DOI:
10.1039/D0RA08767J
(Paper)
RSC Adv., 2020,
10, 44774-44784
Monohydrate and anhydrate of nylon 5I monomer 1,5-pentanediamine–isophthalate†
Received
15th October 2020
, Accepted 11th November 2020
First published on 17th December 2020
Abstract
Nylon 5I is one of the new bio-based nylon materials. Its raw material 1,5-pentanediamine (PDA) is prepared by biological methods using biomass as the raw material. The high-performance polymer materials require the original high-quality monomers. 1,5-Pentanediamine–isophthalate (PDA–IPA) was taken as the direct monomer for the preparation of nylon 5I, and the crystallization was a valuable and essential approach to preparing the good-performance monomer salt. In this report, we found and obtained two crystal forms of PDA–IPA, monohydrate and an anhydrous form. Their crystal structures were determined and analyzed by single crystal X-ray diffraction (SCXRD), powder X-ray diffraction (PXRD), and Fourier transform infrared spectroscopy (FTIR). Hirshfeld surface maps were employed to capture the differences in the interactions present in the two forms. The thermal behaviors were characterized by differential scanning calorimetry (DSC) and thermogravimetric analysis (TGA). Moreover, the monohydrate and anhydrous phase can transform to each other through solid–solid transformation or solution-mediated phase transformation, and the critical values of the phase transformation were determined. Finally, the relative stability of the two forms under different thermodynamic conditions was discussed, especially the influence of temperature and water activity on the stability.
Introduction
Nylon, also called polyamide (PA), is the general name of thermoplastic resins containing repeating amide groups on the molecular backbone, with excellent mechanical properties, such as friction resistance, self-lubricity, acid and alkali resistance. With the increasing requirements for plastic materials in the fields of electronics, automobiles, aerospace, and chemical industry, the market demand for high-temperature resistant nylon with superb performance has expanded dramatically.1 High melting temperature and heat resistance are the main advantages of semi-aromatic polyamides, such as PA4T/4I/6T, which has low cost and moderate water absorption rate, and has been brought on the market as the high-temperature resistant nylon material.2–4 However, the extraction and production of their raw materials involve related toxic chemical substances, which has caused serious damage and pollution to the environment.5,6
In recent years, new bio-based nylons based on crops and other biomass resources have become a new research hotspot in the nylon industry due to its consistency with the national sustainable development concept.7 Since the raw materials can achieve sustainability in the production and preparation process, it is considered to be a new trend leading the direction of future material development.8 The bio-nylon series based on 1,5-pentanediamine (PDA) is opening up a green route for nylon production attributed to its good industry applications.9,10 Nylon 5I, which is polymerized by 1,5-pentanediamine–isophthalate (herein after referred to as PDA–IPA, see Fig. 1, C13H20N2O4, MW 268.33 g mol−1), is considered to be a potential substitute of the traditional nylon 4I/4T/6T for its high-temperature resistance. As we all know, high-quality monomers are essential to synthesize excellent performance polymers, and the crystallization was taken as the main method for the preparation and purification of monomers.11,12 During our research, we found that PDA–IPA exists in two crystal forms, one contains crystal water and the other does not.
 |
| Fig. 1 Molecular structure of 1,5-pentanediamine–isophthalate. | |
It is common that compounds exists in both hydrate and anhydrous forms,13 especially for bio-chemicals involving water as the solvent in their preparation process.14 The hydrates of a compound may well have different physicochemical properties compared to its parent anhydrate forms, such as solubility, density, hygroscopicity, dissolution rate, chemical stability, and mechanical property.15–20 Therefore, investigating the hydration state, possible transformation pathways and mechanism under different conditions is very helpful to guarantee the product quality.21,22
Given that the transportation, use, and storage of the product were significantly influenced by the stability,23 it is necessary to understand the relative stability of different solid forms, especially to find the optimum crystal forms of the target compounds. Related studies on theophylline, ampicillin and naproxen sodium indicate that the water activity (aw) in the experimental system using mixed solvents is the key factor in determining the mutual transition between hydrate and anhydrate.24–26 For a given temperature, there exists a corresponding equilibrium value of water activity, the relative stability of the anhydrous and hydrated forms is consistent at this time. Besides, any fluctuation in the equilibrium water activity under the same conditions may lead to a phase transformation.
Solid-state transformations (SST)27–30 and solution-mediated polymorphic transformations (SMPT) are two common mechanism of the phase transformation.30–35 The SMPT process is more common than the former, mainly because most of the polymorphic conversion process occurs in the solvent systems. Slurry experiments are a special type in the SMPT category. Considering its simplicity and ease of operation, it is often used to screen the polymorphs of the crystal products and to evaluate the relative stability of different forms. Many research cases have shown that the water activity is the major factor in the hydration of the compound in the slurry experiments with water–solvent mixtures.24,36
In this research, the two crystalline powders of the PDA–IPA were characterized from their structures, morphology, stability, and thermal behaviors by SCXRD, PXRD, FTIR, and TGA-DSC. The differences in the crystal structure, hydrogen-bonding interactions, and molecular packing between the monohydrate and anhydrate have also been investigated based on the single crystal data and Hirshfeld surface analysis. Moreover, phase transformations between the two forms under different thermodynamic and humidity conditions were studied through the SST and SMPT experiments, and the transformation mechanism of the two solid forms was finally elucidated.
Experimental section
Materials
1,5-Pentanediamine (PDA, 99.5% purity, mp 180 °C, MW 102.18 g mol−1) was prepared by the biological method of L-lysine decarboxylation in our laboratory. Its purity and concentration were determined by high performance liquid chromatography (HPLC). Analytical-grade ethanol (EtOH, 99.5% purity, bp 78.3 °C, MW 46.07 g mol−1) was obtained from Shanghai Chemistry Reagent Co. (China). Isophthalic acid (IPA, 99.5% purity, mp 341 °C, MW 166.13 g mol−1) was purchased from Shanghai Macklin Biochemical Co., Ltd. Deionized water was obtained from an ultrapure water system (YPYD CO., China).
