DOI:
10.1039/D0RA07551E
(Paper)
RSC Adv., 2020,
10, 39673-39686
Anti-inflammatory activity of a water-soluble polysaccharide from the roots of purple sweet potato
Received
3rd September 2020
, Accepted 9th October 2020
First published on 29th October 2020
Abstract
In this study, a water-soluble polysaccharide was isolated from purple sweet potato roots. The in vitro and in vivo anti-inflammatory effects of the polysaccharide were evaluated by lipopolysaccharide (LPS)-induced inflammatory RAW264.7 macrophages and mice, respectively. The in vitro anti-inflammatory assay showed that the polysaccharide could effectively inhibit the overproduction of nitric oxide and pro-inflammatory cytokines (TNF-α, IL-1β, IL-6) while increasing the secretion of anti-inflammatory cytokine (IL-10). The in vivo anti-inflammatory assay revealed that mice administered with the polysaccharide showed higher IL-10, SOD, and T-AOC levels but lower TNF-α, IL-1β, IL-6 and MDA levels as compared to the LPS-treated model. Meanwhile, mice administered with the polysaccharide showed increased abundance of Lachnospiraceae, Lactobacillales and Parabacteroides but decreased amounts of Psychrobacter and Staphylococcus as compared to the LPS model group. Moreover, mice administered with polysaccharide showed enhanced production of short chain fatty acids by gut microbiota in the lipopolysaccharide-induced inflammatory mice. Our results suggested that the water-soluble polysaccharide from purple sweet potato roots could be utilized as a novel anti-inflammatory agent.
1. Introduction
Macrophages can fight off inflammation and infection, playing an essential role in both innate and adaptive immune responses.1 Macrophages are generally categorized into two main phenotypes: classically activated (M1) macrophages and alternatively activated (M2) macrophages.2 Macrophages can be stimulated by interferons (IFN) or lipopolysaccharides (LPS), resulting in the secretion of pro- or anti-inflammatory cytokines. M1 macrophages exhibit pro-inflammatory activity by producing tumor necrosis factor (TNF)-α, interleukin (IL)-1β, IL-6 and nitric oxide (NO), while M2 macrophages exhibit anti-inflammatory activity via signature molecules of IL-10. An imbalance in M1–M2 polarization can lead to the development of multifarious acute or chronic inflammatory diseases, such as arthritis and asthma.3,4 However, natural polysaccharides can induce RAW264.7 macrophages to express IL-10 and change macrophages into M2 phenotypes to maintain body health.5 Therefore, macrophages are often used as cell models to evaluate inflammatory responses.
As a complex glycolipid, LPS is the main ingredient of the cytomembrane in Gram-negative bacteria. LPS can trigger inflammatory responses in various eukaryotic species ranging from insects to humans by initiating signal pathways and promoting target proteins.6,7 LPS can motivate a range of intercellular signaling pathways associated with inflammation, such as nuclear factor-κB (NF-κB) and mitogen-activated protein kinase (MAPK) pathways. Stimulation of these pathways facilitate the expression of genes associated with inflammatory response factors.8,9 TNF-α is initially released during the inflammatory processes, then accelerates the migration of neutrophils to damaged areas resulting in excessive reactive oxygen species (ROS) production.10 However, excessive accumulation of ROS can lead to the development of inflammation-related diseases. At the same time, IL-1β and IL-6 can accelerate the release of inflammatory mediators by motivating accessorial inflammatory cells. Taken together, strengthening the antioxidant capacity against ROS and inhibiting the expression of pro-inflammatory cytokines are both critical for the treatment of LPS-induced inflammation.11,12
The gastrointestinal tract of mammals inhabit a dynamic and complex microbial community comprising the intestinal flora.13 The relationship of reciprocal symbiosis between microflora and the host is key to maintain the homeostatic balance of intestinal microbiota.14 Intestinal dysregulation is related to the pathogenesis of some inflammatory diseases or infections.15 As reported, plant-originated polysaccharides can not only improve intestinal microbiota composition but also regulate intestinal immunity.16 Purple sweet potato, belonging to the family of Convolvulaceae, is one type of sweet potato. Tang et al. found that three kinds of crude polysaccharides extracted from purple sweet potato possessed immunoregulation activities by reducing opportunistic pathogens and proliferating beneficial bacteria.17 Moreover, intestinal microbiota can generate short chain fatty acids (SCFAs) by metabolizing polysaccharides.18 The impact of gut microbiota on the host has been revealed to be mediated in part by SCFAs. Existing studies have documented that SCFAs can suppress histone deacetylases (HDACs) or induce G protein-coupled receptors (GPRs).19,20
In our previous work, a water-soluble polysaccharide (named WPSPP-1) was purified from purple sweet potato.21 Structural characterization showed WPSPP-1 was mainly constituted by 1,4-linked-α-Glcp, 1,6-linked-α-Glcp, 1,4,6-linked-α-Glcp and 1,2-linked-α-Manp, and the branch terminated with β-Glcp. Its molecular weight was determined by high performance gel permeation chromatography (HPGPC) as 1.03 × 104 Da. Moreover, WPSPP-1 was proved to ameliorate mice colitis inflammation induced by dextran sulfate sodium (DSS).21 Apart from chemical-induced colitis, toll-like receptor 4 (TLR4) ligand LPS can also contribute to the onset of intestinal and systemic inflammation. In this study, we further evaluated the in vitro and in vivo anti-inflammatory effect of WPSPP-1 on LPS-induced inflammatory RAW264.7 macrophages and mice, respectively. Moreover, the effects of WPSPP-1 on gut microbiota and SCFA production in LPS-induced inflammatory mice were also determined.
2. Materials and methods
2.1. Materials and reagents
Purple sweet potato roots were provided by the Sweet Potato Research Institute, Chinese Academy of Agricultural Sciences (Jiangsu, China). Fetal bovine serum (FBS) and Dulbecco's modified Eagle medium (DMEM) were obtained from Gibco/Invitrogen Co. (Carlsbad, CA). LPS was purchased from Sigma Chemicals Company (St. Louis, USA). Enzyme-linked immunosorbent assay (ELISA) kits used for the determination of TNF-α, IL-1β, IL-6 and IL-10 were from Nanjing SenBeiJia Biological Technology Co., Ltd (Nanjing, China). The commercial kits used for assaying the activities of superoxide dismutase (SOD), total antioxidant capacity (T-AOC) and malondialdehyde (MDA) level were purchased from Nanjing Jiancheng Bioengineering Institute (Jiangsu, China). All other reagents and chemicals were analytical grade.
