DOI:
10.1039/D0RA07911A
(Paper)
RSC Adv., 2020,
10, 40625-40636
Effect of the heating process on the physicochemical characteristics and nutritional properties of whole cotyledon soymilk and tofu
Received
16th September 2020
, Accepted 27th October 2020
First published on 9th November 2020
Abstract
This study focused on the effect of the heating process on the whole cotyledon soymilk and tofu. Whole cotyledon soymilk was made from soybean cotyledon and processed by enzymatic hydrolysis using cellulase and high-pressure homogeneity. In this study, a one-step heating method was selected for the cooking process of whole cotyledon soybean milk, and the whole cotyledon soybean milk was heated to 90 °C and held for 4 min. Results showed that the protein, total saccharides and dietary fiber content of the whole cotyledon soymilk were higher than those of the tradition soymilk due to the existence of bean dregs (okara). Both protein aggregation and protein–polysaccharide interaction were observed during the heating process. We also found a change in soymilk physicochemical characteristics such as particle size distribution, viscosity, surface hydrophobicity and soluble protein during the heating process. The results in this study showed that compared with traditional tofu, the phytic acid and trypsin inhibitor content in whole cotyledon tofu was lower, so its protein had higher digestibility in vitro. In conclusion, whole cotyledon tofu had better health properties and application prospects.
1. Introduction
In the recent years, macrobiotic and vegetarian soybean dishes, such as tofu, have become more popular among people seeking to prevent illness, reduce cholesterol levels, and generally improve their health.1 Traditionally, tofu is made by coagulating soymilk from whole soybean.2 The traditional procedures of tofu production including cleaning, soaking, grinding beans, filtering, boiling, coagulation, and pressing.3,4 Traditional tofu processing technology has many problems, such as low utilization of raw material, unpleasant odor, and bean dreg (okara) disposal,4 and it is time-consuming, and this restricts the standardization and modernization of soy food manufacturing.4,5
Okara in soymilk has a negative effect on the gelling property of tofu, so it is generally filtered out during tofu processing.6 However, okara is rich in dietary fiber and protein.7 Disgarding okara causes nutrient loss and environmental pollution.8 In the process of making tofu, if the okara could be preserved, it can greatly improve the material utilization and the nutritional value of tofu.9,10 Previous studies demonstrate that whole cotyledon soymilk treated with fine milling and calcium sulfate addition could successfully produce whole-soybean tofu.11 Because of the existence of okara, whole-soybean tofu has enhanced hardness and springiness than traditional tofu.12,13
Heating treatment is the most important step for tofu gel structure formation from soybean protein.14 During soymilk heating process, hydrophobic region and sulfhydryl groups are exposed, it facilitates soymilk solidification. Finally, unique texture of tofu is obtained.15 The heating conditions have a great influence on the characteristics of soybean milk. Compared with traditional tofu, whole cotyledon tofu processed by high pressure and high temperature has higher protein content and larger average particle size.16 Milder thermal treatment (63 °C for 30 min and 72 °C for 15 s) results in a moderate increase of the surface hydrophobicity of soybean protein.17
The previous and recent researches are mostly focusing on the reducing the size of insoluble particles in whole cotyledon soymilk18 and coagulant selection.19 In addition, the physicochemical and sensory properties changes of traditional tofu during heat-treatment has been well studied.20,21 Because of existence okara, the whole cotyledon tofu may show different physical and chemical changes and texture changes during the heat treatment. The whole cotyledon tofu may also exhibit different nutritional characteristics. However, the effects of heating process on whole cotyledon soymilk or whole soybean tofu are seldom concerned. The objective of this research is to explore the optimized heating condition by determining the texture properties of whole cotyledon tofu. This study will also focus for the first time on the nutritional characteristics and gastrointestinal digestive characteristics of whole cotyledon tofu. Provide guidance for the production of whole cotyledon soymilk and tofu.
2. Materials and methods
2.1. Materials
Soybeans harvested from northeast China were dehulled by a local factory in Shandong province, and all the raw materials were stored in a hermetic plastic package until further experiments. All reagents were of analytical purity and purchased from Sigma Chemical Co., Ltd. (St. Louis, Mo., USA).
2.2. Whole cotyledon soymilk preparation
The samples of whole cotyledon soymilk for experiments were prepared according to Kuo's research19 with a little modification as following steps:
step 1: 50 g of whole soybean cotyledon was soaked using 200 mL of distilled water for 4 h at 20 °C.
Step 2: the hydrated cotyledon was drained and mixed with 500 mL of boiling water, the mixture was then heated in a thermostat water bath at 100 °C for 5 min to obtain the blanched soybean cotyledon. Subsequently, the blanched soybean cotyledon was ground with 350 mL distilled water using a soymilk grinder (Model JYL-Y5, Joyoung Co., China) for 4 min to obtain the slurry.
Step 3: 400 mL of slurry mixed with 3.2 g of commercial cellulase preparation (enzyme activity of cellulase: 7159 U g−1). The mixture was placed into a thermostatic heating magnetic stirrer (Model DF-101, Yuhua Analytical Instrument Co. Ltd., China), and then stirred at 50 °C for 3.5 h to obtain enzymatic hydrolysate of soymilk.
Step 4: enzymatic hydrolysate of soymilk was homogenized 4 times at 30 MPa by high pressure homogenizer (Model J04010065, APV Co., Germany) to obtain the raw whole cotyledon milk.
2.3. Whole cotyledon tofu preparation
Whole cotyledon tofu was prepared according to Shin's research with a little modification.22 Briefly, 0.5 g of NaCl was added to 500 g of cooked whole cotyledon soymilk. After mixing, 1.75 g of CaSO4 was added at 75 °C. When the soymilk was cooled down to 60 °C, let it stand for 20 min. The 500 g cooked soymilk was put into the mold (80 mm × 80 mm × 80 mm), suppressed with 1 kg pressure for 40 min.
2.4. Tradition soymilk preparation
The samples of tradition soymilk for experiments were prepared according to Lv's research with a little modification.23 Briefly, soybeans were washed and soaked in distilled water (soybean
:
water = 1
:
4) for 10 h at 25 °C. The hydrated soybeans were ground with distilled water (1 g of dry soybean per 10 mL of distilled water) for 4 min by a grinder (Model JYL-Y5, Joyoung Co., China). The homogenate was filtered by a 100 mesh sieve.
2.5. Heating treatment
The sample of raw whole cotyledon soymilk was heated from 20 °C to boiling point (95 °C) in 6 min, the temperature was rising at a rate of 8 °C min−1. Then keep boiling for 2, 4, 6, 8, or 10 min with continuous stirring. Heated soymilk was quickly cooled in iced water bath to room temperature.
2.6. Analysis of whole cotyledon soymilk particles size distribution
The distribution of particles size was measured by laser light-scattering with a Series Particle Size analyzer (Model Master Sizer 2000, Malvern Instruments Ltd., UK). The refractive indexes used for the dispersed phase and water were 1.460 and 1.330, respectively. Each sample was measured in triplicate and expressed as the percentage of volumetric particle size distributions by the Mastersizer2000 analytical software.