Preparation of the crystal forms
The PDA–IPA monohydrate was prepared by ethanol anti-solvent crystallization in aqueous solution. A total of 3.6 ± 0.1 g of the PDA was dissolved in 10 g of water in a 50 ml beaker first, and then poured into a 100 ml double-jacketed crystallizer at ambient temperature. The solution was agitated by a stirrer with three blades at 200 rpm (HD2015W, Sile Instrument, China). After complete mixing, 5.85 g IPA powder was added in batches into the aqueous solution of PDA, and was continuously stirred until the mixed solution became transparent. Ethanol was chosen as the anti-solvent, and was added to the mixed solution at a constant rate of 1.0 ml min−1 using a peristaltic pump (model BT100-1L, Baoding Longer, China). The total amount of the anti-solvent added was ten times that of the solvent. Precipitation gradually appeared with the addition of EtOH after about 20 min, and the system was kept stirring for 2 h to obtain crystals.
The anhydrous form of PDA–IPA was prepared by reaction crystallization. 3.6 ± 0.1 g of the PDA was completely dissolved in 50 ml of EtOH at room temperature. 100 ml of ethanol was poured into a 250 ml double-jacketed crystallizer at 45 °C (controlled by a temperature circulation pump, CK-4005GD, SCIENTZ). Then, 5.85 g of the IPA solid powder was slowly added to the crystallizer, and the solution was agitated at 200 rpm for 2 hours. The liquid was transparent after the IPA completely dissolved in EtOH, and then the mixed PDA–EtOH solution was slowly pumped into the jacket using a peristaltic pump at a constant rate of 0.8 ml min−1. The nucleation of the crystal was observed about 15 minutes after adding the PDA–EtOH. The system was held at a constant temperature, and the crystals were obtained after stirring for 2 hours.
After filtering, washing with EtOH, and air-drying in a vacuum oven at 40 °C for 12 hours, we finally obtained the two crystalline products. The structures of the sample were identified by PXRD for consistency.
Transformation experiments
SST process between the monohydrate and anhydrate. The conversion relationship of the two crystal forms under different thermal and humidity conditions was studied. The first factor investigated was humidity. About 200 mg of crystalline powder was taken of the two forms, and placed in Petri dishes with a 5 cm diameter of varying relative humidity (RH = ∼0%, 32%, 43%, 67%, 76%, 98%).37 After being placed at room temperature for 6 weeks, the samples were taken out and characterized by PXRD. Meanwhile, we also investigated the hygroscopicity of the two crystal forms by tracing the mass variation of the samples subjected to different humidity circumstances. Temperature was the second factor to be considered. About 200 mg powder of the two crystal forms was weighted in several dishes, stored at room temperature, 40 °C, 60 °C, 80 °C for one month, and at 120 °C for 15 h. Samples were also identified by PXRD after the test period.
SMPT process between the monohydrate and anhydrate. Five solvents (methanol, ethanol, water, water–2-propanol and water–ethanol) were used to screen for the PDA–IPA polymorphs and phase transformation. Saturated solutions of PDA–IPA were put in a 25 ml triangular conical flask, and stirred using a rotor at 500 rpm. An excessive amount of the two crystal forms of PDA–IPA powder was added to different mixed solvents, and agitated at certain temperatures for 2 weeks. The turbid liquid was filtered after the experiments. The obtained filter cake was dried, and then characterized by PXRD.
Slurry experiments. By slurrying the two crystalline powders in the water–ethanol binary solvents with various water activities, the critical water activity required for the hydration of the two forms was determined.38 Furthermore, when the experiments were performed at various temperatures, different critical water activities were obtained. In this work, the experimental temperatures were 293.15, 298.15, 303.15, 308.15 in 5 K increments to 343.15 ± 0.1 K, and the water activity was calculated by the Margules formula.24 A series of binary mixtures composed of water and ethanol (water mole fractions Xw = 0.02, 0.06, 0.1, 0.15, 0.2, 0.3 in 0.1 increments to 1.0) were prepared. The excess of the two crystalline powders was added into about 8 ml of the mixed solvents, and stirred well for 24 h to reach a solid–liquid two-phase equilibrium. After filtering the mixed solution, the wet cakes were taken out and identified by PXRD as well.
Single crystal X-ray diffraction (SCXRD)
A single crystal of the monohydrate was obtained in pure water by cooling crystallization, while the anhydrate was obtained in a pure ethanol solution by evaporation crystallization. The specific method of evaporation crystallization is to add a slight excess of crystalline powder of the anhydrate to a 20 ml beaker containing 15 ml water–ethanol binary solvent (95% wt ethanol content). After it was stirred thoroughly and the solid was completely dissolved, the beaker is placed into another 100 ml beaker filled with 30 ml DMF solvent. The large beaker was tightly sealed, and then the anhydrous single crystal slowly precipitated out after 1 week.
The single-crystal structures were determined on a Bruker SMART APEX diffractometer using Mo Kα radiation (λ = 0.71073 Å) with a graphite monochromator at room temperature. The Bruker SMART-1000 program was used for data collection. The integration and scaling of the intensity data were accomplished using the SAINT39 program. The structures were analyzed by direct methods, and refined by full matrix least-squares methods on F2.40 All of the hydrogen atoms were treated by a constrained refinement, including the ones connected to amidogen and carboxylic group, and were located in a difference Fourier map with bond-length restraints of N–H = 0.89(1) Å. All non-hydrogen atoms were refined with anisotropic thermal parameters, and calculations were implemented by the SHELXL-2018/3.41 The measurement details of the diffraction and refinements are shown in Table 1, and the data was processed using PLATON.42 MERCURY 3.3
43 was used for structure visualization, and the acquisition of standard diffraction patterns.