2.2. Extraction and purification of WPSPP-1
WPSPP-1 was extracted based on our previously established method.21 Briefly, lyophilized purple sweet potato roots was powdered, degreased with 95% alcohol at 80 °C and then leached at hot water (70 °C) for 2 h. The obtained crude extract was firstly decolorized with AB-8 macroporous resin, and then fractionated on a DEAE-52 ion-exchange column and Sephadex G-100 gel column. The resultant fraction was collected, concentrated and lyophilized to obtain a purified water-soluble polysaccharide, namely WPSPP-1.
2.3. Measurement of the cytotoxicity of WPSPP-1
RAW264.7 cells (CBP No. 74098) were obtained from Shanghai Institute of Cell Biology and Biochemistry of Chinese Academy of Sciences (Shanghai, China). The cells were maintained in DMEM along with penicillin (100 U mL−1), streptomycin (100 μg mL−1) and 10% (v/v) FBS, and were cultured at 37 °C in an atmosphere with 5% CO2.22 The cytotoxicity of WPSPP-1 was determined by the MTT method.23 In short, RAW264.7 macrophages were cultured with a density of 1 × 105 cells per well in 96-well plates and incubated at 37 °C overnight. Then, the cells were pre-treated with various concentrations (25, 50, 100, 200, 400, 800, 1000, 2000, and 4000 μg mL−1) of WPSPP-1 for 24 h. Afterwards, 10 μL of 5 mg mL−1 MTT solution was supplemented to each well of 96-well plates and the cells were incubated for 4 h. Subsequently, the formed formazan crystals were completely dissolved by addition of 100 μL dimethyl sulfoxide. Finally, the absorbance of 96-well plates was recorded at 570 nm by a microplate spectrophotometer.
2.4. In vitro anti-inflammatory activity of WPSPP-1
Briefly, RAW264.7 cells with a density of 1 × 105 cells per well were cultured in 96-well plates at 37 °C for 24 h. Then, the cells were pre-treated with WPSPP-1 at different concentrations (100, 200, 400 and 800 μg mL−1) and 1 μg mL−1 of LPS, and the cells were then incubated at 37 °C for another 24 h. The supernatant was collected and the levels of inflammatory mediator (NO) and cytokines (TNF-α, IL-1β, IL-6 and IL-10) were determined according to the instructions of commercial ELISA kits.12,24
2.5. In vivo anti-inflammatory activity of WPSPP-1
2.5.1. Animal experiments design. The in vivo anti-inflammatory activity of WPSPP-1 was evaluated by a previously reported method.25 ICR mice were purchased from the Comparative Medical Center of Yangzhou University (Yangzhou, China). All mice were housed under controlled environmental conditions at 25 °C and a 12 h light–darkness cycle. Water and standard chow were freely available for mice. All animal procedures were performed in accordance with the Guidelines for Care and Use of Laboratory Animals of Yangzhou University and approved by the Animal Ethics Committee of Yangzhou University (permit no. SYXK2016-0019).To estimate the effect of WPSPP-1 on the LPS-induced inflammatory mice model, forty-eight mice were randomly sorted into four groups (twelve mice per group), including a normal control group treated with 0.9% (w/v) of saline solution, The LPS model group was treated with 2 mg kg−1 of LPS, one sample treatment group was treated with 400 mg kg−1 of WPSPP-1 and 0.9% (w/v) of saline solution, and another sample treatment group was treated with 400 mg kg−1 of WPSPP-1 and 2 mg kg−1 of LPS. Mice in the two LPS treatment groups were intraperitoneally injected with LPS for three days, while mice in the other two groups were intragastrically administered with 0.9% (w/v) of saline solution. Mice feces in the four treatment groups were separately collected and stored at −80 °C. After consecutive gavage for 28 days, mice were sacrificed and their blood, thymus, spleen and liver were quickly collected.
2.5.2. Measurement of immune organ index. The thymus and spleen indexes were calculated according to eqn (1): |
Thymus or spleen index = thymus or spleen weight (mg)/body weight (g)
| (1) |
2.5.3. Measurement of inflammatory cytokines. Blood samples were centrifuged (10
000g, 4 °C) to afford serum. The contents of TNF-α, IL-1β, IL-6 and IL-10 in the serum were measured with ELISA kits based on instructions.
2.5.4. Measurement of hepatic antioxidant and lipid levels. The activities of SOD, T-AOC and MDA in hepatic tissues were assayed according to the manufacturer's instructions (Jiancheng Institute of Biotechnology, Nanjing, China).
2.6. SCFAs analysis
The concentrations of SCFAs including acetate, propionate and butyrate in the feces of mice were measured according to a previous report with some modifications.20 Briefly, fecal samples were pre-treated by deionized water and 10% sulphuric acid, then SCFAs were extracted with diethyl ether. SCFAs in the supernatant were analyzed by a Thermo Trace DSQ II GC-MS System (Thermo Fisher Scientific, USA) with a DB-Wax capillary column (30 m × 0.25 mm × 0.25 μm). The initial column temperature was set at 100 °C for 1 min, then raised to 120 °C at 5 °C min−1, and finally reached 260 °C at 2 °C min−1. The flow rate of carrier gas (helium) was 1.3 mL min−1. For mass spectra, the ionization energy and electron multiplier voltage was 70 eV and 500 V, respectively. Quantitative analysis was conducted according to the peak areas of internal compound (caprylic acid methyl ester).
2.7. 16S rRNA gene sequencing analysis
Total microbial DNA from mice feces was prepared with the TIANamp Stool DNA Extraction kit. PCR was adopted for amplifying the V3–V4 regions of the bacterial 16S rRNA with a forward primer and a reverse primer. A paired-end method was introduced to identify operational taxonomic units (OTUs) after the library was quantified. Sequencing analysis was performed by Majorbio Bio-pharm Technology Co., Ltd (Shanghai, China).
2.8. Statistical analysis
All data were presented as mean ± standard deviation (SD). Statistical significance was estimated by one-way analysis of variance (ANOVA), which was followed by Duncan test using SPSS software (Version 19.0). Differences were considered statistically significant if p < 0.05.
