) as it offers the teacher opportunities to form groups based on individual performances. The TBL collaborative phase serves to apply and deepen the knowledge acquired in the individual study phase, whereas the PIM CP brings up the same tasks of the IP. Therefore, the students will get no feedback on their performance from the teacher. We prefer to use the PIM at a peer-level. Students are often better able to express and discuss uncertainties when the presence of a teacher as an authority does not influence them (e.g.Boud and Walker, 1998; Topping, 2005). Here the PIM also differs from the TPS approach (e.g.Lyman, 1981; Raba, 2017). We recommend delaying, sharing and evaluating individual or collaborative products in the class in favour of an ongoing peer reflection process. We developed an additional part to the CP that asks the group to compare their work with a reference worksheet, consisting of different solutions based on scientifically accepted conceptions (Appendix 3). This process aims to encourage the groups of discussing unreproducible variations between the group work and the reference with the teacher or another authority that might be impediments of building up a coherent conceptual understanding. Based on the referenced worksheet the evaluation (or share) phase will still start on a peer level but also provide an efficient measure to the teacher for ensuring learning goals in classroom practice in terms that he or she could only ask the groups for discrepancies to be addressed in further activities.
Outlook for future research
Our study shows success concerning the goal of developing conceptual understanding. It also gives evidence on the relevance of the seven essential features. However, more research is needed to precise information about the role of, e.g., the quality of the individual artefacts in the discursive process. Referring to the example in Table 9 it could be of interest, how the students concluded in the CP for the concept of mass conservation based on their opposite IP solutions and also who came up with carbon dioxide as the reaction product. In addition to the question whether or in which way the collaborative learning phase fosters conceptual development, the process analysis could also be connected to other data like individual characteristics or group composition as possible promoting or hindering factors. Several studies, like in Le et al. (2018) show that collaborative skills have an impact on the outcome of collaborative processes. Kumpulainen and Kaartinen (2003) report that groups communicate with each other during collaboration not only through language but also through drawings. Concerning the group composition, the results of Le et al. (2018) show that friendship could be an obstacle to collaborative processes. We also choose dyads in our study. Other studies argue for groups with three or four (e.g.Järvelä et al., 2016) or even up to five students (e.g.Mason and Santi, 1998; Liu et al., 2018).
Until now, we generated data from students who use the PIM the first time and only once. Longitudinal studies are currently not available but could provide more information about a long-lasting effect. In this context, Tao and Gunstone (1999) reported a student's need to personally recognize his or her knowledge acquired in the group as meaningful. A pilot study reported in (Schanze and Busse, 2015) with groups using two worksheets based on the PIM in a row showed less regulative and more on-topic conversation parts in the second attempt.
In the frame of this study, we audio-recorded the discussions between the dyads. However, reporting results based on a qualitative data analysis (e.g. describing individual or group parameter as supporting or impeding factors based on contrasting cases) will go beyond the scope of this paper that at first will substantiate and report the educational benefit of the PIM or similar approaches. But it is planned in further publications.
We also developed and validated further worksheets based on PIM that address concepts like weak vs. strong acids or dynamic equilibrium. They will be used in further studies to generate more general results on the use of PIM as a collaborative setting to support conceptual understanding in chemistry classroom practice.
It is also an open question on our list, whether the PIM is a good measure to discuss the conceptual understanding of a phenomenon that was not yet taught in classroom practice. Providing the students with an initial learning situation might as well support productive failure (e.g.Kapur, 2016) when the students are aware of and discuss different explanations for the same problem in the CP.
Finally, the overall results of the study illustrate the relevance of the seven essential features for designing fruitful collaborative learning situations to develop conceptual understanding in classroom practice. As a traditional classroom practice has difficulty integrating all individual learner conceptions for developing a common and resilient conceptual understanding, similar approaches to the PIM could be substantiated and improved by taking these features into account.
Conflicts of interest
There are no conflicts to declare.