Table 1 Crystallographic data for the PDA–IPA monohydrate and anhydrous form
|
Monohydrate |
Anhydrate |
Empirical formula |
C13H20N2O4·H2O |
C13H20N2O4 |
Formula weight |
286.33 |
268.33 |
Crystal system |
Orthorhombic |
Monoclinic |
Space group |
Pbcn |
P21/n |
a (Å) |
10.9462(9) |
13.4758(12) |
b (Å) |
13.6297(11) |
11.6629(11) |
c (Å) |
19.4386(16) |
18.8817(16) |
α (deg) |
90 |
90 |
β (deg) |
90 |
103.974(4) |
γ (deg) |
90 |
90 |
Volume (Å3) |
2900.1(4) |
2879.8(4) |
Z |
8 |
8 |
Dcalc. (g cm−3) |
1.312 |
1.238 |
μ (mm−1) |
0.101 |
0.092 |
F (000) |
1232 |
1152 |
Crystal size (mm) |
0.15 × 0.27 × 0.40 |
0.10 × 0.30 × 0.37 |
Observed data [I > 2σ(I)] |
1764 |
2581 |
R(int) |
0.046 |
0.088 |
R1 [I > 2σ(I)] |
0.0397 |
0.0646 |
wR2 |
0.1104 |
0.1324 |
GOF on F2 |
1.02 |
1.03 |
Diff. density (e Å−3) |
−0.20, 0.16 |
−0.20, 0.23 |
CCDC |
1969111 |
1969113 |
Fourier transform infrared spectroscopy (FTIR)
Infrared spectra of the products were recorded on a FT-IR spectrometer (Nicolet iS5, Thermo Scientific, USA) with an ATR reflectance attachment. Spectra were collected in the wavenumber range of 4000–500 cm−1 with a resolution of 4 cm−1.
Powder X-ray diffraction (PXRD)
PXRD is an advantageous tool for the qualitative identification of crystalline powder. In this work, PXRD patterns were obtained using a Rigaku SmartLab diffractometer system using Cu Kα radiation (λ = 1.5406 Å). The intensity data were collected at 150 K. The sample was scanned within the 2θ range from 5° to 40° in continuous scan mode, with a rate of 10° min−1 and a step size of 0.02°. The experimental PXRD patterns of the samples were compared with those calculated from their single-crystal structures.
Thermogravimetry and differential scanning calorimetry (TGA-DSC)
TGA-DSC (NETZSCH STA 449 F3, NETZSCH, Germany) was conducted to characterize the thermal behaviors of the PDA–IPA monohydrate and anhydrous crystals. Approximately 20 mg of the sample was added to an alumina crucible, and purged with a stream of flowing nitrogen throughout the experiment at 40 ml min−1. The heating rate was 10 °C min−1 and the temperature range was between 25 and 500 °C.
Hirshfeld surface analysis (HSs)
Molecular HSs analysis44 and fingerprint plots of the two crystal forms of PDA–IPA were performed using the CrystalExplorer 17.5 software to gain further insight into the packing modes and intermolecular interactions in crystals.45,46
Results and discussion
PXRD analysis
The PDA–IPA hydrate and its anhydrous phase were characterized by PXRD to ensure phase identity (Fig. 2). The two crystal forms have their individual characteristic peaks: the peaks of the monohydrate were detected at 13.76°, 18.24°, 25.24° and 26.06° (2θ), while those of the anhydrate were located at 21.72°, 25.46° and 25.72° (2θ). The PXRD fingerprint of the two forms can be easily distinguished, and the experimental patterns coincide well with the simulated ones calculated from their single-crystal structures. In fact, it was found that it was also easy to distinguish them by their morphology: rod-like for the monohydrate and block-like for the anhydrate, as illustrated in Fig. 3.
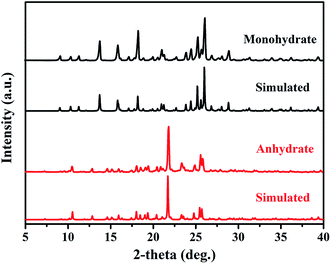 |
| Fig. 2 Comparison of powder X-ray diffraction (PXRD) patterns with the simulated patterns from single-crystal X-ray diffraction (SCXRD) for the monohydrate and anhydrate of PDA–IPA. | |
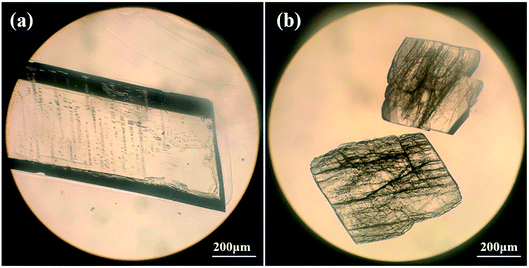 |
| Fig. 3 Optical micrographs of crystals obtained in experiments: (a) monohydrate; (b) anhydrous form. The magnifications are 16× 10. | |
Crystal structures analysis
Crystallographic details of the two crystal forms are listed in Table 1. There exists eight molecules in the unit cells for both crystal forms of PDA–IPA (Fig. S1 and S2†), and the PDA and IPA exist as a protonation and deprotonation state in the two crystal lattices, respectively. In fact, the proton transfer was completed in aqueous solution before the crystallization. Meanwhile, it was revealed that there are considerable structural differences between the two forms of PDA–IPA. The monohydrate is an orthorhombic system, Pbcn space group, while the anhydrate is monoclinic, P21/n space group. There exist eight molecules for both crystalline forms in the unit cell (Fig. S1 and S2†). The monohydrate contains one crystal water molecule, while the anhydrate does not. The crystal density of the monohydrate was 1.312 g cm−3, which is higher than that of the anhydrous form at 1.238 g cm−3.