2.7. Viscosity measurement
Viscosity of soymilk was determined by a rotary viscometer (Model NDJ-5S, LICHEN Co., China) equipped with no. 1 rotor. The soymilk was added to beaker until the soymilk in parallel with the 30 rpm rotor's scale mark.
2.8. Surface hydrophobicity (H0) measurement
H0 was determined according to the method of Alizadeh-Pasdar & Li-Chan24 with some modifications. The sample of whole cotyledon soymilk was diluted 500–5000 times with 0.1 M phosphate buffer (pH 6.8). The soluble protein content of diluted sample was determined by Coomassie brilliant blue method. 20 μL of the ANS solution (8 M, be prepared in the same buffer) was added to 2 mL each of diluted soymilk samples and let stand for 3 min. The fluorescent intensity (FI) of the mixture was measured at wavelengths of 390 nm (excitation) and 470 nm (emission), respectively, and a slit width of 5 nm. The initial slope of the FI versus protein concentration (mg mL−1) plot (calculated by linear regression analysis) was used as an index of H0.
2.9. Determination of protein sulfhydryl group content in soymilk
The sulfhydryl group content in soymilk was measured according to Zuo's method.25 100 μL of soymilk was added with 9.9 mL of Tris–Gly buffer. After 20 min of ultrasonic demulsification, the diluted soymilk was added with 60 L of Ellman reagent. The mixture was kept at 25 °C for 20 min. The mixture was subsequently centrifuged for 20 min at 1644×g. The absorbance value of soymilk supernatant was determined at wavelength of 412 nm.
2.10. Sodium dodecyl sulfate-polyacrylamide gel electrophoresis (SDS-PAGE)
The concentrations of the stacking gel and separating gel were 5% and 12%, respectively. The samples were dissolved in the loading buffer (4 mL 10% SDS, 1.2 mL DTT, 1.6 mL Tris–HCl (1 M, pH 6.8), 4 mg of bromophenol blue and 2.2 mL 100% glycerol diluted in water to 20 mL). The solution was boiled for 5 min. Then 10 μL was loaded into sample well. The gel was stained by Coomassie Brilliant Blue G-250.
2.11. Texture profile analysis
2.11.1. Determination of gel strength. Cubic shaped samples (2.5 cm × 2.5 cm × 2.5 cm) were prepared from the central part of tofu. A test speed of 1.0 mm s−1 and type P/0.5 probe was used. The maximum displacement was 15 mm. Six replicate tests were carried out for every coagulant tofu.
2.11.2. Texture profile analysis (TPA). Cubic shaped samples (2.5 cm × 2.5 cm × 2.5 cm) were prepared from the central part of tofu. A test speed of 1.0 mm s−1 and type P/0.5 probe was used. The samples were compressed to 30% deformation. Six replicate tests were carried out for every coagulant tofu. And such qualitative parameters as hardness, springiness, cohesiveness, gumminess and chewiness were obtained.
2.12. Determination of color of the whole cotyledon tofu
The color of the whole cotyledon tofu was measured at 5 different positions for each sample by an automatic color difference meter (CR-400 Keshengxing Instrument Co. LTD, China). The value of L* represented the brightness or whiteness of tofu. The a* value (red/green) and the b* value (yellow/blue) were used as color indicators.
2.13. Determination of anti-nutrient factor in tofu
2.13.1. Determination of phytic acid content. The content of phytic acid in tofu was determined according to Garcia-Estepa's method.26 The tofu sample (1 g) was extracted under magnetic agitation with 40 mL of extraction solution (10 g/100 g Na2SO4 in 0.4 M HCl) for 3 h at room temperature. The suspension was centrifuged at 5000 rpm for 30 min and the supernatant was filtered. 10 mL of supernatant (containing between 3.3 and 9.0 mg of phytic acid) was mixed with 10.0 mL of 0.4 M HCl, 10.0 mL of 0.02 M FeCl3 and 10.0 mL of 20 g/100 g sulphosalicylic acid, shook gently and the tube used was sealed with a rubber cork through which passes a narrow 30 cm long glass tube, to prevent evaporation. The tube was placed in a boiling water bath for 15 min. The sample was centrifuged at 5000 rpm for 10 min, decanted, filtered and the residue was washed several times with distilled water. The supernatant and washed fractions were diluted (100 mL). 20 mL of mixture was adjusted to pH 2.5 ± 0.5 by addition of glycine was diluted to 200 mL. The solution was heated at 70–80 °C and titrated with 50 mM EDTA solution. The 4
:
6 Fe/P atomic ratio was used to calculate phytic acid content.
2.13.2. Determination of trypsin inhibitor activity (TIA). The TIA in tofu sample was determined according to Call's method.27 Briefly, the tofu sample was briefly shaken with 50 mL of 10 mM NaOH, and the pH of the slurry was adjusted to between 9.4–9.6. The slurry was shaken and left at 4 °C overnight. An aliquot of 1 mL of the benzoyl-m-arginine-p-nitroanilide solution was added to 600 μL of water and equilibrated for 10 min at two different temperature levels (37 °C and room temperature). After addition of 200 μL of trypsin working solution the mixture was incubated at either 37 °C or room temperature for a certain time (10–60 min), before the reaction was stopped by adding 200 μL of 30% acetic acid. After filtration or centrifugation, the absorbance of the clear solutions was measured at 410 nm. Percentages of inhibition (%) were calculated by comparing the absorbance measured for the trypsin standard to the absorbance measured for the sample extract, by following equation:
I (%) = (At − Abt) − (As − Abs)/(At − Abt) × 100 |
where I: percentage of inhibition, At: the absorbance of the solution with trypsin standard, Abt: the absorbance of the blank with trypsin standard, As: the absorbance of the solution with sample, TIA (mg g−1) was defined as trypsin inhibitory activity and was expressed in mg of inhibited trypsin per g of sample, by the following equation:
TIA (mg g−1) = I/100% × ρ × 5.6 × 103/mF |
where ρ: the mass concentration of trypsin working solution, 5.6 × 103: the factor considering trypsin purity, m (mg): the mass of tofu sample, F (mL/100 mL): the dilution of the sample extract.
2.14. Determination of the digestibility of tofu protein in vitro
2.14.1. Preparation of simulated gastric fluids (SGF) and simulated intestinal fluids (SIF). SGF and SIF were prepared according to Hu's method.28 The composition of SGF and SIF were shown in Table 1.