Appendix 1: structure of the PIM worksheets from the IP (page 1)
Appendix 1: structure of the PIM worksheets from the IP (page 2)
Appendix 2: first page of the PIM worksheets for the CP
Appendix 3: example of a reference worksheet (solutions can vary based on teachers’ individual classroom practice)
Appendix 4: 55 possible students’ conceptions regarding burning and combustion
Conception |
Ref. |
* marks the scientific adequate conceptions. |
Potential mass change during the experiment |
C1 |
Submicroscopic entities (e.g. atoms) are destroyed/macroscopic entities (e.g. substances) are destroyed without mentioning the emergence of new entities |
Barker and Millar (2000), Boujaoude (1991), Haupt (1984), Heimann and Harsch (2007), Hundertmark and Schanze (2017), Johannsmeyer et al. (2003), Özmen and Ayas (2003), Petermann et al. (2008), Pfundt (1975), Prieto et al. (1992), Ross (1991), Schollum and Happs (1982) and Watson et al. (1997) |
C2 |
Without exception, all submicroscopic entities (e.g. atoms) are destroyed/without exception, all macroscopic entities (e.g. substances) are destroyed without mentioning the emergence of new entities |
Pfundt (1975)
|
C3 |
Gases weigh less than solids |
Barker and Millar (2000), Boujaoude (1991), Hundertmark and Schanze (2017), Johannsmeyer et al. (2003), Kirbulut and Geban (2014), Özmen and Ayas (2003), Petermann et al. (2008), Valanides et al. (2003) and Watson et al. (1995) |
C4 |
Gases weigh nothing |
Johannsmeyer et al. (2003), Kirbulut and Geban (2014), Petermann et al. (2008) and Watson et al. (1995) |
C5 |
Due to the buoyancy of gases, the flask weighs less |
Based on Kirbulut and Geban (2014) |
C6 |
Due to another modification of the substance, the flask weighs less |
Barker and Millar (2000), Boujaoude (1991), Gabel et al., (2001), Haupt (1984), Hundertmark and Schanze (2017), Johannsmeyer et al. (2003) and Watson et al. (1995) |
C7 |
Due to another modification of the substance, the flask weighs more |
Chang et al. (2010) and Hundertmark and Schanze (2017) |
C8 |
A larger volume means a higher weight |
Johannsmeyer et al. (2003) and Prieto et al. (1992) |
C9 |
(*) The number of atoms remains the same in a chemical reaction |
Barker and Millar (2000), Heimann and Harsch (2002),Johannsmeyer et al. (2003) and Özmen and Ayas (2003) |
C10 |
(*) There is no mass transfer with the environment |
Barker and Millar (2000), Heimann and Harsch (2002), Johannsmeyer et al. (2003) and Özmen and Ayas (2003) |
Process of combustion |
C11 |
Reactants (e.g. coal) evaporate |
Barker and Millar (2000), Boujaoude (1991), Heimann and Harsch (2002, 2007), Hundertmark and Schanze (2017), Johannsmeyer et al. (2003), Meheut et al. (1985), Petermann et al. (2008), Petermann et al. (2010), Ross (1991), Valanides et al. (2003) and Watson et al. (1997) |
C12 |
Reactants (e.g. coal) melt |
Haupt (1984), Hundertmark and Schanze (2017), Meheut et al. (1985), Prieto et al. (1992) and Watson et al. (1997) |
C13 |
Oxygen transform into another product (e.g. soot) |
Haupt (1984) and Watson et al. (1995) |
C14 |
The appearance or properties of some reactant have changed, the substance (e.g. coal) still exists |
Boujaoude (1991), Chang et al. (2010), Heimann and Harsch (2007), Hundertmark and Schanze (2017), Meheut et al. (1985), Pfundt (1975), Prieto et al. (1992), Valanides et al. (2003) and Watson et al. (1997) |
C15a |
Coal atoms are destroyed/Coal is destroyed without mentioning the emergence of new substances |
Andersson (1990), Heimann and Harsch (2007), Hundertmark and Schanze (2017), Johannsmeyer et al. (2003) and Petermann et al. (2008) |
C15b |
(*) All carbon atoms are bound forming a new molecule/Coal reacts with mentioning the emergence of a new product |
Based on Wiberg et al. (2001) |
C16a |
Oxygen molecules are destroyed/Oxygen is destroyed without mentioning the emergence of new substances |
Heimann and Harsch (2007), Johannsmeyer et al. (2003), Meheut et al. (1985), Petermann et al. (2008), Petermann et al. (2010) and Watson et al. (1995) |
C16b |
(*) All oxygen atoms are bound forming a new molecule/Oxygen reacts with mentioning the emergence of a new product |
Based on Wiberg et al. (2001) |
C17 |
Combustion is an irreversible process. The reactants cannot be recovered. |
Pfundt (1975), Prieto et al. (1992) and Watson et al. (1995 (1997) |
C18a |
Reactants transform into new substances |
Andersson (1990), Boujaoude (1991) and Prieto et al. (1992) |
C19 |
Reactants (e.g. coal) dissolves and there is no substance left |
Boujaoude (1991) and Özmen and Ayas (2003) |
C20 |
Air transform into carbon dioxide |
Based on Prieto et al. (1992) and Watson et al. (1995, 1997) |
C21 |
Reactants transform into Energy/light/heat |
Andersson (1990), Barke (2006), Barker and Millar (2000), Prieto et al. (1992) and Ross (1991) |
C22a |
Energy/light/heat transforms into a substance, which can transform into other substances afterwards |
Meheut et al. (1985)
|
C32 |
(*) Coal atoms reacts with oxygen molecules to form carbon dioxide molecules/Coal reacts with oxygen to form carbon dioxide |
Binnewies et al. (2016), Petermann et al. (2008) and Wiberg et al. (2001) |
C33 |
Coal atom reacts with air molecules/Coal reacts with the air |
Based on Gabel et al. (2001) |