Compared to the asymmetric unit in the crystal structure of the monohydrate, that of the anhydrous form contains a pair of PDA–IPA species. A huge twist of the single PDA molecule in the crystal lattice is a significant feature of the conformational difference between the two forms, reflecting the difference of the torsion angles of the PDA species: C11–C12–C13–N2 (−178.68°) for the monohydrate, in comparison with C19–C20–C21–N2 (−174.28°) and C23–C24–C25–C26 (66.05°) for the anhydrate (shown in Fig. 4a and b). The phenomenon was mainly caused by the different intermolecular interactions of the host molecules (see details of the hydrogen bonds data and torsion angles in Tables S1 and S2†). The carbon chain of another PDA species in the anhydrate shows a big twist, making it tightly connect to the adjacent IPA and PDA molecules. It should be pointed out that such conformation rotation never appeared in the nylon 54 salt\56 salt\5T salt (monomer) reported in our previous research.47–49
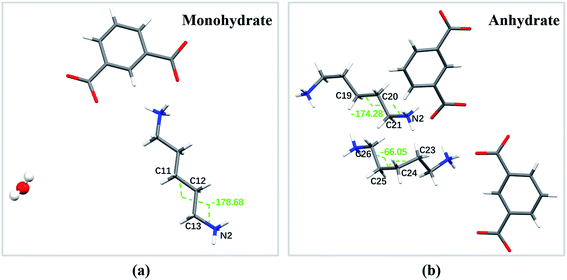 |
| Fig. 4 The conformational difference between the monohydrate (a) and anhydrate (b) of PDA–IPA. | |
The structures of the PDA–IPA monohydrate and anhydrate show different packing modes along the b axis, as shown in Fig. 5. There are two types of hydrogen-bond contact, N–H⋯O and O–H⋯O, in the monohydrate. In Fig. 5a, the H atom of the amino group of PDA is bonded with the O atom of the carboxyl group of IPA by hydrogen bond interaction. Moreover, the water molecule acts as a bridge to connect two adjacent IPA molecules that interact through individual carboxyl groups, which form a closed ring and is represented by the symbol R44(12). As for the anhydrous form (Fig. 5b), only the single N–H⋯O hydrogen-bond contact exists, and the stacking patterns of the crystal structure are mainly caused by the bridging of the two H atoms of the amino group of PDA and the O atoms on the neighboring two IPA carboxyl groups, which are represented by the symbols R44(8) and R44(24). In addition, from the perspective of the crystal density, it seems that the monohydrate is supposed to be more stable. To more intuitively describe the intermolecular interactions of the two crystal forms, further analysis by the Hirshfeld surface was carried out.50
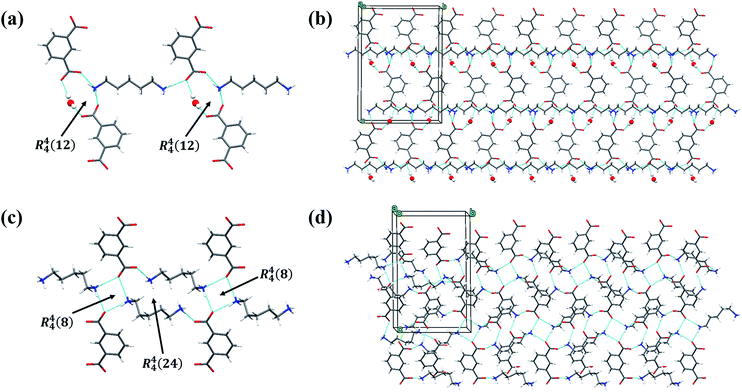 |
| Fig. 5 (a) 2D structure and (b) 3D network of the monohydrate, and (c) 2D structure and (d) 3D network of the anhydrous form. The blue dashed line denotes the hydrogen-bonding interactions. | |
Hirshfeld surface analysis
HSs analysis can be employed to visualize the molecular interactions, and identify the different interaction types between the two crystal forms. Associated 2D fingerprint plots were enabled to further quantify the level of contact interactions between the molecules by highlighting their particular close contacts.51
The HSs maps (Fig. S3 and S4†) show the intermolecular contacts as red-colored spots, which indicate the closer contacts of the N–H⋯O and O–H⋯O hydrogen bonds. The percentage contributions of these interactions are shown on a more quantitative basis, as displayed graphically in Fig. 6. We can see that the H⋯H and O⋯H contacts remarkably contribute the largest percentage (37.0–43.9%). In addition, the C⋯H interactions are another important contact, but they vary with the multicomponent specific crystal structure. The hydrogen-bond contacts that showed obvious red spots in HS were also analyzed, as shown in Fig. 7, S5 and S6.† As seen, the PDA–IPA molecules obviously show several red spots on the amino group in the anhydrate, corresponding to an amino–amino pair by N–H⋯O (Fig. 7b). Compared to the anhydrate, the red spots in the monohydrate are caused by the N–H⋯O and O–H⋯O hydrogen bonds together, which is due to the presence of water molecules in the crystal lattice (Fig. 7a).
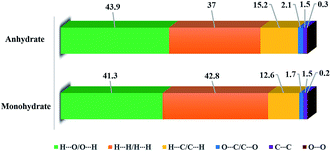 |
| Fig. 6 Percentage contributions to the Hirshfeld surface areas for the various close intermolecular contacts for the molecules in the two forms of PDA–IPA. | |
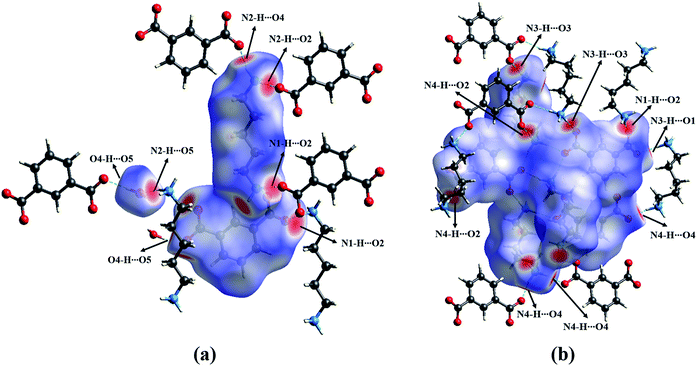 |
| Fig. 7 The Hirshfeld surface of the two forms of PDA–IPA mapped over dnorm in the color range −0.7263 to 1.2573 a.u., showing the hydrogen-bond contacts with the N–H⋯O and O–H⋯O groups in the monohydrate (a) and only N–H⋯O in the anhydrate (b). | |
The HSs analysis showed the importance of H-atom contacts and the difference of hydrogen-bond contacts in constructing the spatial packing of the two forms. Numerous H⋯H, O⋯H, and C⋯H interactions suggested that van der Waals interactions and hydrogen bonding play the major roles in the crystal packing.
FTIR analysis
FT-IR spectroscopy can easily complete the identification of substances containing strong hydrogen bonds, such as the N–H⋯O and O–H⋯O hydrogen bonds.52 Fig. 8 illustrates the absorption infrared spectra of the two PDA–IPA crystal forms in the region of 550–4000 cm−1. The FTIR pattern of the anhydrate has one legible characteristic peak at 1557.72 cm−1, while the monohydrate has two characteristic peaks at 1561.25 and 1553.23 cm−1. These absorbing peaks were both taken as the interaction of the hydrogen bond between the secondary amine N–H of PDA and the C
O group of IPA. Furthermore, when compared to anhydrate, it can be observed that there are three extra bands at 3372.95 cm−1, 1658.54 cm−1, and 603.97 cm−1 of the monohydrate, which mainly correspond to the hydroxyl group stretching vibration of the water molecules.53 Thus, the FTIR pattern analysis can be a tool for the phase identification of two such crystal forms.