3. Results and discussion
3.1. Extraction and purification of WPSPP-1
Water-soluble polysaccharides were extracted from freeze-dried purple sweet potato at 70 °C for 2 h. The resultant crude polysaccharides were purified by a DEAE-52 ion exchange column and Sephadex G-100 gel column to obtain a purified polysaccharide, named WPSPP-1. GC-MS, 1D NMR, and 2D NMR were employed to investigate the structure of WPSPP-1. Our previous study showed that WPSPP-1 was composed of glucose and mannose in the molar ratio of 20.44
:
1.00 (Fig. 1).21
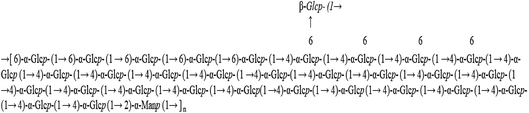 |
| Fig. 1 Structure of WPSPP-1 from purple sweet potato with a molecular weight of 103 kDa. | |
3.2. Cytotoxicity of WPSPP-1
In this study, the cytotoxic effect of WPSPP-1 on normal RAW264.7 macrophages was determined by the MTT assay. The cell viability of RAW264.7 macrophages treated with WPSPP-1 is shown in Fig. 2A. At the concentration of 25–800 μg mL−1, WPSPP-1 was non-toxic to RAW264.7 macrophages. However, the cell viability of RAW264.7 macrophages significantly increased when the concentration of WPSPP-1 increased from 800 to 4000 μg mL−1 (p < 0.05).
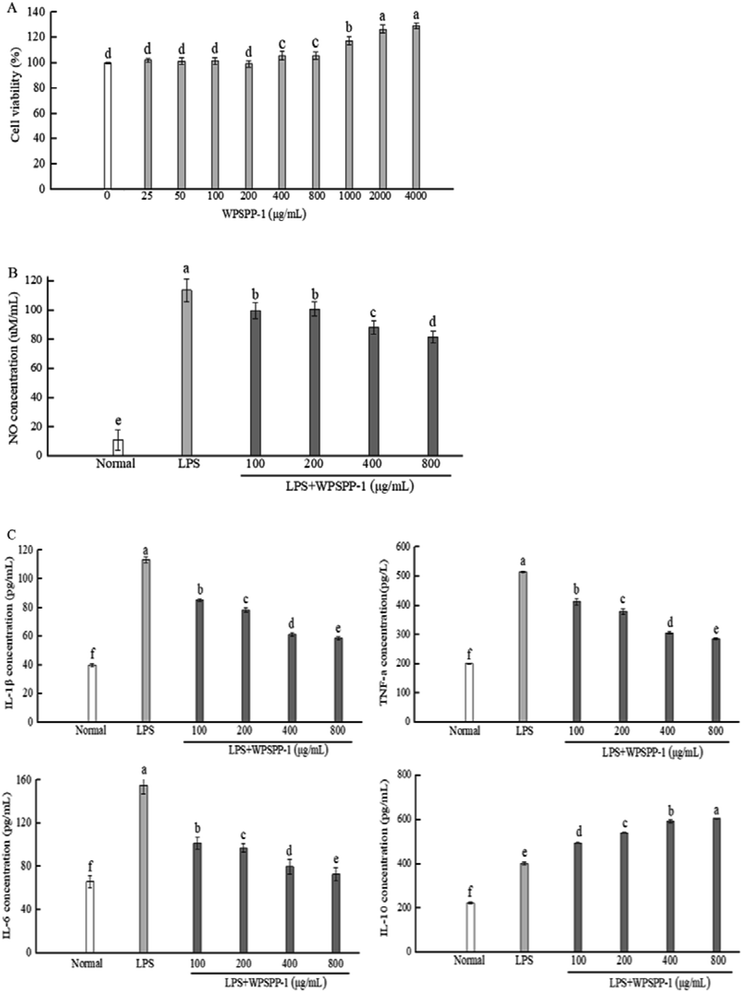 |
| Fig. 2 The cytotoxicity of WPSPP-1 on normal RAW264.7 macrophages (A) and the effect of WPSPP-1 on NO production (B) and IL-1β, IL-6, TNF-α and IL-10 levels (C) in LPS-induced inflammatory RAW264.7 macrophages. Data are expressed as mean ± SD (n = 3). Different lowercase alphabet letters are significantly different at the level of p < 0.05. | |
3.3. In vitro anti-inflammatory activity of WPSPP-1
3.3.1. NO production in LPS-stimulated RAW264.7 macrophages. In LPS-induced macrophages, NO production is considered a representative signal of ordinary inflammatory responses. As shown in Fig. 2B, 1.0 μg mL−1 of LPS significantly stimulated the release of NO in RAW264.7 cells. WPSPP-1 reduced the NO level in a dose-dependent manner. In a previous study, Wang et al. found that 150 μg mL−1 of neutral polysaccharide from North American ginseng could also suppress LPS-stimulated NO overproduction.26
3.3.2. Cytokines secretion in LPS-stimulated RAW264.7 macrophages. Cytokines secreted by RAW264.7 cells can regulate immunity.27 TNF-α plays a pro-inflammatory role in the immune system. IL-1β is concerned with fever during the induction of acute phase protein response. IL-6, generated by monocytes and macrophages, can promote the proliferation and differentiation of B cells and T cells. IL-10 is a major anti-inflammatory cytokine with an immunosuppressive effect.28–30 Compared with the normal control group, the levels of TNF-α, IL-1β, IL-6, and IL-10 were significantly elevated (p < 0.05) in the LPS treatment group (Fig. 2C), indicating that LPS successfully induced RAW264.7 cells to release inflammatory cytokines. As compared to the LPS treatment group, the WPSPP-1 treatment group showed dose-dependent decreased TNF-α, IL-1β and IL-6 levels but increased IL-10 level in RAW264.7 cells (p < 0.05). Our study showed that WPSPP-1 could inhibit the expression of pro-inflammatory cytokines, while stimulating the expression of anti-inflammatory cytokines in a dose-dependent manner.Under normal circumstance, T helper (Th) 1 cytokines and Th2 cytokines regulate relative homeostasis. However, various stimulus-mediated disruptions of Th1 and Th2 immune responses may result in the onset and development of several disorders. Severe Th1 immune responses are involved in the initiation of inflammatory bowel disease (IBD), whereas abnormal Th2 immune responses participate in the development of asthma.31 Plant-derived polysaccharides, such as Astragalus polysaccharides, could reduce the secretion of Th1 and modulate the imbalance between Th1 and Th2 in inflammatory disorders.32 Our results showed that overproduction of Th1 cytokine IL-1β and Th2 cytokine IL-6 was suppressed by WPSPP-1 treatment, suggesting that WPSPP-1 had the potential to regulate the immune response balance between Th1 and Th2 in LPS-stimulated RAW264.7 macrophages. In this study, WPSPP-1 treatment was proven to boost the secretion of anti-inflammatory cytokine IL-10 in LPS-treated macrophages. Previous literature also elucidated that IL-10 possessed the ability to decrease excessive pro-inflammatory cytokines (TNF-α, IL-1β and IL-6).33 Thus, the improvement of IL-10 secretion contributed to the anti-inflammatory activity of WPSPP-1.