C34 |
Oxygen serve as a catalyst and speed up the reaction |
Freienberg et al. (2007) and Haupt (1984) |
C35 |
Coal atoms transform into carbon dioxide molecules/Coal transform into carbon dioxide |
Based on Watson et al. (1997) |
C36a |
The product is created by mixing a substance with the oxygen instead of a chemical reaction |
Hundertmark and Schanze (2017), Meheut et al. (1985), Pfundt (1975), Prieto et al. (1992), Valanides et al. (2003) and Watson et al. (1995) |
C36b |
(*) The product (e.g. carbon dioxide) mixes with the remaining oxygen |
Based on Wiberg et al. (2001) |
C37 |
Oxygen reacts with a substance other than coal to form a substance |
Based on Watson et al. (1995) |
C38 |
Oxygen molecule transform into carbon dioxide molecules/Oxygen transform into carbon dioxide |
Based on Prieto et al. (1992) |
C39 |
The hydrogen atoms of a substance (e.g. from the air) combine with the oxygen atoms to form water molecules |
Ross (1991)
|
C40 |
Substances (e.g. coal) contain the product (e.g. carbon dioxide), that is released |
Freienberg et al. (2007), Hundertmark and Schanze (2017), Prieto et al. (1992) and Watson et al. (1995, 1997) |
Potential reactants and products |
C23 |
(*) An oxygen molecule consists of two oxygen atoms |
Wiberg et al. (2001)
|
C24 |
Air is a single substance (e.g. oxygen) |
Meheut et al. (1985)
|
C25 |
(*) Coal is made up of carbon atoms |
Wiberg et al. (2001)
|
C26 |
Ash is a product |
Boujaoude (1991), Haupt (1984),Prieto et al. (1992),Watson et al. (1995, 1997) |
C27 |
Carbon monoxide is the only product |
Andersson (1986)
|
C28 |
(*) Carbon dioxide is a product |
Binnewies et al. (2016) and Wiberg et al. (2001) |
C29 |
Carbon trioxide is a product |
Based on Andersson (1986) |
C30 |
The product is a different gas than carbon dioxide |
Haupt (1984) and Watson et al. (1997) |
C31 |
(*) Water is a product |
Meheut et al. (1985), Ross (1991) and Watson et al. (1997) |
Prerequisites for the combustion |
C22b |
(*) The reaction requires (activation) energy |
Wiberg et al. (2001)
|
C41 |
The flame/annealing is a prerequisite for the combustion |
Haupt (1984), Hundertmark and Schanze (2017), Prieto et al. (1992) and Watson et al. (1997) |
C42 |
(*) Oxygen is a prerequisite for the combustion |
Freienberg et al. (2007) and Gabel et al. (2001) |
C43 |
Due to the different amounts of energy substances can contain or store, processes of combustion can run to a different degree |
Freienberg et al. (2007), Hundertmark and Schanze (2017) |
C44 |
Intensity of combustion depends on the properties of the reactants (e.g. density, condition of aggregation) |
Chang et al. (2010), Freienberg et al. (2007) and Hundertmark and Schanze (2017) |
C45 |
Intensity of combustion depends on the amount of energy supplied |
Freienberg et al. (2007) and Hundertmark and Schanze (2017) |
C46 |
Intensity of combustion depends on the composition of the substances |
Freienberg et al. (2007)
|
C47 |
Intensity of combustion depends on the arrangement of the submicroscopic particles (e.g. atoms) |
Freienberg et al. (2007)
|
C48 |
Intensity of combustion depends on the properties of a substance |
Freienberg et al. (2007)
|
C49 |
Intensity of combustion depends on the reactivity of the reactants |
Freienberg et al. (2007)
|
Anthropomorphic and animistic conceptions |
C50 |
Causes are anthropomorphized (C50) |
Barke (2006), Boujaoude (1991) and Freienberg et al. (2007) |
C51 |
Flame as cause for the formation of the products is anthropomorphized (C51) |
Freienberg et al. (2007), Haupt (1984), Rahayu and Tytler (1999) and Watson et al. (1997) |
Acknowledgements
The authors would like to thank all students and teachers involved in the study for their contribution to this project. Also, we would like to thank the reviewers for the comprehensive and constructive comments that helped improving this article significantly.
References
- Ainsworth S., Prain V. and Tytler R., (2011), Drawing to Learn in Science, Science, 6046, 1096–1097.
- Akaygun S. and Jones L. L., (2014), Words or Pictures: a comparison of written and pictorial explanations of physical and chemical equilibria, Int. J. Sci. Educ., 36, 783–807.
- Alvarez-Bell R., Wirtz D. and Bian H., (2017), Identifying Keys to Success in Innovative Teaching: Student Engagement and Instructional Practices as Predictors of Student Learning in a Course Using a Team-Based Learning Approach, Teach. Learn. Inquiry: ISSOTL J., 5, 128–146.
- Amin T. G., (2009), Conceptual Metaphor Meets Conceptual Change, Hum. Dev., 52, 165–197.
- Anderson J. R., Greeno J. G., Reder L. M. and Simon H. A., (2000), Perspectives on Learning, Thinking, and Activity, Educ. Res., 29, 11–13.
- Andersson B., (1986), Pupils’ explanations of some aspects of chemical reactions, Sci. Educ., 70, 549–563.
- Andersson B., (1990), Pupils’ Conceptions of Matter and its Transformations (age 12–16), Stud. Sci. Educ., 18, 53–85.
- Aronson E., (1978), The jigsaw classroom, Sage.
- Artut P. D. and Tarim K., (2007), The effectiveness of Jigsaw II on prospective elementary school teachers, Asia-Pac. J. Teach. Educ., 35, 129–141.
- Bamiro A. O., (2015), Effects of Guided Discovery and Think-Pair-Share Strategies on Secondary School Students’ Achievement in Chemistry, SAGE Open, 5, 1–7.