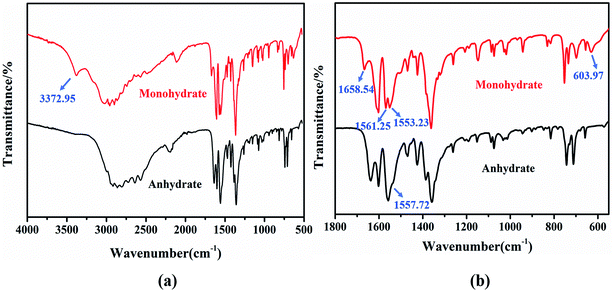 |
| Fig. 8 Comparison of the FTIR patterns of the PDA–IPA monohydrate and anhydrate. | |
Thermal analysis
To investigate the thermal stability variation of the two crystalline forms, TGA and DSC experiments were performed. The monohydrate shows a total mass loss of 6.60%, starting at approximately 122.5 °C in the DSC curve in Fig. 9a, which corresponds to just one crystal water loss (theoretical: 6.71%). This process was also accompanied by the phase transformation from the monohydrate to anhydrous form, which was confirmed by PXRD measurements for the sample after thermal dehydration (see Fig. S7†). The anhydrate shows no weight loss before its melting point in Fig. 9b. An obvious endothermic peak occurs around 229 °C for both specimens, along with a major weight loss, which may be attributed to a combined effect of melting and decomposition. The almost same temperature onset of the endotherm indicates the identical structures of both samples at this point as well. This phenomenon also appeared in the study of the nylon 5T monomer PDA–TPA.49 However, the melting point of PDA–IPA is a little lower than that of PDA–TPA (Tm = 240 °C), which may be more advantageous during polymerization.
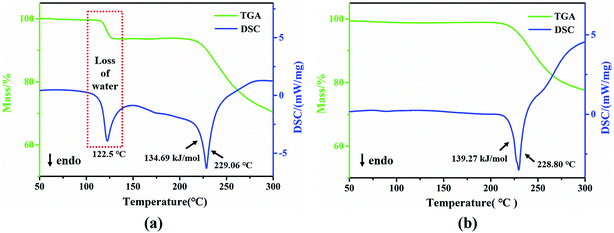 |
| Fig. 9 TGA and DSC curves of the monohydrate (a) and anhydrate (b) of PDA–IPA. | |
In addition, the heat of fusion values were calculated, with 134.69 kJ mol−1 for the monohydrate and 139.27 kJ mol−1 for the anhydrous form. The crystal energy of the monohydrate appeared slightly lower than that of the anhydrate, suggesting that the monohydrate is more stable in this situation.
Transitions between the monohydrate and anhydrate
The effects of temperature and relative humidity on the stability and transition between the PDA–IPA monohydrate and anhydrous phase were investigated by SST experiments, and the results are displayed in Fig. 10. Fig. 10a illustrates the influence of the two research factors on the stability of the monohydrate. When the temperature exceeds 80 °C, it can be observed that the monohydrate can be converted to the anhydrate. The crystal monohydrate appears to have favorable stability in terms of the humidity, even though the value was close to 0%. The transition from the monohydrate to anhydrous form was mainly dominated by the temperature. On the contrary, the stability of the anhydrate form was minimally affected by temperature, but extremely sensitive to the surrounding relative humidity. Fig. 10b shows that the anhydrate could be transformed to the monohydrate when the relative humidity was greater than 67%. However, the transformation phenomenon would not occur if the humidity was lower than 43%. Thus, the transformation behaviors in the SST experiments can be mainly attributed to the effect of the water activity in the environment.54 This indicated that the transformation between the anhydrous form and monohydrate of PDA–IPA is reversible under the appropriate conditions.
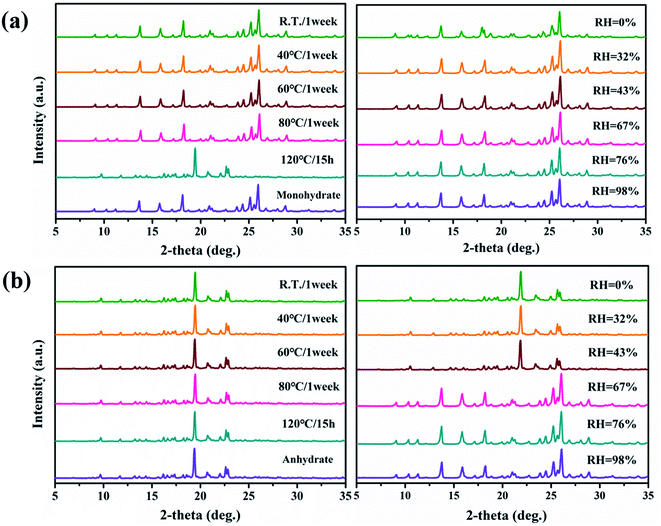 |
| Fig. 10 The SST process of the monohydrate (a) and anhydrate (b) under different temperature and humidity conditions. | |
The hygroscopicity experiments of the monohydrate and anhydrous forms of PDA–IPA subjected to various surrounding humidity values were further performed at room temperature, and the results can be seen in Fig. S8.† When the relative humidity was 67% or less, it showed that the two crystalline solid powders were stable. When it was 76%, both forms indicated slight moisture absorption. Finally, when it reached up to 98%, obvious moisture absorption can be observed, but the crystal structure was still unexpectedly stable in this case.
In order to clarify the effect of the solvent on the phase transition and explore whether there are new unknown crystal forms, the experiments of solvent-mediated transformation in different pure solvents or binary organic–water mixtures were also carried out. The findings are illustrated in Fig. 11. Fig. 11a and b shows us that no new polymorphs occur under the test conditions, except for a phase transformation between the monohydrate and anhydrate. The hydrate was the preferential structure in the system of water participation. To further shed light on the influence mechanism of temperature and water activity on the relative stability and transformation of the two crystal forms of PDA–IPA under various thermodynamic conditions, a slurry experiment in a water–ethanol binary system was designed and performed.