Table 1 Preparation of stock solution of simulated gastric fluid (SGF) and simulated intestinal fluid (SIF)
Components |
Concentration of electrolytic liquid |
SGF stock solution (pH = 3) |
SIF stock solution (pH = 7) |
Volume |
Concentration in SGF |
Volume |
Concentration in SIF |
g L−1 |
mol L−1 |
mL |
mM |
mL |
mM |
KCl |
37.3 |
0.5 |
6.9 |
6.9 |
6.8 |
6.8 |
KH2PO4 |
68.0 |
0.5 |
0.9 |
0.9 |
0.8 |
0.8 |
NaHCO3 |
84.0 |
1.0 |
12.5 |
25.0 |
42.5 |
85.0 |
NaCl |
117.0 |
2.0 |
11.8 |
47.2 |
9.6 |
38.4 |
MgCl2(H2O)6 |
30.5 |
0.15 |
0.4 |
0.1 |
1.1 |
0.33 |
(NH4)2CO3 |
48.0 |
0.5 |
0.5 |
0.5 |
— |
— |
CaCl2(H2O)2 |
44.1 |
0.3 |
— |
0.15 |
— |
0.6 |
2.14.2. Determination of the protein digestibility of tofu protein in SGF. Twelve g tofu samples were accurately weighed, and 300 mL simulated gastric juice with constant temperature of 37 °C was added. The samples were sealed and placed in a constant temperature water bath with stirring function. The tofu samples were stirred and digested at 37 °C for 0, 0.5, 1, 1.5 and 2 h. The digested solution was centrifuged at 4000 rpm for 10 min. Protein content in supernatant was determined by Kjeldahl method. Take the solution without adding the tofu sample as blank. In vitro digestibility of tofu protein in SGF was calculated as following formula.
where A1: in vitro digestibility in SGF (%), C1: protein content in supernatant, C2: protein content in blank sample, C3: total protein content before digestion.
2.14.3. Determination of the protein digestibility of tofu protein in SIF. The tofu sample was digested in SGF for 2 h. 200 mL of SGF containing tofu sample was mixed with 200 mL of SIF with a constant temperature of 37 °C. The pH value was adjusted to 7. The digestion solution was sealed and placed in a thermostatic water bath with stirring function, stirred and digested at 37 °C for 0, 0.5, 1, 1.5 and 2 h. The digested solution was centrifuged at 4000 rpm for 10 min. Protein content in supernatant was determined by Kjeldahl method. Take the solution without adding the tofu sample as blank. In vitro digestibility of tofu protein in SIF was calculated as following formula.
where A2 in vitro digestibility in SIF (%), C4 protein content in supernatant, C5 protein content in blank sample, C6 total protein content before digestion.
2.15. Statistical analysis
All determinations were conducted for three times, and the whole experiment was repeated twice. The data were analyzed by an one-way ANOVA and t-test by SSPS 19.0. All tests of significance were at p < 0.05.
3. Results and discussion
3.1. Chemical composition of whole cotyledon soymilk
Compared with the traditional soymilk, the whole cotyledon soymilk had higher content of protein, carbohydrate and dietary fiber (Table 2). It was mainly caused by the existence of okara in the soymilk. Macromolecules in okara play important roles in viscosity, particulate, protein content of soymilk and the breaking stress of tofu.6 Therefore, the nutrient content of whole cotyledon soymilk were different from traditional soymilk.
Table 2 Chemical compositions of raw whole cotyledon soymilk and traditional soymilk
Main components |
Content (g/100 mL) |
Whole cotyledon soymilk |
Traditional soymilk |
p < 0.001 when compared with whole cotyledon tofu. |
Protein |
4.68 ± 0.09 |
2.75 ± 0.03a |
Fat |
2.22 ± 0.24 |
2.04 ± 0.11 |
Carbohydrate |
2.10 ± 0.01 |
1.50 ± 0.03a |
Fiber |
1.42 ± 0.02 |
0.30 ± 0.01a |
3.2. The varying tendency of the volume average particle size and temperature of whole cotyledon milk during the heating process
As shown in Fig. 1, raw whole cotyledon soymilk was heated from 20 °C to boiling point (95 °C) for 6 min. After the soymilk was heated for 18 min, the boiling point was down to 91 °C. The decrease of the boiling point induced by the prolongation of boiling could be attributed to the composition changes of whole cotyledon soymilk during the heating process, such as degeneration, aggregation and Maillard reaction.29
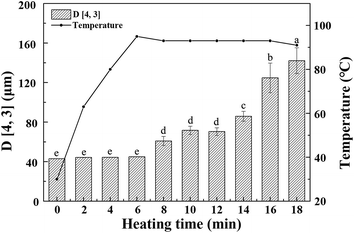 |
| Fig. 1 The varying tendency of volume average particle size and temperature of whole cotyledon soymilk during the process of heating. Bars indicate standard deviation. (a–e) Means do not share a common superscript letter are significantly different (p < 0.05). | |
As the heating temperature increasing, the volume average particle size of the whole cotyledon soymilk had no significant difference before the soymilk was boiled, and it increased slightly when heated to 6–12 min. Volume average particle size increased significantly after 12 min heating. This phenomenon was partly caused by the thermal aggregation of particles in the whole cotyledon soymilk.30 It was presumably caused by protein aggregation induced by heating and fiber adsorption in soymilk.31 Soybean cotyledons contain many kinds of polysaccharides such as cellulose, hemicellulose, and acidic polysaccharides.32 Aggregated protein particles were easy to combine with soluble polysaccharide chain, and then form a composite aggregation.33 In addition, after enzymatic hydrolysis of cellulase and high-pressure homogenization, the macromolecular cellulose in soymilk was disintegrated into fiber particles with smaller particle size and lower polymerization degree, which have large specific surface area and large amount of hydroxyl groups on the surface.34 With the soymilk temperature increase, hydrophobic areas of protein were gradually exposed after the protein heat-induced denaturation, fiber particles cannot absorb the protein.35 Protein molecules converge on each other during heating processing, and hydrophobic area wrap into protein aggregation again.36 Fiber particles with large specific surface area were easily adsorbed by macromolecular protein aggregation, which result in particle size of protein–fiber aggregate increasing quickly.37
3.3. The varying tendency of the particle size distribution of whole cotyledon soymilk during the heating process
The composition and quantity of protein particles significantly affect the physical and chemical properties of soymilk.38 As shown in Fig. 2, we observed significant difference of particle size distribution between whole cotyledon soymilk and tradition milk. The particle size distribution of raw whole cotyledon soymilk was mainly divided into two parts, the small peak was a monomodal (peak 1), with the size in the 0.3–2 μm range, while the larger part was formed by the merger of two peaks, the particle size were in the range of 2–8 μm (peak 2) and 8–130 μm (peak 3), respectively. The particle size distribution of raw traditional soymilk was divided into two parts. However, large size region was a monomodal, with the size in the 10–140 μm (peak 3) range, and the small size region was formed by the merger of two peaks, range in 0.2–4 μm (peak 1) and 4–10 μm (peak 2), respectively. It can be indicated that the traditional soymilk had more extensive particle size distribution, and its particle size was mostly distributed less than 10 μm, while the particle size of whole cotyledon soymilk was mostly distributed more than 50 μm.
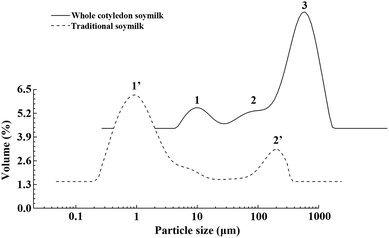 |
| Fig. 2 Differences of particle size distribution between the raw whole cotyledon soymilk and traditional soymilk. | |
Combining the main components analysis (Table 2) of whole cotyledon soymilk and traditional soymilk, it could be speculated that peak 1 might indicate soluble protein in the soymilk, peak 2 might be the macromolecular insoluble protein and peak 3 might indicate the decomposed cellulose.