- Barke H.-D., (2006), Chemiedidaktik: Diagnose und Korrektur von Schülervorstellungen, Berlin, Heidelberg: Springer.
- Barker V. and Millar R., (2000), Students’ reasoning about basic chemical thermodynamics and chemical bonding: What changes occur during a context-based post-16 chemistry course? Int. J. Sci. Educ., 22, 1171–1200.
- Bauer R., Ullmann M. and Baumgartner P., (2013), Think – Write – Pair – Share: Der Writers’ Workshop als Learning-Ressource beim Verfassen von Qualifizierungsarbeiten, in Arnold R. and Lermen M. (ed.), Independent Learning: Die Idee und ihre Umsetzung, Hohengehren: Schneider Verlag, pp. 69–82.
- Berger R. and Hänze M., (2009), Comparison of two small-group learning methods in 12th-grade physics classes focusing on intrinsic motivation and academic performance, Int. J. Sci. Educ., 31, 1511–1527.
- Binnewies M., Finze M., Jäckel M., Schmidt P., Willner H. and Rayner-Canham G., (2016), Allgemeine und Anorganische Chemie, Berlin, Heidelberg: Springer.
- Boud D. and Walker D., (1998), Promoting Reflection in Professional Courses: The challenge of context, Stud. High. Educ., 23(2), 191–206.
- Boujaoude S. B., (1991), A study of the nature of students’ understandings about the concept of burning, J. Res. Sci. Teach., 28, 689–704.
- Caravita S. and Halldén O., (1994), Re-framing the problem of conceptual change, Learn. Instruct., 4, 89–111.
- Carey S., (1985), Conceptual change in childhood, MIT Press.
- Celikten O., Ipekcioglu S., Ertepinar H. and Geban O., (2012), The effect of the conceptual change oriented instruction through cooperative learning on 4th grade students’ understanding of earth and sky concepts, Sci. Educ. Int., 23, 84–96.
- Chang J.-M., Lee H. and Yen C.-F., (2010), Alternative conceptions about burning held by Atayal indigene students in Taiwan, Int. J. Sci. Math. Educ., 8, 911–935.
- Cohen J., (2013), Statistical Power Analysis for the Behavioural Sciences, Burlington: Elsevier Science.
- Crone T. S. and Portillo M. C., (2013), Jigsaw Variations and Attitudes About Learning and the Self in Cognitive Psychology, Teach. Psychol., 40, 246–251.
- Davidowitz B., Chittleborough G. and Murray E., (2010), Student-generated submicro diagrams: a useful tool for teaching and learning chemical equations and stoichiometry, Chem. Educ. Res. Pract., 11, 154–164.
- Davis E. A., (2003), Prompting Middle School Science Students for Productive Reflection: Generic and Directed Prompts, J. Learn. Sci., 12, 91–142.
- Devetak I., Vogrinc J. and Glažar S. A., (2009), Assessing 16-Year-Old Students’ Understanding of Aqueous Solution at Submicroscopic Level, Res. Sci. Educ., 39, 157–179.
- Dillenbourg P., (1999), What do you mean by collaborative learning? in Dillenbourg P. (ed.), Collaborative Learning: Cognitive and Computational Approaches (Advances in learning and instruction series), Pergamon, pp. 1–19.
- Dillenbourg P., (2002), Over-scripting CSCL: the risks of blending collaborative learning with instructional design, in Kischner P. A. (ed.), Three worlds of CSCL: Can we support CSCL? Heerlen: Open Universiteit Nederland, pp. 61–91.
- DiSessa A. A., (2008), A Bird’2-Eye View of the “Pieces” vs. “Coherence” Controversy: (From the “Pieces” Side of the Fence), in Vosniadou S. (ed.), Educational psychology handbook series. International handbook of research on conceptual change, New York: Routledge, pp. 35–60.
- Donovan M. S., Bransford J. and Pellegrino J. W., (1999), How people learn: bridging research and practice, Washington, DC: National Academy Press.
- Doymus K., Karacop A. and Simsek U., (2010), Effects of jigsaw and animation techniques on students’ understanding of concepts and subjects in electrochemistry, Educ. Technol. Res. Dev., 58, 671–691.
- Driver R., (1981), Pupils’ Alternative Frameworks in Science, Eur. J. Sci. Educ., 3, 93–101.
- Driver R., Asoko H., Leach J., Scott P. and Mortimer E., (1994), Constructing Scientific Knowledge in the Classroom, Educ. Res., 23, 5–12.
- Duit R., (2009), Bibliography – Students’ and Teachers’ Conceptions and Science Education.
- Duit R., Treagust D. F. and Mansfield H., (1996), Investigating Student Understanding as a Prerequisite to Improving Teaching and Learning in Science and Mathematics, in Treagust D. F., Duit R. and Fraser B. J. (ed.), Ways of knowing in science series. Improving teaching and learning in science and mathematics, New York: Teachers College Press, pp. 17–41.
- Duit R., Gropengießer H., Kattmann U., Komorek M. and Parchmann I., (2012), The Model of Educational Reconstruction – a Framework for Improving Teaching and Learning Science, in Jorde D. and Dillon J. (ed.), Science Education Research and Practice in Europe, Rotterdam: Sense Publishers, pp. 13–37.