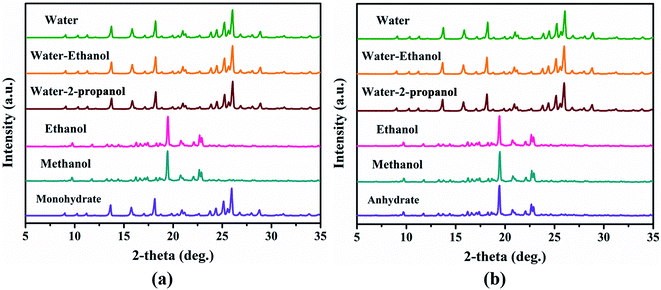 |
| Fig. 11 The SMPT profiles for the monohydrate (a) of PDA–IPA, and its anhydrate (b) in different solvents. The ratio in the binary solvent system is 1 : 1 (v : v). | |
The phase diagram after equilibration for 24 h was measured to investigate the dependence of PDA–IPA on the water activity (aw) in ethanol/water mixtures during the hydration process at different temperatures. The results were displayed in Fig. 12. At 25 °C, if the value of aw was lower than 0.14, the anhydrate was the favorable form at equilibrium, whereas the monohydrate appeared to be more stable if the value was more than 0.15. The area of the anhydrous zone was narrow, and the hydrate zone was rather widespread in this case (the phase diagram of the water activity–temperature conversion at ambient temperature is shown in Fig. S9 and S10†). However, at 60 °C, the anhydrate was the stable form if the value of aw was less than 0.38, whereas the monohydrate will be more stable if the value was more than 0.39. Notably, the value of the transition temperature of the two forms can be significantly affected by the water activity, showing a clear trend of rising with the increase of the water activity. With increasing water activity, the transition temperature from the anhydrate to monohydrate form increases. Meanwhile, we can see that the anhydrous zone of the PDA–IPA crystals is relatively narrow compared with its hydrate zone. In addition, the trend became more noticeable as the temperature and water activity increased.
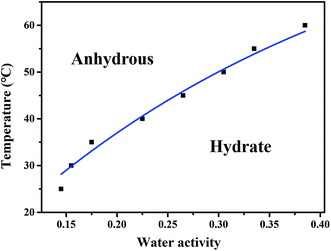 |
| Fig. 12 Water activity–temperature phase diagram of the PDA–IPA monohydrate and anhydrous form, which determined the boundary of transformation between the hydrate and anhydrous zones. | |
Conclusions
In this contribution, we found that PDA–IPA exists in two crystal forms. One is the rod-like orthorhombic monohydrate, and the other form is the block-like monoclinic anhydrate. We investigated their crystal structures, thermal properties, stabilities, transformation behaviors, as well as their conversion mechanisms by SCXRD, PXRD, FT-IR, TGA-DSC, and transformation experiments.
The huge difference in the crystal structure conformation of the monohydrate and anhydrous phases, which leads to their distinct hydrogen bonding modes and intermolecular interactions. There are two types of dominant hydrogen-bond contacts, N–H⋯O and O–H⋯O, in the monohydrate. Conversely, the anhydrate form only has the N–H⋯O interaction. The Hirshfeld surface analysis shows the differences clearly between the two forms of PDA–IPA to study their structure–property relationships. Moreover, the water loss of the monohydrate leads to the changes in packing and conformation, which are critical to the transformation into the anhydrous form.
The relative stability relationship between the two forms was further established. The stability of the monohydrate form against hygroscopicity was proved to be higher than that of the anhydrous phase. The transformation from the monohydrate to the anhydrate was mainly dominated by the temperature in the direct solid–solid transformation experiments. However, the conversion from the anhydrate to hydrate was hardly influenced by the temperature, but more sensitive to the surrounding relative humidity. Besides, it was found that the phase transition between the two crystal forms depends strongly on the temperature and water activity. Through the water activity–temperature phase diagram, the zones where the two crystalline forms can exist in stable forms were determined. The results presented in this work could give some meaningful instructions for the control preparation of the target crystal form of PDA–IPA, and offer the high-quality monomer for the bio-based nylon 5I.
Conflicts of interest
The authors declare that there is no conflict of interest.
Acknowledgements
This work was supported by the National Natural Science Foundation of China (NSFC 21908100), the Jiangsu Synergetic Innovation Center for Advanced Bio-Manufacture (XTE1847), the Key R&D Plan of Jiangsu Province (BE2019001) and National Key Research and Development Program of China (2019YFD1101202, 2019YFD1101204).
References
- B. Liu, G. Hu, J. Zhang and W. Yan, Non-isothermal crystallization, yellowing resistance and mechanical properties of heat-resistant nylon 10T/66/titania dioxide/glass fibre composites, RSC Adv., 2019, 9(13), 7057–7064 Search PubMed.
- T. F. Novitsky, C. A. Lange, L. J. Mathias, S. Osborn, R. Ayotte and S. Manning, Eutectic melting behavior of polyamide 10,T-co-6,T and 12,T-co-6,T copolyterephthalamides, Polymer, 2010, 51(11), 2417–2425 Search PubMed.
- Y. J. Kim, K. E. Yohana, H. S. Lee and J. Kim, Solid-State Polymerization of Semiaromatic Copolyamides of Nylon-4,T and Nylon-4,6: Composition Ratio Effect and Thermal Properties, Ind. Eng. Chem. Res., 2012, 51(49), 15801–15810 Search PubMed.
- R. J. Gaymans and A. G. J. V. d. Ham, Nylon 4,I: an amorphous polyamide, Polymer, 1984, 25, 1755 Search PubMed.
- M. Firdaus and M. A. R. Meier, Renewable polyamides and polyurethanes derived from limonene, Green Chem., 2013, 15, 370–380 Search PubMed.
- K. Hashimoto, N. Hashimoto, T. Kamaya, J. Yoshioka and H. Okawa, Synthesis and properties of bio-based polyurethanes bearing hydroxy groups derived from alditols, J. Polym. Sci., Part A: Polym. Chem., 2011, 49(4), 976–985 Search PubMed.
- T. A. Osswald and S. García-Rodríguez, History of sustainable bio-based polymers, Green Chem., 2011, 1–21 Search PubMed.