To clarify the change of particle size distribution during heating producing, the particles size distribution of whole cotyledon soymilk was measured by the laser particle size analyzer. The particle size distribution of soymilk was not significantly changed before boiling (before 8 min, Fig. 3a). After boiling (after 8 min), the peak 1 disappeared, which mainly due to the heat-induced aggregation of the small molecular proteins in the whole cotyledon soymilk (Fig. 3). Thermal aggregation of protein requires the unfolding of the protein to generate aggregation-prone molecules. It also requires the ability of these molecules to approach each other close enough so that the intermolecular interaction for aggregates formation can efficiently occur, such as hydrophobic interactions.39 And boiling processing causes the dissociation, denaturation and aggregation of both 7S and 11S proteins.40
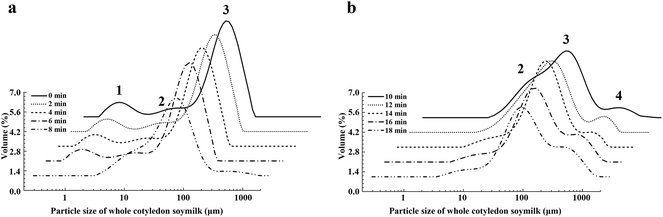 |
| Fig. 3 The varying tendency of particle size distribution of whole cotyledon soymilk during the heating process. (a) Heated 0–8 min and (b) heated 10–18 min. | |
The relative volume of peak 2 increased before heating 12 min, while decreased after heating 12–18 min. However, peak 3 decreased when heating for 6–10 min and increased when heating for 10–14 min. After heating for 14–18 min, the relation volume of peak 3 descended again (Fig. 3b). When the soymilk was boiled, we observed peak 4, which was distributed in the region of 200 μm. The relative volume of peak 4 was continuously rising with the prolongation of heating time.
The increasing of relative volume of peak 2 indicated the increase of insoluble protein produced by the denaturation and aggregation of soluble protein, and degraded-protein and fiber particles would form a protein–fiber complex.41,42 Peak 4 appeared after soymilk was boiled and it could be inferred that peak 4 might indicate the protein–fiber complex.
3.4. The changes of viscosity of whole cotyledon soymilk during the heating process
The viscosity of raw whole cotyledon soymilk was significantly higher than that of raw traditional soymilk. It was partly because whole cotyledon soymilk contained a higher content of okara, which was rich in protein and fiber. Higher concentration of nutrients indicated higher viscosity of soymilk system.6 In addition, as the fiber was degraded by cellulase, the contents of soluble polysaccharides in whole cotyledon soymilk were increased.
The change of viscosity and temperature of whole cotyledon soymilk during the heating process are shown in Fig. 4. It was consistent with the results of previous studies, which demonstrated that thermal treatment increased the viscosity of almond milk.43 With the temperature increased, the viscosity of the whole cotyledon milk was slightly decreased before it was boiled. However, when the temperature reached the boiling point, the viscosity increased rapidly from 20 Cp to 50 Cp. This phenomenon was caused by a series of reactions such as structural changes of protein molecules, disaggregation of oligomers, hydrophobic groups and hydrogen bond exposure reacted after soymilk heating.44
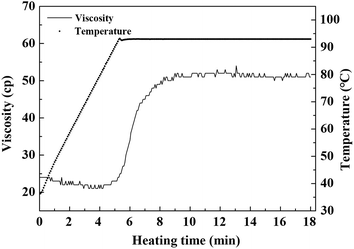 |
| Fig. 4 The varying tendency of viscosity and temperature of whole cotyledon soymilk during the heating process. | |
Therefore, with the heat-induced denaturation of protein in soymilk, the protein granular structure expanded, and its molecular surface area increased. The protein molecules collide with each other and the adjacent protein molecules were easy to form the disulfide bond, which cause the increase of soymilk viscosity.45 Besides, the soluble polysaccharide was combined with soybean protein to form the protein–polysaccharide gel, resulting in the increase of the soymilk viscosity.46 The high viscosity of soymilk had a great influence on the dispersion of coagulant in the coagulating process, and it could directly affect the quality of the whole cotyledon tofu product.47 The soluble fiber fraction could also contribute to the increase in viscosity by the extension and hydration of the biopolymer chains induced by the temperature.43
3.5. The change of surface hydrophobicity and soluble protein content of whole cotyledon soymilk during the heating process
The soluble protein content of whole cotyledon soymilk was continuously decreasing with the prolongation of heating time (Table 3). It was mainly because the small soluble protein particles in the whole cotyledon soymilk had aggregated after thermal degeneration, formed large particles of protein polymer with poor solubility.
Table 3 The changes of surface hydrophobicity and soluble protein content of whole cotyledon soymilk during the heating process
Boiling time |
0 min |
2 min |
4 min |
6 min |
8 min |
10 min |
12 min |
14 min |
16 min |
18 min |
P-value |
Surface hydrophobicity |
1071.72 ± 69.29 |
1021.84 ± 52.66 |
1147.44 ± 61.06 |
1544.07 ± 41.96 |
1785.84 ± 74.81 |
1743.90 ± 54.82 |
2024.34 ± 70.02 |
1731.80 ± 88.07 |
1303.80 ± 35.00 |
1274.15 ± 44.56 |
<0.0001 |
Soluble protein content (mg mL−1) |
230.04 ± 12.70 |
213.77 ± 11.20 |
157.34 ± 14.98 |
100.31 ± 7.01 |
69.33 ± 12.64 |
52.67 ± 11.05 |
48.43 ± 10.38 |
48.03 ± 10.26 |
47.87 ± 10.17 |
48.20 ± 11.05 |
<0.0001 |
During heating in 6–12 min, the aggregation between protein molecular was faster than the aggregation between protein and fiber, therefore the relative volume of peak 2 showed an increase and peak 3 showed a decrease trend. After 12 min heating, most of the soluble protein particles had been aggregated. It was consistent with the varying tendency of soluble protein content of whole cotyledon soymilk during the heating process. In this case, the aggregation between proteins was much slower than that between the protein and fiber. Therefore, the relative volume of peak 2 showed a downward trend and the peak 4 continued to increase.
The hydrophobicity of protein molecules was due to its hydrophobicity side-chains of amino acid residue.48 These side-chains would not interact with the polarity water molecules (with the exception of van der Waals force), which resulted in the hydrophobic groups tend to be combined in the interior of the natural globulin hydrophobic region.48,49 During heating process, the hydrophobic areas and hydrophobic groups in the protein were exposed, which contribute to the formation of protein gel network during coagulating process.50
As shown in Table 3, when the heating time was less than 12 min, protein surface hydrophobicity increased significantly with the prolongation of heating time, it showed that as the heating of whole cotyledon soymilk, protein hydrophobic area gradually exposed. With the prolongation of the boiling time of soymilk, the surface hydrophobicity of protein was reduced during heating 12 to 18 min. It suggested that the heat accumulation between proteins was faster than the disaggregation caused by thermal denaturation, exposed hydrophobic area was wrapped up again. The results showed that boiling time of the whole cotyledon soymilk had a great influence on the formation of protein gel network. When the soymilk was boiled for 6 min, the protein surface hydrophobic reached the maximum. Theoretically, it was the most beneficial condition for the coagulating process.