- Duit R., Treagust D. F. and Widodo A., (2013), Teaching science for conceptual change, in Vosniadou S. (ed.), Educational Psychology Handbook. International Handbook of Research on Conceptual Change, Hoboken: Taylor & Francis, Ltd, 2nd edn, pp. 487–503.
- Eilks I., (2005), Experiences and reflections about teaching atomic structure in a jigsaw classroom in lower secondary school chemistry lessons, J. Chem. Educ., 82, 313–319.
- Evans H. G., Heyl D. L. and Liggit P., (2016), Team-Based
Learning, Faculty Research, and Grant Writing Bring Significant Learning Experiences to an Undergraduate Biochemistry Laboratory Course, J. Chem. Educ., 93, 1027–1033.
- Freienberg J., Kandt W., Schmidt M. and Parchmann I., (2007), Verbrennung verstehen – Vom Phänomen zum Basiskonzept der chemischen Reaktion, NiU - Chemie, 100/101, 70–75.
- Fischer F., Bruhn J., Gräsel C. and Mandl H., (2002), Fostering collaborative knowledge construction with visualization tools, Learn. Instr., 12, 213–232.
- Fischer F., Kollar L., Haake J. M. and Mandl H., (2007), Perspectives on collaboration scripts, in Fischer F. Kollar I., Mandl H. and Haake J. M. (ed.), Computer-supported collaborative learning: v. 6. Scripting computer-supported collaborative learning: Cognitive, computational and educational perspectives, New York: Springer, pp. 1–10.
- Gabel D. L., Stockton J. d., Monaghan D. L. and MaKinster J. G., (2001), Changing Children's Conceptions of Burning, Sch. Sci. Math., 101, 439–451.
- Gambari A. I. and Yusuf M. O., (2017), Relative Effectiveness of Computer-Supported Jigsaw II, STAD and TAI Cooperative Learning Strategies on Performance, Attitude, and Retention of Secondary School Students in Physics, J. Peer Learn., 10, 76–94.
- Garnett P. J., Garnett P. J. and Hackling M. W., (1995), Students’ Alternative Conceptions in Chemistry: A Review of Research and Implications for Teaching and Learning, Stud. Sci. Educ., 25, 69–96.
- Glaser R., (1991), The maturing of the relationship between the science of learning and cognition and educational practice, Learn. Instr., 1, 129–144.
- Gobert J., (2005), Leveraging Technology and Cognitive Theory on Visualization to Promote Students’ Science, in Gilbert J. K. (ed.), Visualization in Science Education, Dordrecht: Springer Netherlands, pp. 73–90.
- Gok T., (2018), The Evaluation of Conceptual Learning and Epistemological Beliefs on Physics Learning by Think-Pair-Share, J. Educ. Sci. Environ. Health, 4, 69–80.
- Gurel D. K., Eryilmaz A. and McDermott L. C., (2015) A Review and Comparison of Diagnostic Instruments to Identify Students’ Misconceptions in Science, Eurasia J. Math., Sci. Technol., 11, 989–1008.
- Haidar A. H., (1997), Prospective chemistry teachers’ conceptions of the conservation of matter and related concepts, J. Res. Sci. Teach., 34, 181–197.
- Hathorn L. G. and Ingram A. L., (2002), Cooperation and Collaboration Using Computer-Mediated Communication, J. Educ. Comput. Res., 26, 325–347.
- Haupt P., (1984), Verbrennungs- und Oxidationsvorgänge im Verständnis von Schülern, Köln: Aulis Verlag.
- Heimann R. and Harsch G., (2002), Schülervorstellungen zur Verbrennung von Kohlenstoff im geschlossenen System, UC, 72, 51–53.
- Heimann R. and Harsch G., (2007), Die Chemische Reaktion im Chemieanfangsunterricht. Eine experimentelle Erarbeitung am Beispiel der Verbrennung, CHEMKON, 14, 75–83.
- Hidi S., Weiss J., Berndorff D. and Nolan J., (1998), The role of gender, instruction, and a cooperative learning technique in science education across formal and informal settings, in L. Hoffmann, A. Krapp, K. A. Renninger and J. Baumert (ed.), Interest and learning (215–227), Kiel, Germany: IPN.
- Hundertmark S. and Schanze S., (2017), Was wird bei Verbrennungen vernichtet? Unt. Chem., 159, 19–25.
- Järvelä S., Järvenoja H., Malmberg J., Isohätälä J. and Sobocinski M., (2016), How do types of interaction and phases of self-regulated learning set a stage for collaborative engagement?, Learn. Instruct., 43, 39–51.
- Jeong H. and Chi M. T. H., (1997), Construction of Shared Knowledge During Collaborative Learning, in International Society of the Learning Sciences (ed.), Proceedings of the 2nd international conference on Computer support for collaborative learning, pp. 130–134.
- Johannsmeyer F., Schneider J. and Oetken M., (2003), Schülervorstellungen zum Boyle-Versuch, CHEMKON, 10, 73–74.
- Johnstone A. H., (1991), Why is science difficult to learn? Things are seldom what they seem, J. Comput. Assist. Learn., 7, 75–83.
- Kaddoura M., (2013), Think Pair Share: A teaching Learning Strategy to Enhance Students’ Critical Thinking, Educ. Res. Q., 36, 3–24.