- P. Yang, X. Li, H. Liu, Z. Li, J. Liu, W. Zhuang, J. Wu and H. Ying, Thermodynamics, crystal structure, and characterization of a bio-based nylon 54 monomer, CrystEngComm, 2019, 21(46), 7069–7077 Search PubMed.
- D. S. Ogunniyi, Castor oil: a vital industrial raw material, Bioresour. Technol., 2006, 97(9), 1086–1091 Search PubMed.
- S. H. Hong, J. S. Kim, S. Y. Lee, Y. H. In, S. S. Choi, J. K. Rih, C. H. Kim, H. Jeong, C. G. Hur and J. J. Kim, The genome sequence of the capnophilic rumen bacterium Mannheimia succiniciproducens, Nat. Biotechnol., 2004, 22(10), 1275–1281 Search PubMed.
- J. Bosch, Modeling Techniques for Measuring Galaxy Properties in Multi-Epoch Surveys, J. Bus. Strat., 2011, 35(6), 49–57 Search PubMed.
- D. J. Burke, T. Kawauchi, M. J. Kade, F. A. Leibfarth, B. McDearmon, M. Wolffs, P. H. Kierstead, B. Moon and C. J. Hawker, Ketene-Based Route to Rigid Cyclobutanediol Monomers for the Replacement of BPA in High Performance Polyesters, ACS Macro Lett., 2012, 1(11), 1228–1232 Search PubMed.
- B. Bechtloff, S. Nordhoff and J. Ulrich, Pseudopolymorphs in industrial use, Cryst. Res. Technol., 2015, 36(12), 1315–1328 Search PubMed.
- P. A. Basford, K. R. Back, M. Cram, R. Docherty, R. J. Davey and A. J. Cruz-Cabeza, Impact of Crystal Structure and Molecular Conformation on the Hydration Kinetics of Fluconazole, Cryst. Growth Des., 2019, 19(12), 7193–7205 Search PubMed.
- P. Cui, Q. Yin, Y. Guo and J. Gong, Polymorphic Crystallization and Transformation of Candesartan Cilexetil, Ind. Eng. Chem. Res., 2012, 51(39), 12910–12916 Search PubMed.
- H. G. Brittain, Polymorphism in Pharmaceutical Solids, Informa Healthcare, New York, 1999, vol. 192 Search PubMed.
- K. Fujii, M. Aoki and H. Uekusa, Solid-State Hydration/Dehydration of Erythromycin A Investigated by Ab Initio Powder X-ray Diffraction Analysis: Stoichiometric and Nonstoichiometric Dehydrated Hydrate, Cryst. Growth Des., 2013, 13(5), 2060–2066 Search PubMed.
- Z. Liu, Q. Yin, X. Zhang, J. Gong and C. Xie, Characterization and Structure Analysis of Cefodizime Sodium Solvates Crystallized from Water and Ethanol Binary Solvent Mixtures, Ind. Eng. Chem. Res., 2014, 53(8), 3373–3377 Search PubMed.
- S. SeethaLekshmi, M. S. R. N. Kiran, U. Ramamurty and S. Varughese, Phase Transitions and Anisotropic Mechanical Response in a Water-rich Trisaccharide Crystal, Cryst. Growth Des., 2020, 20(1), 442–448 Search PubMed.
- F. Liu, D. E. Hooks, N. Li, N. A. Mara and J. A. Swift, Mechanical Properties of Anhydrous and Hydrated Uric Acid Crystals, Chem. Mater., 2018, 30(11), 3798–3805 Search PubMed.
- W. Du, Q. Yin, H. Hao, Y. Bao, X. Zhang, J. Huang, X. Li, C. Xie and J. Gong, Solution-Mediated Polymorphic Transformation of Prasugrel Hydrochloride from Form II to Form I, Ind. Eng. Chem. Res., 2014, 53(14), 5652–5659 Search PubMed.
- R. R. E. Steendam, U. B. R. Khandavilli, L. Keshavarz and P. J. Frawley, Solution versus Crystal Hydration: The Case of γ-Amino Acid Pregabalin, Cryst. Growth Des., 2019, 19, 4483–4488 Search PubMed.
- M. Sacchetti, Determining the relative physical stability of anhydrous and hydrous crystal forms of GW2016, Int. J. Pharm., 2004, 273(1–2), 195–202 Search PubMed.
- Y. Li, P. S. Chow, R. B. H. Tan and S. N. Black, Effect of Water Activity on the Transformation between Hydrate and Anhydrate of Carbamazepine, Org. Process Res. Dev., 2008, 12(2), 264–270 Search PubMed.
- X. Zhang, Q. Yin, W. Du, J. Gong, Y. Bao, M. Zhang, B. Hou and H. Hao, Phase Transformation between Anhydrate and Monohydrate of Sodium Dehydroacetate, Ind. Eng. Chem. Res., 2015, 54(13), 3438–3444 Search PubMed.
- H. Grünewald, Book Review: The Merck Index. An Encyclopedia of Chemicals and Drugs, Angew. Chem., Int. Ed., 1969, 8(1), 86–87 Search PubMed.
- J. Boeckmann and C. Näther, Solid-state transformation of [Co(NCS)2(pyridine)4] into [Co(NCS)2(pyridine)2]n: from Curie-Weiss paramagnetism to single chain magnetic behaviour, Dalton Trans., 2010, 39(45), 11019–11026 Search PubMed.
- D. C. Souza, V. Pralong, A. J. Jacobson and L. F. Nazar, A reversible solid-state crystalline transformation in a metal phosphide induced by redox chemistry, Science, 2002, 296(5575), 2012–2015 Search PubMed.
- F. Liu, F. Sommer, C. Bos and E. J. Mittemeijer, Analysis of solid state phase transformation kinetics: models and recipes, Metall. Rev., 2007, 52(4), 193–212 Search PubMed.
- F. Zou, Q. Chen, P. Yang, J. Zhou, J. Wu, W. Zhuang and H. Ying, Solution-Mediated Polymorphic Transformation: From Amorphous to Crystals of Disodium Guanosine 5′-Monophosphate in Ethanol, Ind. Eng. Chem. Res., 2017, 56, 8274–8282 Search PubMed.