3.6. Effect of heating method on viscosity and sulfhydryl content of soluble protein in whole cotyledon soymilk
It could be seen from Table 4 that the viscosity of soymilk obtained by two-step heating method was significantly higher than that of soymilk obtained by one-step heating method, while the content of sulfhydryl group was relatively low. Two-step heating method lengthened the heating time at lower temperature, allowing more polysaccharides to aggregate with the unfolding proteins, resulting in a reduction in the sulfhydryl content in whole cotyledon soymilk.
Table 4 Effect of heating method on viscosity and content of free sulfhydryl group in whole cotyledon soymilka
Heating method |
Condition |
Viscosity (mPa s) |
Free sulfhydryl group (μmol g−1) |
Different letters indicated significant differences (p < 0.05). Capital letters indicated the difference of viscosity of whole cotyledon soybean milk in different heating methods, and lower letters indicate the difference of thiol group content in whole cotyledon soybean milk in different heating methods. |
Two-step |
70 °C (5 min) to 95 °C (5 min) |
81.0 ± 0.01B |
0.95 ± 0.01d |
75 °C (5 min) to 95 °C (5 min) |
73.3 ± 0.6C |
1.13 ± 0.06d |
80 °C (5 min) to 95 °C (5 min) |
84.3 ± 0.6A |
1.36 ± 0.01c |
One-step |
85 °C (5 min) |
46.7 ± 0.6F |
1.51 ± 0.01c |
90 °C (5 min) |
62.3 ± 0.6E |
2.11 ± 0.06b |
95 °C (5 min) |
69.0 ± 0.01D |
2.45 ± 0.16a |
In one-step heating experiment, the viscosity of the whole cotyledon soymilk increased with the rise of temperature and sulfhydryl content also increased with heating temperature. It showed that for the one-step heating method, the increase of heating temperature could deepen the degree of protein degeneration and thus increased the content of sulfhydryl group. Meanwhile, as the heating time of one-step heating was shorter than two-step heating, the amount of sulfhydryl group wrapped by polysaccharide gel was relatively less, so one-step heating method was suitable for whole cotyledon soybean milk.
As shown in Table 5, when the boiling temperature was 85 °C and kept for 5 min, the tofu structure could hardly be solidified and formed. This result was consistent with the low content of sulfhydryl in soybean milk under this condition, indicating that the protein was not completely deformed and unfolded at this temperature, so it was not conducive to the subsequent formation of tofu gel. The gel strength, hardness, springiness and other structural characteristics of whole cotyledon soybean milk heated at 90 °C were all higher than those of the soymilk kept at 95 °C (boiling point), mainly because the boiling of whole cotyledon soybean milk for a long time would make more proteins produce gelation, and accelerate aggregation between polysaccharide and protein, which significantly increased the large particle in soymilk.51
Table 5 Effect of heating method on texture properties of whole cotyledon tofu
Temperature |
Texture properties |
Gel strength (g) |
Hardness (g) |
Springiness |
Chewiness |
90 °C (5 min) |
71.5 ± 4.9 |
108.9 ± 0.3 |
0.945 ± 0.003 |
37.8 ± 1.7 |
95 °C (5 min) |
61.0 ± 4.2 |
67.8 ± 2.1 |
0.939 ± 0.003 |
28.9 ± 4.4 |
As shown in Table 6, as the heating process continuous, the content of sulfhydryl group in water-soluble protein in soybean milk reached its maximum value at 4 min, but in general, the content of sulfhydryl group did not change significantly during the heating process. In the process of heating, protein structure denatured and the sulfhydryl group exposed to the surface of the protein structure. At the beginning of the heating process, the sulfhydryl content in soymilk increased slightly. As the heating continued, the sulfhydryl group exposed to the surface of the protein structure oxidized with oxygen to form molecular- or intramolecular-disulfide bonds.52,53 The results shown in the figure might be due to the fact that the exposure rate of sulfhydryl group in whole cotyledon soybean milk was close to the rate of disulfide bond re-formation, which resulted in the non-significant change of sulfhydryl content in soybean milk, and the ultrasonic demulsification treatment of experimental samples also had a certain influence on the content of protein sulfhydryl.
Table 6 The changes of sulfhydryl group content of whole cotyledon soymilk during the heating process
Boiling time |
0 min |
2 min |
4 min |
6 min |
8 min |
10 min |
12 min |
14 min |
16 min |
18 min |
P-value |
Sulfhydryl group content (μmol g−1 protein) |
2.43 ± 0.14 |
2.39 ± 0.12 |
3.40 ± 0.15 |
2.98 ± 0.13 |
2.62 ± 0.11 |
2.99 ± 0.09 |
2.49 ± 0.06 |
2.70 ± 0.07 |
2.59 ± 0.10 |
3.04 ± 0.08 |
<0.0001 |
3.7. SDS-PAGE profile of whole cotyledon soymilk during the heating process
According to the different sedimentation coefficient, soy protein could be divided into 4 components (2S, 7S, 11S and 15S). The components of 7S and 11S accounted for 80% of soybean protein. Many studies have shown that 7S subunit content is negatively correlated with the product yield, hardness and springiness of tofu, and the content of acidic polypeptides of 11S is positively correlated with yield and hardness of tofu, the basic polypeptides of 11S is positively correlated with yield of tofu.54,55
As shown in Fig. 5, soy protein of whole cotyledon soymilk exhibited the characteristic bands representing the subunits of 7S (α, α′, and β) and 11S (A and B). The bands of 24 kDa (BX1) and 18 kDa (BX2) are revealed to be oleosin proteins,56 which are unique proteins in lipid bodies.57
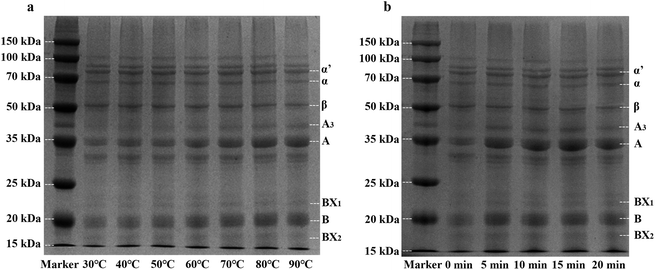 |
| Fig. 5 SDS-PAGE profile of whole cotyledon soymilk under different temperature (a) and different heating time (b) α, α′ and β: subunits of β-conglycinin; A, A3 and B: acidic and basic subunits of glycinin; BX1 and BX2: oleosins. | |
Fig. 5a showed the SDS-PAGE profile of whole cotyledon soymilk heated by different temperature (30–90 °C). Before the temperature reached to 60 °C, band intensities of 7S and 11S subunits had no significant change. However, when the treatment temperature was higher than 60 °C, band intensities of α, α′, and β subunits substantially decreased with the increase of soymilk temperature. In contrast, band intensities of A and B subunits substantially increased. These results were in agreement with previous work which reported that the larger particles in unheated soymilk were composed of 11S protein.38 Heating process decreases the solubility of 7S subunits protein, the large particles of 11S subunits protein were broken and more 11S subunits particles were recovered in the supernatants of heated soymilk.31 Therefore, the heating process could improve the ratio of 11S/7S subunits, which was beneficial to improve the quality of the whole cotyledon tofu.