- Kapur M., (2016), Examining Productive Failure, Productive Success, Unproductive Failure, and Unproductive Success in Learning, Educ. Psychol., 51, 289–299.
- Kirbulut Z. D. and Geban O., (2014), Using Three-Tier Diagnostic Test to Assess Students’ Misconceptions of States of Matter, Eurasia J. Math., Sci Tech., 10, 509–521.
- Knox K. J., Gillis E. A. L. and Dake G. R., (2019), A positive student experience of collaborative project work in upper-year undergraduate chemistry, Chem. Educ. Res. Pract., 20, 340–357.
- Kollar I., Fischer F. and Hesse F. W., (2006), Collaboration Scripts – A Conceptual Analysis, Educ. Psychol. Rev., 18, 159–185.
- Köse S., (2008), Diagnosing Student Misconceptions: Using Drawings as a Research Method, World Appl. Sci. J., 3, 283–293.
- Kumpulainen K. and Kaartinen S., (2003), The Interpersonal Dynamics of Collaborative Reasoning in Peer Interactive Dyads, J. Exp. Educ., 71, 333–370.
- Land S. M. and Zembal-Saul C., (2003), Scaffolding reflection and articulation of scientific explanations in a data-rich, project-based learning environment: an investigation of progress portfolio, Educ. Tech. Res. Dev., 51, 65–84.
- Le H., Janssen J. and Wubbels T., (2018), Collaborative learning practices: teacher and student perceived obstacles to effective student collaboration, Camb. J. Educ., 48, 103–122.
- Lee C.-Q. and She H.-C., (2010), Facilitating Students’ Conceptual Change and Scientific Reasoning Involving the Unit of Combustion, Res. in Sci. Educ., 40, 479–504.
- Lehtinen E., Hakkarainen K., Lipponen L., Rahikainen M. and Muukkonen H., (1999), Computer supported collaborative learning: a review, JHGI Giesbers reports on education, 10, Nehterlands: University of Nijmegen.
- Leppävirta J., (2012), The effect of naïve ideas on students’ reasoning about electricity and magnetism, Res. Sci. Educ., 42, 753–767.
- Linn M. C., (2000), Designing the Knowledge Integration Environment, Int. J. Sci. Educ., 22, 781–796.
- Liu Q.-T., Liu B.-W. and Lin Y.-R., (2018), The influence of prior knowledge and collaborative online learning environment on students’ argumentation in descriptive and theoretical scientific concept, Int. J. Sci. Educ., 43, 1–23.
- Lyman F., (1981), The Responsive Classroom Discussion, in Anderson A. S. (ed.), Mainstreaming Digest, College Park, MD: University of Maryland College of Education, pp. 109–113.
- Mason L. and Santi M., (1998), Discussing the Greenhouse Effect: children's collaborative discourse reasoning and conceptual change, Environ. Educ. Res., 4, 67–85.
- Meheut M., Saltiel E. and Tiberghien A., (1985), Pupils’ (II – 12 year olds) conceptions of combustion, Eur. J. Sci. Educ., 7, 83–93.
- Mercer N., (1995), The guided construction of knowledge: talk amongst teachers and learners, Clevedon: Multilingual Matters.
- Mercer N., Wegerif R. and Dawes L., (1999), Children's Talk and the Development of Reasoning in the Classroom, Br. Educ. Res. J., 25, 95–111.
- Michaelsen L. K. and Sweet M., (2008), The essential elements of team-based learning, New Dir. Teach. Learn., 116, 7–27.
- Michaelsen L. K. and Sweet M., (2011), Team-Based Learning, New Dir. Teach. Learn., 128, 41–51.
- Morrison J. A. and Lederman N. G., (2003), Science teachers’ diagnosis and understanding of students’ preconceptions, Sci. Educ., 87, 849–867.
- Nyachwaya J. M., Mohamed A.-R., Roehrig G. H., Wood N. B., Kern A. L. and Schneider J. L., (2011), The development of an open-ended drawing tool: an alternative diagnostic tool for assessing students’ understanding of the particulate nature of matter, Chem. Educ. Res. Pract., 12, 121–132.
- Nyachwaya J. M., Warfa A.-R. M., Roehrig G. H. and Schneider J. L., (2014), College chemistry students’ use of memorized algorithms in chemical reactions, Chem. Educ. Res. Pract., 15, 81–93.
- Olsen J. K., Rummel N. and Aleven V., (2019), It is not either or: an initial investigation into combining collaborative and individual learning using an ITS, Int. J. Comput. Support. Collab. Learn., 3, 1–29.
- Özdemir G. and Clark D. B., (2007), An Overview of Conceptual Change Theories, Eurasia J. Math., Sci Tech., 3, 351–361.
- Özmen H. and Ayas A., (2003), Students’ difficulties in understanding of the conservation of matter in open and closed-system chemical reactions, Chem. Educ. Res. Pract., 4, 279–290.
- Perkins D. V. and Tagler M., (2011), Jigsaw classroom, in R. Miller and B. Rycek (ed.), Promoting student engagement, Volume I: Programs, techniques, and Opportunities, Syracuse, NY: Society for the Teaching of Psychology, pp. 195–197.