Our results showed that high concentrations of WPSPP-1 (more than 400 μg mL−1) exhibited better anti-inflammatory effects in LPS-stimulated RAW264.7 macrophages. Although there was no significant differences between 400 μg mL−1 and 800 μg mL−1 of WPSPP-1 on the cell viability of RAW264.7 cells (Fig. 2A), a high dose of sweet potato polysaccharide might decrease the abundance of gut microbiota.34 So 400 mg kg−1 of WPSPP-1 was used in in vivo experiments.
3.4. In vivo anti-inflammatory effect of WPSPP-1
3.4.1. Immune organ index of LPS-induced inflammatory mice. The effect of WPSPP-1 on the immune organ indices (thymus and spleen indexes) of mice is shown in Fig. 3A. No significant differences were observed in thymus and spleen indices between the normal control group and WPSPP-1 treatment group, revealing that WPSPP-1 had no side-effect on the immune organ index of normal mice. Compared with the normal control group, the thymus index of mice in the LPS treatment group was significantly reduced, but the spleen index in the LPS treatment group was significantly increased (p < 0.05), indicating that the LPS-induced inflammation model was successfully established. As compared with the LPS model group, the thymus index increased while the spleen index decreased in the WPSPP-1 + LPS treatment group (p < 0.05). These results suggested that WPSPP-1 possessed a positive effect on improving the immune organs of inflammatory mice. Similar effects were also found in inflammatory mice administered with sulfated Cyclocarya paliurus polysaccharide and Camellia nitidissima polysaccharide.35,36
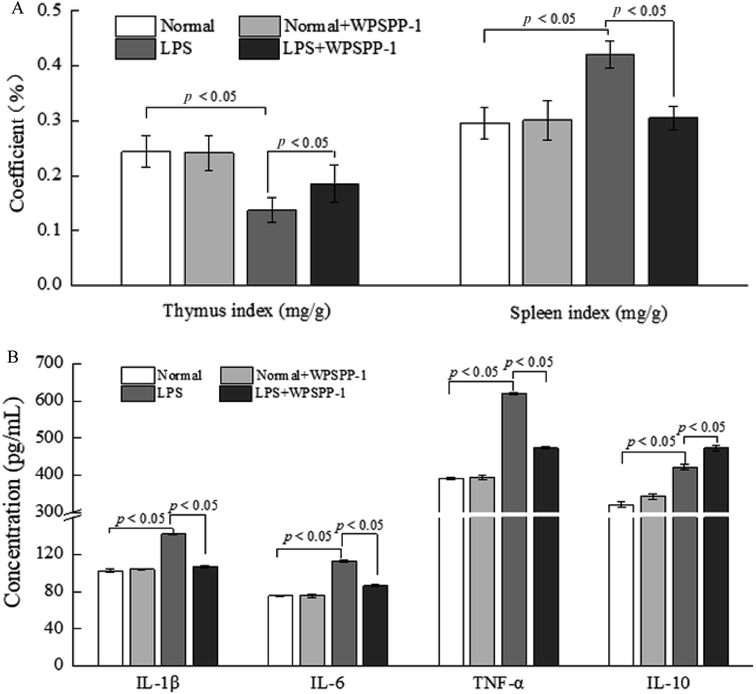 |
| Fig. 3 Effect of WPSPP-1 on immune organ (thymus and spleen) indices (A) and the secretion of cytokines (B) in LPS-induced inflammatory mice. Data are expressed as mean ± SD (n = 10). Significant difference is presented at the level of p < 0.05 among different groups. | |
3.4.2. Cytokines levels in LPS-induced inflammatory mice. To investigate the anti-inflammatory effect of WPSPP-1 on LPS-treated mice, the levels of serum cytokines were evaluated. The inflammatory cytokines, such as TNF-α, IL-1β and IL-6, arise at early stages of the inflammatory response that can contribute to the defense against harmful stimuli. LPS can stimulate overproduction of inflammatory cytokines, leading to excessive inflammatory responses.37 The imbalance between pro-inflammatory cytokines and anti-inflammatory cytokines is a hallmark of the pathogenesis of inflammation.38 The anti-inflammatory effect of WPSPP-1 on LPS-induced inflammatory mice is shown in Fig. 3B. As compared to the normal control group, the levels of IL-1β, IL-6, TNF-α and IL-10 were significantly increased in the LPS model group (p < 0.05). However, as compared with the LPS model group, the levels of pro-inflammatory cytokines (TNF-α, IL-1β and IL-6) decreased while the level of IL-10 increased in the LPS + WPSPP-1 treatment group. In a previous study, a water-soluble polysaccharide isolated from Sedum dendroideum exhibited anti-inflammation activity through reducing IL-1β and TNF-α production.39 Another study also found that the alkali-soluble polysaccharide from purple sweet potato significantly restrained pro-inflammatory agents while enhancing anti-inflammatory mediators.40 These results indicated that WPSPP-1 had potential in vivo anti-inflammatory activity.
3.4.3. Levels of SOD, T-AOC and MDA in LPS-induced inflammatory mice. The inflammatory reaction induced by LPS may give rise to oxidative stress. The overproduction of ROS can destroy the antioxidant defense system and eventually exacerbate the damage of organs.41,42 SOD is the forefront of the antioxidant enzyme system against ROS, which converts superoxide into oxygen and hydrogen peroxide.43 As for the T-AOC, it is a non-enzymatic antioxidant indicator of the defense system in organs.44 As presented in Fig. 4, the SOD and T-AOC levels in liver tissue were strikingly decreased but the MDA level was distinctly elevated in the LPS model group as compared with the normal control group (p < 0.05). However, as compared with the LPS model group, the T-AOC and SOD levels were enhanced after LPS + WPSPP-1 treatment. Meanwhile, the MDA level was significantly decreased by LPS + WPSPP-1 treatment (p < 0.05). A previous study also showed that LPS damaged the endogenous antioxidant defense system (e.g. SOD and T-AOC) and increased the production of MDA.45 Increasing evidence demonstrated that polysaccharides ameliorated LPS-induced oxidative damages.37,46–48 Our results further demonstrated that WPSPP-1 could alleviate LPS-induced inflammatory and oxidative damages.