Fig. 5b showed the SDS-PAGE profile of whole cotyledon soymilk heated to different time (0–20 min). Compared with the raw whole cotyledon soymilk, band intensities of 11S rapidly increased after 5 min heating. However, the change of the band intensities of both 7S and 11S subunits were not obvious after boiling. This phenomenon indicated the protein aggregation of both 7S and 11S after soymilk boiling, and the boiling time has no significant effect on the ratio of 11S/7S subunits.
3.8. Texture properties of whole cotyledon tofu prepared from whole cotyledon soymilk with different boiling time
The gel strength and hardness of whole cotyledon tofu were lower than those of traditional tofu under experimental conditions, which might be related to the presence of a large amount of soybean polysaccharide in soymilk. Polysaccharide concentration of whole cotyledon soymilk increased significantly after enzymatic hydrolysis, which resulted in accelerating of phase separation in the soymilk system. The polysaccharide molecules appear to aggregate together, and the protein particles cannot distribute evenly in aqueous dispersion. In addition, protein–polysaccharide complex can block the formation of gel network structure formed between proteins, which have a negative effect on the strength of tofu gel, and the structure of protein gel network was relatively loose.58–60
After 6 min boiling, the whole cotyledon soymilk could form tofu structure. Thus, we selected 0–6 min as the research gradient of boiling time in this experiment. The textural properties of whole cotyledon tofu prepared by whole cotyledon soymilk with different boiling time are shown in Table 7. With the prolongation of boiling time, the gel strength of the whole cotyledon tofu rose first and then decreased, it reached a maximum point at 4 min boiling. Compared to the gel strength, the hardness, the springiness, and the chewiness changed slightly with the extension of time. Together with the volume average particle size change of the soymilk, it indicated that protein denaturation and aggregation occurred during the 6 min boiling, protein molecules formed thermal gels under the physical interaction of hydrophobic interactions, hydrogen bonding, electrostatic interaction.61 The hydrophobic region is encapsulated by the protein aggregate, which then affect the formation of the gel network structure in the coagulation process.62 The surface hydrophobicity of protein rose first and then decreased in boiling process, and reached the maximum value at 12 min after boiling. However, after 4 min of boiling, the gel strength of whole cotyledon tofu reached its maximum point. It suggests that the gel strength of whole cotyledon tofu was not only affected by the surface hydrophobicity of the protein, but also with other factors such as particle size and content of polysaccharides and viscosity. Considering the quality of the whole cotyledon tofu, the optimized heating condition of the whole cotyledon milk in the experimental conditions was determined: boil for 4 min.
Table 7 Texture properties of whole cotyledon tofu prepared from heating whole cotyledon soymilk with different boiling time
Boiling time |
0 min |
2 min |
4 min |
6 min |
P-value |
Gel strength (g) |
84.5 ± 7.8 |
94.5 ± 0.7 |
120.0 ± 9.9 |
84.0 ± 4.2 |
<0.0001 |
Hardness (g) |
77.1 ± 2.971 |
85.4 ± 0.727 |
94.8 ± 1.536 |
67.8 ± 2.164 |
<0.0001 |
Springiness |
0.8 ± 0.004 |
0.9 ± 0.013 |
1.0 ± 0.010 |
1.0 ± 0.003 |
<0.0001 |
Chewiness |
29.6 ± 1.415 |
38.6 ± 0.014 |
47.1 ± 0.254 |
28.9 ± 4.439 |
<0.0001 |
3.9. Comparison of the content of anti-nutrient factors between whole cotyledon tofu and traditional tofu
Phytic acid and trypsin inhibitors are widespread anti-nutritional factors in soybean. Phytic acid can chelate with calcium, iron, zinc and other minerals in food to form insoluble compounds, which affect the absorption of minerals in the small intestine.63 Trypsin inhibitors can inhibit or hinder the trypsin digestion function, thus affecting protein absorption.64 Thus reducing the content of the two substances has great significances to the nutrition quality of tofu.
In this study, the content of phytic acid and trypsin inhibitors in whole cotyledon tofu were significantly lower than those in the traditional tofu (Table 8). This was mainly because the soaking operation in traditional tofu process could effectively remove phytic acid from soybean embryo, and the water absorption rate of soybean cotyledon was much faster than that of soybean, which accelerated the phytic acid dissolution. In addition, the heating treatment of whole cotyledon tofu could effectively reduce the content and activity of anti-nutrient factors in tofu.
Table 8 Differences of anti-nutrient factor content between the whole cotyledon tofu and traditional tofu
Anti-nutrient factors |
Whole cotyledon tofu |
Traditional tofu |
p < 0.001 when compared with whole cotyledon tofu. |
Phytic acid (mg g−1) |
1.71 ± 0.81 |
23.35 ± 0.79a |
Trypsin inhibitor (mg g−1) |
0.56 ± 0.06 |
1.65 ± 0.06a |
3.10. Comparison of digestibility between whole cotyledon tofu and traditional tofu protein in vitro
As could be seen from Fig. 6a, the protein digestibility in SGF of whole cotyledon tofu increased rapidly in the first 0.5 h, while the protein digestibility changed slowly in the following 1.5 h. While the digestibility of traditional tofu kept constant growth within 2 h. In the SIF (Fig. 6b), the digestibility rate of whole cotyledon tofu and traditional tofu was very slow. With 2 h of digestion, the digestibility increased by about 10%.
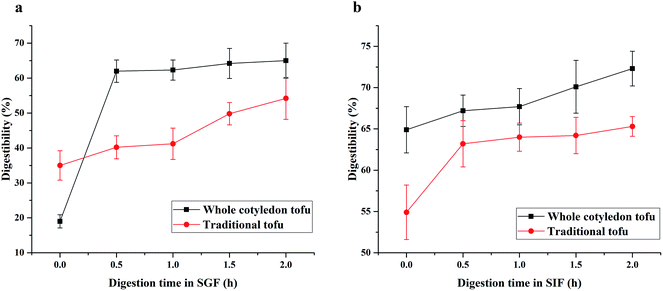 |
| Fig. 6 The difference of protein digestibility in SGF (a) and SIF (b) between whole cotyledon tofu and traditional tofu. | |
In this study, we found that the digestion rate of whole cotyledon tofu was faster than traditional tofu. The reasons were as follows: (1) protein denaturation occurred under suitable heating conditions, peptide chain was broken, the folded part of peptide chain became loose, and the action site of digestive enzyme was exposed, digestive enzyme was easier to play the role of digestion.65 (2) Phytic acid chelated metal ions and polymerized with protein, resulting in the protease sites being shielded. In addition, trypsin inhibitor could inhibit the trypsin activity. The above results observed in this study confirmed that heating treatment could reduce the content of trypsin inhibitor in the whole cotyledon tofu, thus improving the protein digestibility of whole cotyledon tofu.