- Petermann K., Friedrich J. and Oetken M., (2008), “Das an Schülervorstellungen orientierte Unterrichtsverfahren”: Inhaltliche Auseinandersetzung mit Schülervorstellungen im naturwissenschaftlichen Unterricht, CHEMKON, 15, 110–118.
- Petermann K., Friedrich J. and Oetken M., (2010), Diagnosetest: Zur Erhebung von Schülervorstellungen zum Themenfeld “Massenerhalt bei chemischen Reaktionen”, PdN – Chemie in der Schule, 59, 34–37.
- Pfundt H., (1975), Ursprüngliche Erklärungen der Schüler für chemische Vorgänge, MNU, 28, 157–162.
- Prieto T., Watson R. and Dillon J., (1992), Pupils’ understanding of combustion, Res. Sci. Educ., 22, 331–340.
- Raba A. A. A., (2017), The Influence of Think-Pair-Share (TPS) on Improving Students’ Oral Communication Skills in EFL Classrooms, Creative Educ., 8, 12–23.
- Rahayu S. and Tytler R., (1999), Progression in primary school children's conceptions of burning: Toward an understanding of the concept of substance, Res. Sci. Educ., 29, 295–312.
- Rau M. A., Bowman H. E. and Moore J. W., (2017), An adaptive collaboration script for learning with multiple visual representations in chemistry, Comput. Educ., 109, 38–55.
- Rennie L. J. and Jarvis T., (1995), Children's choice of drawings to communicate their ideas about technology, Res. Sci. Educ., 25, 239–252.
- Repice M. d., Keith Sawyer R., Hogrebe M. C., Brown P. L., Luesse S. B., Gealy D. J. and Frey R. F., (2016), Talking through the problems: a study of discourse in peer-led small groups, Chem. Educ. Res. Pract., 17, 555–568.
- Roschelle J., (1992), Learning by Collaborating: Convergent Conceptual Change, J. Learn. Sci., 2, 235–276.
- Roschelle J., (1996), Learning by collaborating: Convergent conceptual change, in Koschmann T. (ed.), Computers, cognition, and work. CSCL: Theory and practice of an emerging paradigm, Hillsdale, NJ, US: Lawrence Erlbaum Associates, Inc., pp. 209–248.
- Roschelle J. and Teasley S. D., (1995), The Construction of Shared Knowledge in Collaborative Problem Solving, in O’Malley C. (ed.), NATO ASI Series, Series F: Vol. 128. Computer Supported Collaborative Learning, Berlin, Heidelberg: Springer, vol. 13, pp. 69–97.
- Ross K., (1991), Burning: A Constructive Not a Destructive Process, Sch. Sci. Rev., 72, 39–49.
- Rotbain Y., Marbach-Ad G. and Stavy R., (2005), Understanding molecular genetics through a drawing-based activity, J. Biol. Educ., 39, 174–178.
- Saab N., van Joolingen W. R. and van Hout-Wolters B. H. A. M., (2007), Supporting Communication in a Collaborative Discovery Learning Environment: The Effect of Instruction, Instruct. Sci., 35, 73–98.
- Salomon G. and Perkins D. N., (1998), Chapter 1: Individual and Social Aspects of Learning, Rev. Res. Educ., 23, 1–24.
- Schanze S. and Busse M., (2015), Peer-Interaction: Förderung des Konzeptverständnisses durch ein kollaboratives Aufgabenformat, Unt. Chem., 149, 26–34.
- Schollum B. and Happs J. C., (1982), Learners’ views about burning, Australian Sci. Teach. J., 28, 84–88.
- Schraw G., Crippen K. J. and Hartley K., (2006), Promoting Self-Regulation in Science Education: Metacognition as Part of a Broader Perspective on Learning, Res. Sci. Educ., 36, 111–139.
- Slavin R. E., (1996), Research on Cooperative Learning and Achievement: What We Know, What We Need to Know, Contemp. Educ. Psychol., 21, 43–69.
- Stavy R., (1990), Pupils’ problems in understanding conservation of matter, Int. J. Sci. Educ., 12, 501–512.
- Stoyanova N. and Kommers P., (2002), Concept Mapping as a Medium of Shared Cognition in Computer-Supported Collaborative Problem Solving, J. Interact. Learn. Res., 13, 111–133.
- Swanson E., McCulley L. V., Osman D. J., Lewis N. S. and Solis M., (2019), The effect of team-based learning on content knowledge: a meta-analysis, Active Learn. High. Educ., 20, 39–50.
- Taber K. S., (2000), Multiple frameworks?: Evidence of manifold conceptions in individual cognitive structure, Int. J. Sci. Educ., 22, 399–417.
- Taber K. S., (2001a), Constructing chemical concepts in the classroom? Using research to inform practice, Chem. Educ. Res. Pract., 2, 43–51.
- Taber K. S., (2001b), The Mismatch between Assumed Prior Knowledge and the Learner's Conceptions: A typology of learning impediments, Educ. Stud., 27, 159–171.
- Taber K. S., (2009), Learning at the Symbolic Level, in Gilbert J. and Treagust D. F. (ed.), Models and modeling in science education: 4. Multiple representations in chemical
education, Dordrecht: Springer, pp. 75–105.
- Taber K. S., (2013), Revisiting the chemistry triplet: drawing upon the nature of chemical knowledge and the psychology of learning to inform chemistry education, Chem. Educ. Res. Pract., 14, 156–168.