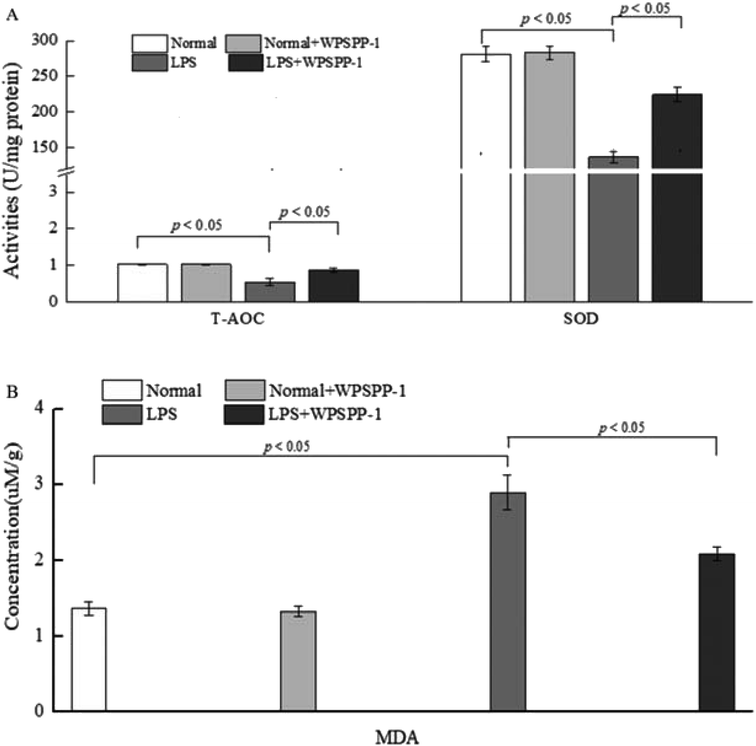 |
| Fig. 4 Effect of WPSPP-1 on T-AOC and SOD activity (A) and MDA level (B) in LPS-induced inflammatory mice. Data are expressed as mean ± SD (n = 10). Significant difference is presented at the level of p < 0.05 among different groups. | |
3.5. SCFAs content in LPS-induced inflammatory mice
SCFAs are the by-products of dietary fibre fermented by the intestinal microorganisms.49 A large proportion of ingested foods including dietary fibers, resistant starch, proteins and fats cannot be digested and absorbed in the small intestine and finally reach the colon.50 Most of these unabsorbed compounds are fermented by gut microbiota in the colon. Intestinal flora degrade undigested polysaccharides to produce various SCFAs, such as acetate, propionate, and butyrate. These SCFAs are easily absorbed and can be utilized by colonic epithelial cells as sources of energy and carbon.51 As shown in Fig. 5, acetic acid (AA), propionic acid (PA) and butyric acid (BA) were all dramatically reduced in the LPS model group as compared with the normal control group (p < 0.05). However, the WPSPP-1 + LPS treatment group effectively promoted SCFA production as compared to the LPS model group (p < 0.05). Numerous studies have revealed that SCFAs have the potential to suppress inflammatory responses. On one hand, SCFAs can reduce the translocation of inflammatory stimulus into systemic circulation by strengthening the intestinal epithelial barrier. On the other hand, SCFAs can act on FOXP3+ regulatory T cells to produce anti-inflammatory factors TGF-β and IL-10 and inactivate the NF-κB signaling pathway.52 Therefore, WPSPP-1 might ameliorate LPS-induced inflammation partly through promoting SCFA production. Previous studies showed that linear polysaccharides, such as inulin, xylan and glucan, could elevate SCFAs levels in animals or humans under inflammatory conditions.53,54 Our results suggested WPSPP-1 had a similar effect on SCFAs production in LPS-induced inflammatory mice.
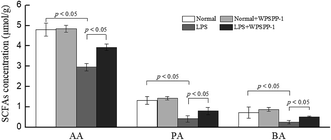 |
| Fig. 5 Effect of WPSPP-1 on the content of SCFAs (AA, PA and BA) in mice. AA, acetic acid; PA, propionic acid; BA, butyric acid. Data are expressed as mean ± SD (n = 7). Significant difference is presented at the level of p < 0.05 among different groups. | |
3.6. Diversity of gut microbiota in LPS-induced inflammatory mice
OTUs can reflect the microbial diversity and abundance of different microorganisms in the gut.55 The total number of OTUs in four treatment groups are displayed in Fig. 6A. The total number of OTUs in the LPS model group was significantly reduced as compared with the normal control group. However, LPS-treated mice administered with WPSPP-1 had a distinctly elevated total number of OTUs as compared to the LPS model group. These indicated that administration of LPS had a negative effect while WPSPP-1 had a positive effect on gut microbiota. The same total number of OTUs in these four groups was 585, indicating that most of the bacteria were the core microbiota in these groups.
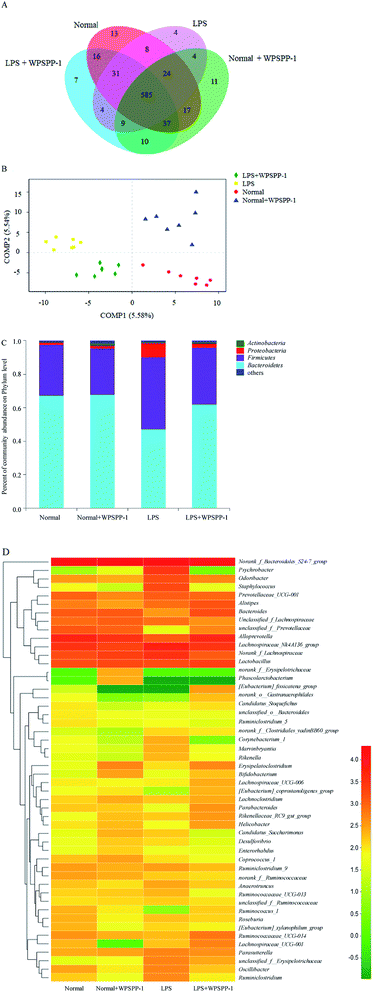 |
| Fig. 6 Effects of WPSPP-1 on the gut microbiota compositions and relative abundances in mice. (A) Venn diagram, (B) PCA, (C) relative abundances of phylum level, (D) heat map of relative abundances on genus level. | |
The whole structural changes of gut microbiota in four treatment groups were described by the unsupervised multivariate statistical method. As shown in Fig. 6B, the normal control group and normal + WPSPP-1 treatment group were separated from LPS and LPS + WPSPP-1 treatment groups in the first component (COMP1). For LPS-treated mice, the whole structure of gut microbiota was obviously altered. By comparison, the composition of intestinal flora in the LPS + WPSPP-1 treatment group was close to that in the normal control group, suggesting that the administration of WPSPP-1 could adjust gut microbiota in LPS-induced inflammatory mice.