3.11. Comparison of physical properties between whole cotyledon tofu and traditional tofu
Table 9 showed the color difference between the two types of tofu. The whiteness of whole cotyledon tofu was significantly higher than that of traditional tofu, and the color of traditional tofu was more red and yellow. Soybean was rich in β-carotene, which caused the color of traditional tofu to be light yellow. However, β-carotene was unstable in heat, light and oxygen environment,66 while the whole cotyledon tofu was processed by heat treatment and enzymolysis, resulting in the decrease of β-carotene. Therefore, the color of the whole cotyledon tofu was whiter.
Table 9 Differences of color indexes between the whole cotyledon tofu and traditional tofu
Color index |
Whole cotyledon tofu |
Traditional tofu |
p < 0.001 when compared with whole cotyledon tofu. |
L* |
88.33 ± 0.86 |
81.13 ± 1.90a |
A* |
0.24 ± 0.09 |
1.77 ± 0.12a |
b* |
13.19 ± 0.39 |
15.93 ± 0.60a |
As shown in Table 10, the texture characteristics such as gel strength, hardness and chewiness were all lower than those of traditional tofu, while springiness was closer to traditional tofu. Combined with previous studies, the size of insoluble particles in the whole cotyledon soymilk was significantly larger than that in traditional soybean milk, which hindered the formation of protein gel network. In addition, the interaction between soybean protein and soybean residue polysaccharides was weaker than that between proteins. The stability of the gel network constructed by polysaccharide and protein was relatively poor.34 Therefore, the whole cotyledon tofu gel exhibits a weak gel state.
Table 10 Differences of texture properties between the whole cotyledon tofu and traditional tofu
|
Gel strength/g |
Hardness/g |
Springiness |
Chewiness |
p < 0.05 when compared with whole cotyledon tofu. p < 0.001 when compared with whole cotyledon tofu. |
Whole cotyledon tofu |
120.0 ± 9.9 |
94.8 ± 1.5 |
1.00 ± 0.01 |
47.1 ± 0.3 |
Traditional tofu |
178.4 ± 4.3a |
181.2 ± 4.3b |
0.99 ± 0.02 |
37.7 ± 1.8a |
4. Conclusions
Based on the above results, the heating parameters of whole cotyledons tofu were determined. In addition, compared with traditional tofu, whole cotyledon tofu could make full use of soybean raw materials and had higher nutritional value. The processing of whole cotyledon tofu included heat processing and enzymatic hydrolysis technology, which greatly reduced the content and activity of anti-nutrient factors and made higher protein digestibility of tofu. Therefore, whole cotyledon tofu had better health properties and application prospects. More researches are required to understand the mechanism of interaction between soy polysaccharide and protein during the heating process in order to illuminate the effects of polysaccharide on the formation of tofu gels.
Conflicts of interest
The authorship declare no competing interests.
Acknowledgements
This work was supported by Key Research and Development Program of Zhejiang Province 2017C02008 (to Z. G.) and National Key R&D Program of China 2016YFD0400202 (to Z. G.).
References
- D. J. Fukushima, J. Am. Oil Chem. Soc., 1981, 58, 346–354 CrossRef CAS.
- T. Ono, Y. Onodera, Y. Chen and K. Nakasato, ACS Symp. Ser., 2010, 1059, 219–229 CAS.
- C. R. Rekha and G. Vijayalakshmi, J. Food Sci. Technol., 2013, 50, 176–180 CrossRef CAS.
- Q. Zhang, C. Wang, B. K. Li, L. Li, D. Lin, H. Chen, Y. W. Liu, S. Q. Li, W. Qin, J. Liu, W. G. Liu and W. Yang, Crit. Rev. Food Sci. Nutr., 2018, 58, 1448–1467 CrossRef CAS.
- M. Faisal, F. Mulana, A. Gani and H. Daimon, Res. J. Pharm., Biol. Chem. Sci., 2015, 6, 1053–1058 CAS.
- K. Toda, J. Food Sci., 2007, 72, C108–C113 CrossRef CAS.
- J. Xiong, Y. Yang and Y. Hua, Soybean Sci., 2009, 28, 1119–1122 Search PubMed.
- M. H. Wong and C. C. Lay, Environ. Pollut., Ser. A, 1980, 23, 247–259 CrossRef CAS.
- L. L. Forster and L. K. Ferrier, J. Food Sci., 1979, 44, 583–590 CrossRef.
- H. Yu, X. Xu, Y. Wang, C. Piao, J. Liu, D. Ren, W. Dai and Y. Hu, J. Food Saf. Food Qual., 2015, 6, 1401–1408 Search PubMed.
- F.-J. Kao, N.-W. Su and M.-H. Lee, ACS Symp. Ser., 2010, 1059, 277–291 CAS.
- J. Sung Il, K. Ji-Eun and L. Sam-Pin, Food Sci. Biotechnol., 2011, 20, 437–444 CrossRef.
- C. Wu, X. Xia, Y. Cheng, J. Huo and J. Huo, Sci. Technol. Food Ind., 2015, 36, 143–152 CAS.
- C. H. Lee and C. Rha, J. Food Sci., 1978, 43, 79–84 CrossRef CAS.
- L. Wang, D. Li, E. Tatsumi, Z. Liu, X. Chen and L. Li, Chem. Eng. Process., 2007, 46, 486–490 CrossRef CAS.
- F. Zuo, X. Peng, X. Shi and S. Guo, Food Chem., 2016, 209, 50–56 CrossRef CAS.
- Q. Sui, H. Roginski, R. P. W. Williams, C. Versteeg and J. Wan, Int. Dairy J., 2011, 21, 206–213 CrossRef CAS.
- L. Zhang, P. Chen, J. Huang and G. Yang, J. Appl. Polym. Sci., 2003, 88, 422–427 CrossRef CAS.
- H. Kuo, S. Chen and A. Yeh, J. Agric. Food Chem., 2014, 62, 742–749 CrossRef CAS.
- H. Yoon and M. Kim, J. Texture Stud., 2010, 38, 393–403 CrossRef.
- A. D. Murugkar, J. Food Sci. Technol., 2015, 52, 2886–2893 CrossRef.
- J. Shin, J. Exercise Nutr. Biochem., 2015, 19, 297–302 CrossRef.
- Y. Lv, H. Song, X. Li, L. Wu and S. Guo, J. Food Sci., 2011, 76, S20–S25 CrossRef CAS.
- N. Alizadeh-Pasdar and E. C. Li-Chan, J. Agric. Food Chem., 2000, 48, 328–334 CrossRef CAS.