- Taber K. S., (2014), Ethical considerations of chemistry education research involving ‘human subjects’, Chem. Educ. Res. Pract., 15(2), 109–113.
- Taber K. S., (2019), Alternative Conceptions and the Learning of Chemistry, Israel J. Chem., 59, 450–469.
- Taber K. S. and Bricheno P., (2009), Coordinating Procedural and Conceptual Knowledge to Make Sense of Word Equations: Understanding the complexity of a ‘simple’ completion task at the learner's resolution, Int. J. Sci. Educ., 31, 2021–2055.
- Tao P.-K. and Gunstone R. F., (1999), Conceptual change in science through collaborative learning at the computer, Int. J. Sci. Educ., 21, 39–57.
- Thomaz M. F., Malaquias I. M., Valente M. C. and Antunes M. J., (1995), An attempt to overcome alternative conceptions related to heat and temperature, Phys. Educ., 30, 19–26.
- Topping K. J., (2005), Trends in peer learning, Educ. Psychol., 25(6), 631–645.
- Treagust D. F., (1988), Development and use of diagnostic tests to evaluate students’ misconceptions in science, Int. J. Sci. Educ., 10, 159–169.
- Treagust D. F., (2006), Diagnostic assessment in science as a means to improving teaching, learning and retention, in Proceedings of The Australian Conference on Science and Mathematics Education (formerly UniServe Science Conference).
- Treagust D. F., Duit R. and Nieswandt M., (2000), Sources of students’ difficulties in learning chemistry, Educ. Quím., 11, 228–235.
- Treagust D. F., Chittleborough G. and Mamiala T., (2003), The role of submicroscopic and symbolic representations in chemical explanations, Int. J. Sci. Educ., 25, 1353–1368.
- Tversky B., (2001), Spatial schemas in depictions, in Grialou P., Longo G. and Okado M. (ed.), Spatial schemas and abstract thought, Cambridge: MIT Press, pp. 79–112.
- Valanides N., Nicolaidou A. and Eilks I., (2003), Twelfth grade students’ understanding of oxidation and combustion: using action research to improve teachers’ practical knowledge and teaching practice, Res. Sci. Technol. Educ., 21, 159–175.
- Van Boxtel C., van der Linden J. and Kanselaar G., (2000), Collaborative learning tasks and the elaboration of conceptual knowledge, Learn. Instr., 10, 311–330.
- Vosniadou S., (2013), Conceptual change in learning and instruction: The framework theory approach, in Vosniadou S. (ed.), Educational Psychology Handbook. International Handbook of Research on Conceptual Change, Hoboken: Taylor & Francis, Ltd, 2nd edn, pp. 11–30.
- Vosniadou S. and Brewer W. F., (1987), Theories of knowledge restructuring in development, Rev. Educ. Res., 57, 51–67.
- Wandersee J., Mintzes J. J. and Novak J. D., (1994), Research on alternative conceptions in science, in Gabel D. L. (ed.), Handbook of research on science teaching and learning: A project of the National Science Teachers Association, New York, NY: Macmillan, pp. 177–210.
- Wang X. and Mu J., (2017), Introduction to Collaboration Scripts, in X. Wang and J. Mu (ed.), Perspectives on Rethinking and Reforming Education. Flexible Scripting to Facilitate Knowledge Construction in Computer-supported Collaborative Learning, Singapore: Springer Singapore, pp. 13–24.
- Watson R., Prieto T. and Dillon J. S., (1995), The effect of practical work on students’ understanding of combustion, J. Res. Sci. Teach., 32, 487–502.
- Watson J. R., Prieto T. and Dillon J. S., (1997), Consistency of students’ explanations about combustion, Sci. Educ., 81, 425–443.
- Webb N. M., (1989), Peer interaction and learning in small groups, Int. J. Educ. Res., 13, 21–39.
- Wegerif R. and Mercer N., (1997), A Dialogical Framework for Investigating Talk, in Wegerif R. and Scrimshaw P. (ed.), The language and education library: Vol. 12. Computers and talk in the primary classroom, Clevedon: Multilingual Matters, pp. 49–65.
- Weinberger A., Stegmann K. and Fischer F., (2007), Knowledge convergence in collaborative learning: concepts and assessment, Learn. Instruct., 17, 416–426.
- Wiberg E., Wiberg N. and Holleman A. F., (2001), Inorganic chemistry (1st English ed.), San Diego, Berlin, New York: Academic Press; De Gruyter.
- Wiegant F., Boonstra J., Peeters A. and Scager K., (2012), Team-based learning in honors science education: the benefit of complex writing assignments, J. Nat. Collegiate Honors Council, 13, 219–227.
- Wu S. P. W. and Rau M. A., (2019), How Students Learn Content in Science, Technology, Engineering, and Mathematics (STEM) Through Drawing Activities, Educ. Psychol. Rev., 31, 87–120.
- Yan F. and Talanquer V., (2015), Students’ ideas about how and why chemical reactions happen: mapping the conceptual landscape, Int. J. Sci. Educ., 37, 3066–3092.
|
This journal is © The Royal Society of Chemistry 2020 |
Click here to see how this site uses Cookies. View our privacy policy here.