Histograms were employed to display the differences in species and relative abundance of gut microbiota among different treatment groups. As shown in Fig. 6C, the phylum-level distribution features of the four treatment groups were distinct. Firmicutes and Bacteroidetes are two predominant populations at the phylum level, accounting for approximately 90% of total microbes, which play important roles in regulating carbohydrate, lipid and bile acid metabolisms.56 When compared to the normal control group, a significant increase in the relative abundance of Firmicutes and a significant decrease in the relative abundance of Bacteroidetes were observed in the LPS model group (p < 0.05) (Table 1). However, mice administered with LPS + WPSPP-1 exhibited an evident decrease in the relative abundance of Firmicutes and a significant increase in the relative abundance of Bacteroidetes as compared to the LPS model group (p < 0.05). In addition, the Proteobacteria level in the LPS model group was strikingly increased as compared with that of the normal control group. The treatment of WPSPP-1 could strikingly reduce the relative abundance of Proteobacteria in LPS-treated mice. No distinct differences were found in the levels of Bacteroidetes, Firmicutes and Proteobacteria between the WPSPP-1 group and normal control group. The ratio of Bacteroidetes to Firmicutes is often used to evaluate the distribution of gut flora. The increased ratio of Bacteroidetes to Firmicutes is responsible for protecting the host against systemic inflammatory diseases.57 The Bacteroidetes to Firmicutes ratio in the LPS + WPSPP-1 group was increased as compared with that of the LPS model group. The abnormal dilatation of Proteobacteria is generally considered to be a key feature of the intestinal microbiota imbalance.17 WPSPP-1 treatment reduced the level of Proteobacteria in LPS-treated mice. All these results revealed that WPSPP-1 exhibited a potential role in regulating intestinal flora in LPS-treated inflammatory mice.
Table 1 Differences in gut microbiota at phylum levels of each groupa
Group |
Bacteroidetes |
Firmicutes |
Proteobacteria |
Actinobacteria |
One-way ANOVA and Duncan test were used to evaluate significant differences in gut microbiota at phylum levels. Values are presented as mean ± SD (n = 7). Different lowercase alphabet letters are significantly different at the level of p < 0.05. |
Normal |
67.35 ± 5.87a |
30.36 ± 3.77bc |
1.22 ± 0.36c |
0.63 ± 0.21b |
Normal + WPSPP-1 |
67.73 ± 6.04a |
27.76 ± 5.11c |
1.80 ± 0.51c |
1.36 ± 0.43a |
LPS |
47.26 ± 7.31b |
42.96 ± 2.13a |
8.42 ± 1.01a |
0.84 ± 0.26b |
LPS + WPSPP-1 |
61.97 ± 2.45a |
34.07 ± 1.86b |
2.49 ± 0.27b |
0.71 ± 0.19b |
To further verify the differences in the intestinal flora among different treatment groups, the genus level of fecal microflora in different treatment groups was contrasted. As shown in Fig. 6D, the microbial structure of different treatment groups was different. At the genus level, the dominant intestinal flora in four groups mainly included nonrank_f_Bacteroidales S24-7 group, Alloprevotella, Lachnospiraceae NK4A136 group, Norank_f_Lachnospiraceae and Lactobacillus. The relative abundances of Psychrobacter and Staphylococcus were remarkably elevated, while the levels of Bacteroides, Lactobacillius and Parabacteroides were significantly inhibited in LPS-stimulated inflammatory mice as compared with the normal control group (Table 2). However, the relative abundances of Bacteroides, Lactobacillius, Bifidobacterium and Parabacteroides in the LPS + WPSPP-1 treatment group were increased as compared with the LPS model group. Bacteroides are important members of human intestinal microorganisms that bring countless benefits to the host, devoting more than 20% of their genomes to transport and degrade polysaccharides.58,59 Lactobacillus is a member of lactic acid-producing microorganisms that promote health.60 Bifidobacterium can generate butyrate, which provides an anti-inflammatory effect.61 Parabacteroides has been demonstrated to possess metabolic benefits on decreasing weight gain, hyperglycemia and hepatic steatosis in mice fed with high-fat diet.62 Our results indicated that WPSPP-1 might ameliorate inflammation by increasing the relative abundance of Bacteroides, Lactobacillus, Bifidobacterium and Parabacteroides, and thus enhance the content of beneficial metabolites in the feces, such as SCFAs.
Table 2 Differences in gut microbiota at genus levels of each groupa
Group |
Psychrobacter |
Staphylococcus |
Bacteroides |
Lactobacillius |
Bifidobacterium |
Parabacteroides |
One-way ANOVA and Duncan test were used to evaluate significant differences in gut microbiota at genus levels. Values are presented as mean ± SD (n = 7). Different lowercase alphabet letters are significantly different at the level of p < 0.05. |
Normal |
0.03 ± 0.01b |
0.21 ± 0.08b |
3.34 ± 0.91b |
4.52 ± 0.57b |
0.09 ± 0.03c |
0.43 ± 0.13b |