- F. Zuo, Z. Chen, X. Shi, R. Wang and S. Tang, Food Chem., 2016, 213, 561–566 CrossRef CAS.
- R. M. García-Estepa, E. Guerra-Hernández and B. García-Villanova, Food Res. Int., 1999, 32, 217–221 CrossRef.
- C. Lisa, R. Elisabeth Viktoria, W. O. Gisela, S. Irmengard, G. Heinrich, S. Regine and D. Stefano, Food Chem., 2019, 299, 125038 CrossRef.
- J. Hu, S. Nie, F. Min and M. Xie, Carbohydr. Polym., 2013, 92, 1143–1150 CrossRef CAS.
- Y. Yang, S. W. Cui, J. Gong, Q. Guo, Q. Wang and Y. Hua, Food Hydrocolloids, 2015, 48, 155–164 CrossRef CAS.
- A. P. Gandhi and M. C. Bourne, Int. J. Food Sci. Technol., 1991, 26, 117–121 CrossRef.
- A. M. Nik, S. Tosh, V. Poysa, L. Woodrow and M. Corredig, Food Res. Int., 2008, 41, 286–294 CrossRef.
- J. Li, S. Matsumoto, A. Nakamura, H. Maeda and Y. Matsumura, Biosci., Biotechnol., Biochem., 2009, 73, 2568–2575 CrossRef CAS.
- M. Tirado-Miranda, A. Schmitt, J. Callejas-Fernández and A. Fernández-Barbero, Eur. Biophys. J., 2003, 32, 128–136 CrossRef CAS.
- Z. Liu and S. K. C. Chang, J. Agric. Food Chem., 2004, 52, 3405–3411 CrossRef CAS.
- R. Wang, J. Liu and S. Guo, Food Hydrocolloids, 2018, 84, 368–378 CrossRef CAS.
- L. Deng, Y. Li, X. Dong, Y. Wang and J. Pan, Trans. Chin. Soc. Agric. Eng., 2014, 30, 307–316 CAS.
- L. Wen, M. Lyu, H. Xiao, H. Lan, Z. Zuo and Z. Yin, Mol. Pharmaceutics, 2018, 15, 2257–2267 CrossRef CAS.
- O. Tomotada, C. M. Rak, I. Ako and O. Satoshi, Agric. Biol. Chem., 1991, 55, 2291–2297 Search PubMed.
- E. Y. Chi, S. Krishnan, T. W. Randolph and J. F. Carpenter, Pharm. Res., 2003, 20, 1325–1336 CrossRef CAS.
- J. L. Mession, M. L. Chihi, N. Sok and R. Saurel, Food Hydrocolloids, 2015, 46, 233–243 CrossRef CAS.
- J. Slavin, J. Am. Diet. Assoc., 1991, 91, 816–819 CAS.
- A. Nawrocka, M. Szymańska-Chargot, A. Miś, A. Z. Wilczewska and K. H. Markiewicz, Food Chem., 2017, 231, 51–60 CrossRef CAS.
- N. Bernat, M. Cháfer, J. Rodríguez-García, A. Chiralt and C. González-Martínez, LWT--Food Sci. Technol., 2015, 62, 488–496 CrossRef CAS.
- K. Jean, M. Renan, M. H. Famelart and F. Guyomarc'h, Int. Dairy J., 2006, 16, 303–315 CrossRef CAS.
- C. Ren, L. Tang, M. Zhang and S. Guo, J. Agric. Food Chem., 2009, 57, 1921–1926 CrossRef CAS.
- A. Nakamura, N. Fujii, J. Tobe, N. Adachi and M. Hirotsuka, Food Hydrocolloids, 2012, 29, 75–84 CrossRef CAS.
- B. T. Lim, J. M. Deman, L. Deman and R. I. Buzzell, J. Food Sci., 1990, 55, 1088–1092 CrossRef.
- J. M. Kovacs, C. T. Mant and R. S. Hodges, Biopolymers, 2006, 84, 283–297 CrossRef CAS.
- B. Yu Zaslavsky, N. M. Mestechkina, L. M. Miheeva and S. V. Rogozhin, J. Chromatogr., A, 1982, 240, 21–28 CrossRef.
- A. Obata and M. Matsuura, Biosci., Biotechnol., Biochem., 1993, 57, 542–545 CrossRef CAS.
- X. Peng, C. Ren and S. Guo, Trends Food Sci. Technol., 2016, 54, 138–147 CrossRef CAS.
- C. Bhattacharjee and K. P. Das, FEBS J., 2000, 267, 3957–3964 CAS.
- L. K. Creamer, A. Bienvenue, H. Nilsson, M. Paulsson, M. van Wanroij, E. K. Lowe, S. G. Anema, M. J. Boland and R. Jiménez-Flores, J. Agric. Food Chem., 2004, 52, 7660–7668 CrossRef CAS.
- C. Chen, Z. Wang, Y. Shi and H. Zhao, China Oils Fats, 2006, 31, 16–19 Search PubMed.
- A. T. James and A. Yang, Food Chem., 2015, 194, 284–289 CrossRef.
- J. T. Tzen, Y. K. Lai, K. L. Chan and A. H. Huang, Plant Physiol., 1990, 94, 1282–1289 CrossRef CAS.
- A. H. Huang, Plant Physiol., 1992, 43, 177–200 CAS.
- E. Çakir and E. A. Foegeding, Food Hydrocolloids, 2011, 25, 1538–1546 CrossRef.
- C. Schmitt, C. Sanchez, S. Desobry-Banon and J. Hardy, Crit. Rev. Food Sci. Nutr., 1998, 38, 689–753 CrossRef CAS.
- X. Lu, Y. Cheng and L. Li, Trans. Chin. Soc. Agric. Mach., 2010, 41, 128–132 CAS.
- N. Rezaei-Ghaleh, H. Ramshini, A. Ebrahim-Habibi, A. A. Moosavi-Movahedi and M. Nemat-Gorgani, Biophys. Chem., 2008, 132, 23–32 CrossRef CAS.
- C. Huang, H. Lin, C. Chou, W. Kao, W. Chou and H. Lee, PLoS One, 2016, 11, e0145957 CrossRef.
- D. A. Kwon, S. Park, D. Kwon, K. H. Kim, B. C. Oh and J. H. Auh, Food Sci. Biotechnol., 2014, 23, 1067–1072 CrossRef CAS.
- T. Koide and T. Ikenaka, Eur. J. Biochem., 2010, 32, 417–431 CrossRef.
- K. G. Duodu, A. Nunes, I. Delgadillo, M. L. Parker, E. N. C. Mills, P. S. Belton and J. R. N. Taylor, J. Cereal Sci., 2002, 35, 161–174 CrossRef CAS.
- M. Saiz-Abajo, C. Gonzalez-Ferrero, A. Moreno-Ruiz, A. Romo-Hualde and C. J. Gonzalez-Navarro, Food Chem., 2013, 138, 1581–1587 CrossRef CAS.
|
This journal is © The Royal Society of Chemistry 2020 |