- D. L. T. Nguyen and K.-J. Kim, Solvent-Mediated Polymorphic Transformation of α-Taltirelin by Seeded Crystallization, Chem. Eng. Technol., 2016, 39(7), 1281–1288 Search PubMed.
- A. Maher, C. C. Seaton, S. Hudson, D. M. Croker, Å. C. Rasmuson and B. K. Hodnett, Investigation of the Solid-State Polymorphic Transformations of Piracetam, Cryst. Growth Des., 2012, 12, 6223–6233 Search PubMed.
- A. Maher, D. M. Croker, C. C. Seaton, Å. C. Rasmuson and B. K. Hodnett, Solution-Mediated Polymorphic Transformation: Form II to Form III Piracetam in Organic Solvents, Cryst. Growth Des., 2014, 14, 3967–3974 Search PubMed.
- L. Chee-wei Jennifer and W. R. Ronald, Solubilities of and Transformations between the Anhydrous and Hydrated Forms of L-Serine in Water–Methanol Solutions, Cryst. Growth Des., 2006, 6(8), 1808–1812 Search PubMed.
- S. Maruyama, H. Ooshima and J. Kato, Crystal structures and solvent-mediated transformation of Taltireline polymorphs, Chem. Eng. J., 1999, 75(3), 193–200 Search PubMed.
- R. Mohan, K. K. Koo, C. Strege and A. S. Myerson, Effect of Additives on the Transformation Behavior of l-Phenylalanine in Aqueous Solution, Ind. Eng. Chem. Res., 2001, 40(26), 6111 Search PubMed.
- P. Yang, C. Lin, W. Zhuang, Q. Wen, F. Zou, J. Zhou, J. Wu and H. Ying, Insight into a direct solid–solid transformation: a potential approach for the removal of residual solvents, CrystEngComm, 2016, 18, 1699–1704 Search PubMed.
- Z. Ding, W. Su, X. Huang, B. Tian, X. Cheng, Y. Mao, G. Li, H. Liu and H. Hao, Understanding the Role of Water in Different Solid Forms of Avibactam Sodium and Its Affecting Mechanism, Cryst. Growth Des., 2020, 20(2), 1150–1161 Search PubMed.
- Bruker, SAINT and SMART-1000, Bruker AXS Inc., Madison, Wisconsin, USA, 2012 Search PubMed.
- G. M. Sheldrick, SHELXT – Integrated space-group and crystal-structure determination, Acta Crystallogr., 2015, 71(1), 3–8 Search PubMed.
- I. Usón and G. M. Sheldrick, An introduction to experimental phasing of macromolecules illustrated by SHELX: new autotracing features, Acta Crystallogr., 2018, 74(2), 106 Search PubMed.
- A. L. Spek, Single-crystal structure validation with the program PLATON, J. Appl. Crystallogr., 2003, 36(1), 7–13 Search PubMed.
- A. Gavezzotti and G. Filippini, Geometry of the Intermolecular X-H.cntdot..cntdot..cntdot.Y (X, Y = N, O) Hydrogen Bond and the Calibration of Empirical Hydrogen-Bond Potentials, J. Phys. Chem., 1994, 98, 4831–4837 Search PubMed.
- F. L. Hirshfeld, Bonded-atom fragments for describing molecular charge densities, Theor. Chim. Acta, 1977, 44(2), 129–138 Search PubMed.
- M. J. Turner; J. J. McKinnon; S. K. Wolff; D. J. Grimwood; P. R. Spackman; D. Jayatilaka and M. A. Spackman, CrystalExplorer17, The University of Western Australia, 2017 Search PubMed.
- M. A. Spackman and J. J. McKinnon, Fingerprinting intermolecular interactions in molecular crystals, CrystEngComm, 2002, 4, 378–392 Search PubMed.
- P. Yang, X. Li, H. Liu, Z. Li, J. Liu, W. Zhuang, J. Wu and H. Ying, Thermodynamics, crystal structure, and characterization of a bio-based nylon 54 monomer, CrystEngComm, 2019, 21, 7069–7077 Search PubMed.
- P. Yang, X. Peng, S. Wang, D. Li, M. Li, P. Jiao, W. Zhuang, J. Wu, Q. Wen and H. Ying, Crystal structure, thermodynamics, and crystallization of bio-based polyamide 56 salt, CrystEngComm, 2020, 22, 3234–3241 Search PubMed.
- Z. Li, P. Yang, H. Liu, J. Liu, S. Zhu, X. Li, W. Zhuang, J. Wu and H. Ying, Crystal forms and phase transformation of 1,5-pentanediamine-terephthalate: a bio-based nylon 5T monomer, Acta Crystallogr., Sect. B: Struct. Sci., Cryst. Eng. Mater., 2020, 76, 524–533 Search PubMed.
- M. A. Spackman and D. Jayatilaka, Hirshfeld surface analysis, CrystEngComm, 2009, 11(1), 19–32 Search PubMed.
- X. Zhang, L. Zhou, C. Wang, Y. Li, Y. Wu, M. Zhang and Q. Yin, Insight into the role of hydrogen bonding playing in the molecular self-assembly process of sulfamethazine solvates, Cryst. Growth Des., 2017, 17, 6151–6157 Search PubMed.
- A. M. Tudor, S. J. Church, P. J. Hendra, M. C. Davies and C. D. Melia, The Qualitative and Quantitative Analysis of Chlorpropamide Polymorphic Mixtures by Near-Infrared Fourier Transform Raman Spectroscopy, Pharm. Res., 1993, 10(12), 1772–1776 Search PubMed.
- C. S. Jamieson, A. M. Mebel and R. I. Kaiser, Understanding the Kinetics and Dynamics of Radiation-induced Reaction Pathways in Carbon Monoxide Ice at 10 K, Astrophys. J., Suppl. Ser., 2006, 163(1), 184–206 Search PubMed.
- H. Qu, M. Louhi-Kultanen, J. Rantanen and J. Kallas, Solvent-Mediated Phase Transformation Kinetics of an Anhydrate/Hydrate System, Cryst. Growth Des., 2006, 6(9), 2053–2060 Search PubMed.
Footnote |
† Electronic supplementary information (ESI) available. CCDC 1969111 and 1969113. For ESI and crystallographic data in CIF or other electronic format see DOI: 10.1039/d0ra08767j |
|
This journal is © The Royal Society of Chemistry 2020 |