Normal + WPSPP-1 |
0.11 ± 0.03b |
0.06 ± 0.02b |
3.22 ± 0.67b |
4.80 ± 0.72b |
0.74 ± 0.21a |
0.38 ± 0.08b |
LPS |
6.84 ± 1.09a |
4.33 ± 1.35a |
0.81 ± 0.23c |
3.90 ± 0.43c |
0.12 ± 0.04c |
0.14 ± 0.03c |
LPS + WPSPP-1 |
0.01 ± 0.01b |
0.15 ± 0.04b |
4.67 ± 1.16a |
6.55 ± 1.11a |
0.49 ± 0.12b |
1.06 ± 0.35a |
Linear discriminant analysis (LDA) was performed to identify specialized microbial communities. Four treatment groups were shown in cladograms and LDA scores of more than 2 were confirmed by linear discriminant analysis effect size (LEfSe) (Fig. 7). In the LPS model group, eight bacteria were predominant, namely Clostridiales_vadinBB60_group (from family to species), Aerococcaceae (the family and genus of Facklamia to species), Atopostipes (from genus to species), Pseudogracilibacillus (from genus to species), Planococcaceae (the family and genus of Sporosarcina to species), Bacilli (from order to species), Paenalcaligenes (from genus to species), and Psychrobacter (from genus to species). For the LPS + WPSPP-1 treatment group, five bacteria were significantly enriched, namely Lachnospiraceae_UCG_001 (genus), Streptococcaceae (from family to species), Parabacteroides (from order to species), Alphaproteobacteria (the class and order of Rhodospirillales to genus), and Cyanobacteria (the phylum and its class, order of Gastranaerophilales to species). For the normal control group, the bacteria of Anaerovorax (from genus to species) and Alloprevotella (from genus to species) were enriched. But no microbiota were detected at a significant level in the WPSPP-1 treatment group. Therefore, there was an obvious difference in gut microbiota composition among different treatment groups. Notably, WPSPP-1 increased the relative abundance of Lachnospiraceae_UCG_001 and Streptococcaceae. It has been reported that Lachnospiraceae is able to breakdown polysaccharides and produce SCFAs, which might disintegrate WPSPP-1.63 Streptococcaceae belongs to the order of Lactobacillales, which is a dominant bacterial species in the gut and is widely used as a probiotic.64
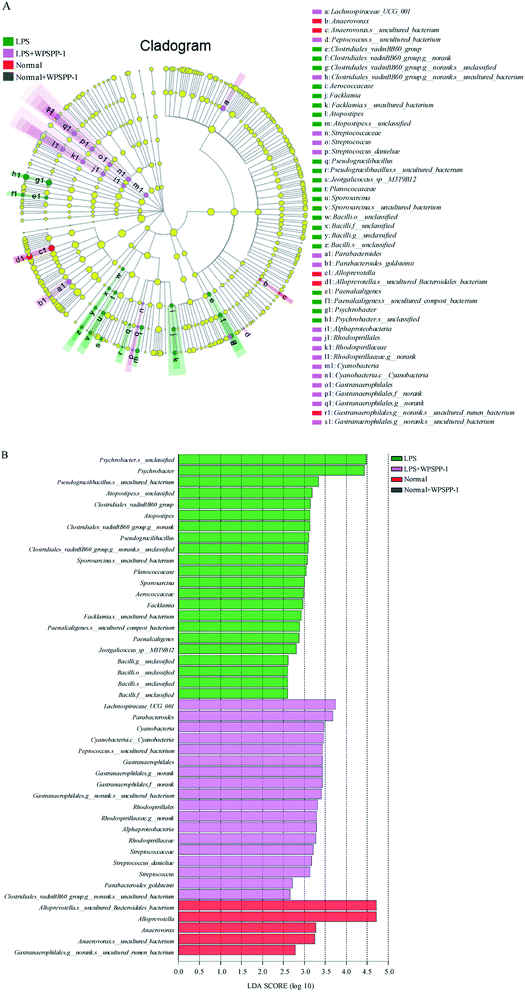 |
| Fig. 7 LEfSe analysis of WPSPP-1 intervention on gut microbiota. (A) Phylogenetic distribution cladogram of the bacterial lineages, (B) LDA scores with 2 or greater of indicator bacteria. Circles indicate phylogenetic levels from domain to genus. The diameter of each circle is proportional to the abundance of the group. | |
A phylogenetic investigation of communities by the reconstruction of unobserved states (PICRUSt) method was adopted to predict metagenomic functions; a total of 262 KEGG pathways were detected in all samples. A significant difference in KEGG pathways is shown in Fig. 8. Compared to the normal control group, 34 functional modules (7 increased and 27 decreased) were obviously detected in the LPS model group (p < 0.05). However, the intervention of WPSPP-1 improved the abundance of these pathways. Metabolic activities of intestinal microbiota were the most important functions in mammals and avians.65 Our results suggested that metabolism accounted for 48.56% on average of KEGG pathways in four different groups. Energy metabolism was remarkably enhanced in the LPS model group, and carbohydrate metabolism, amino acid metabolism, glycan biosynthesis and metabolism, and cofactor and vitamin metabolisms were strikingly elevated in the LPS + WPSPP-1 treatment group (p < 0.05). Besides, WPSPP-1 could lower the disease risk, such as infectious diseases. Nevertheless, it is plausible that microbiota in the LPS model group were involved in maintaining fundamental activities, while microbiota in the LPS + WPSPP-1 treatment group may ameliorate metabolic disorders and inhibit inflammatory reactions.66 Our results suggest that WPSPP-1 could suppress LPS-induced inflammation by improving the gut microbiota composition, enhancing the anti-oxidative defense system and stimulating the production of anti-inflammatory metabolite SCFAs.
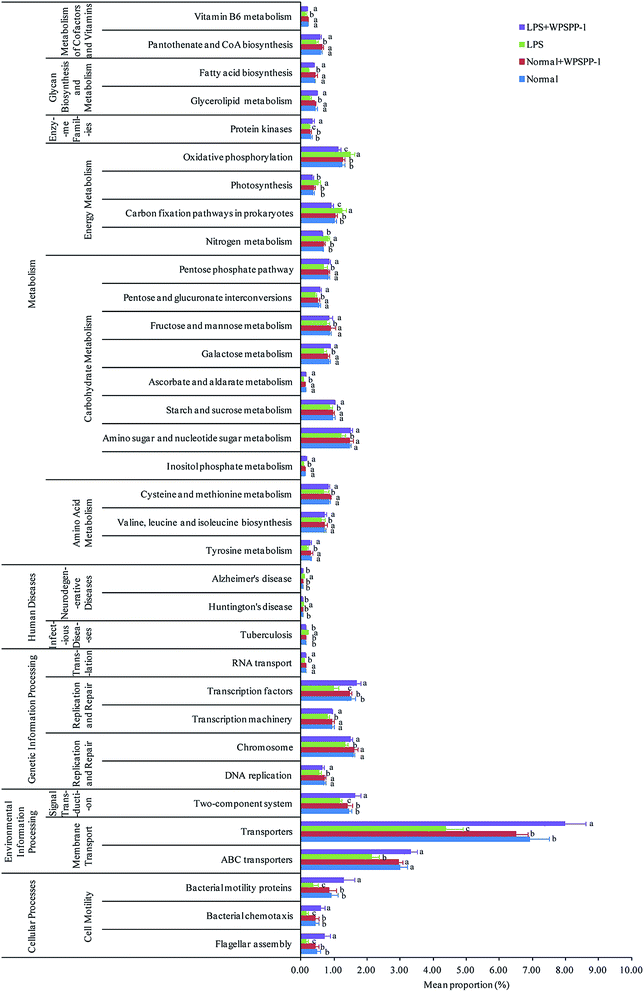 |
| Fig. 8 Effect of WPSPP-1 on gut microbiota function predicted by PICRUSt. Data are expressed as mean ± SD (n = 7). Different lowercase alphabet letters are significantly different at the level of p < 0.05. | |
4. Conclusion
In summary, WPSPP-1 effectively regulated the levels of pro-inflammatory cytokines and anti-inflammatory cytokines, suppressed the growth of Psychrobacter and Staphylococcus, and promoted the growth of health-promoting microorganisms including Lachnospiraceae, Lactobacillales and Parabacteroides. In addition, WPSPP-1 significantly elevated the production of SCFAs in the gut of inflammatory mice and enhanced the anti-oxidative defense system against oxidative stress. Therefore, WPSPP-1 could effectively alleviate LPS-induced inflammation both in vitro and in vivo. In the future, more in-depth studies can be carried out to reveal the anti-inflammatory mechanisms of WPSPP-1 at molecular levels.
Conflicts of interest
The authors declare that there are no conflicts of interest.
Acknowledgements
This work was supported by National Natural Science Foundation of China (No. 31871803 and 31571788), Agriculture Research System of China (CARS-10-B20), 333 High Level Talent Training Project of Jiangsu Province (BRA2019235), Six Major Talent Summit Project of Jiangsu Province (SWYY-224) and Science and Technology Project of Xuzhou City (KC19243).
References
- H. Li, Z. Dong, X. Liu, H. Chen, F. Lai and M. Zhang, Structure characterization of two novel polysaccharides from Colocasia esculenta (taro) and a comparative study of their immunomodulatory activities, J. Funct. Foods, 2018, 42, 47–57 CrossRef CAS.
- L. Chen, B. Gao, Y. Zhang, H. Lu, X. Li, L. Pan and X. Zhi, PAR2 promotes M1 macrophage polarization and inflammation via FOXO1 pathway, J. Cell. Biochem., 2019, 120, 9799–9809 CrossRef CAS.
- L. Cicchitti, M. Martelli and F. Cerritelli, Chronic inflammatory disease and osteopathy: A systematic review, PloS One, 2014, 10, e0121327 CrossRef.
- M. Zhang, W. Wu, Y. Ren, X. Li, Y. Tang, T. Min and H. Wu, Structural characterization of a novel polysaccharide from Lepidium meyenii (maca) and analysis of its regulatory function in macrophage polarization in vitro, J. Agric. Food Chem., 2017, 65, 1146–1157 CrossRef CAS.
- Q. Li, Y. Feng, W. He, L. Wang, R. Wang, L. Dong and C. Wang, Post-screening characterisation and in vivo evaluation of an anti-inflammatory polysaccharide fraction from Eucommia ulmoides, Carbohydr. Polym., 2017, 169, 304–314 CrossRef CAS.
- A. Khan, S. Khan, H. Ali, K. U. Shah, H. Ali, O. Shehzad and Y. S. Kim, Anomalin attenuates LPS-induced acute lungs injury through inhibition of AP-1 signaling, Int. Immunopharmacol., 2019, 73, 451–460 CrossRef CAS.
- Y. Chen, H. Zhang, Y. Cheng, Y. Li, C. Wen and Y. Zhou, Dietary L-threonine supplementation attenuates lipopolysaccharide-induced inflammatory responses and intestinal barrier damage of broiler chickens at an early age, Br. J. Nutr., 2018, 119, 1254–1262 CrossRef CAS.
- P. A. Baeuerle and V. R. Baichwal, NF-κB as a frequent target for immunosuppressive and anti-inflammatory molecules, Adv. Immunol., 1997, 65, 111–138 CAS.
- H. Wu, A. Dai, X. Chen, X. Yang, X. Li, C. Huang and G. Deng, Leonurine ameliorates the inflammatory responses in lipopolysaccharide-induced endometritis, Int. Immunopharmacol., 2018, 61, 156–161 CrossRef CAS.
- Y. Huang, F. Zhou, C. Shen, H. Wang and Y. Xiao, LBP reduces the inflammatory injury of kidney in septic rat and regulates the Keap1-Nrf2/ARE signaling pathway, Acta Cir. Bras., 2019, 34, 1–13 Search PubMed.
- Z. Gao, J. Li, X. Song, J. Zhang, X. Wang, H. Jing and L. Jia, Antioxidative, anti-inflammation and lung-protective effects of mycelia selenium polysaccharides from Oudemansiella radicata, Int. J. Biol. Macromol., 2017, 104, 1158–1164 CrossRef CAS.
- Z. Gao, C. Zhang, H. Liu, Y. Zhu, Z. Ren, H. Jing and L. Jia, The characteristics and antioxidation of Oudemansiella radicata selenium polysaccharides on lipopolysaccharide-induced endo-toxemic mice, Int. J. Biol. Macromol., 2018, 116, 753–764 CrossRef CAS.
- X. Song, D. Dai, X. He, S. Zhu, Y. Yao, H. Gao and Y. Qian, Growth factor FGF2 cooperates with interleukin-17 to repair intestinal epithelial damage, Immunity, 2015, 43, 488–501 CrossRef CAS.
- M. J. A. Saad, A. Santos and P. O. Prada, Linking gut microbiota and inflammation to obesity and insulin resistance, Physiology, 2016, 31, 283–293 CrossRef CAS.
- X. Meng, H. Y. Zhou, H. H. Shen, E. Lufumpa, X. M. Li, B. Guo and B. Z. Li, Microbe-metabolite-host axis, two-way action in the pathogenesis and treatment of human autoimmunity, Autoimmun. Rev., 2019, 18, 455–475 CrossRef CAS.
- L. Chen, W. Xu, D. Chen, G. Chen and H. Zhu, Digestibility of sulfated polysaccharide from the brown seaweed Ascophyllum nodosum and its effect on the human gut microbiota in vitro, Int. J. Biol. Macromol., 2018, 112, 1055–1061 CrossRef CAS.
- C. Tang, J. Sun, B. Zhou, C. Jin, J. Liu and J. Kan, Effects of polysaccharides from purple sweetpotatoes on immune response and gut microbiota composition in normal and cyclophosphamide treated mice, Food Funct., 2018, 9, 937–950 RSC.
- Y. G. Xia, T. L. Wang, S. M. Yu, J. Liang and H. X. Kuang, Structural characteristics and hepatoprotective potential of Aralia elata root bark polysaccharides and their effects on SCFAs produced by intestinal flora metabolism, Carbohydr. Polym., 2019, 207, 256 CrossRef CAS.
- H. Ohira, W. Tsutsui and Y. Fujioka, Are short chain fatty acids in gut microbiota defensive players for inflammation and atherosclerosis, J. Atheroscler. Thromb., 2017, 24, 660–672 CrossRef CAS.
- M. Kobayashi, D. Mikami, H. Kimura, K. Kamiyama, Y. Morikawa, S. Yokoi and M. Iwano, Short-chain fatty acids, GPR41 and GPR43 ligands, inhibit TNF-α-induced MCP-1 expression by modulating p38 and JNK signaling pathways in human renal cortical epithelial cells, Biochem. Biophys. Res. Commun., 2017, 486, 499–505 CrossRef CAS.
- Y. Gou, J. Sun, J. Liu, H. Chen, J. Kan, C. Qian and C. Jin, Structural characterization of a water-soluble purple sweetpotato polysaccharide and its effect on intestinal inflammation in mice, J. Funct. Foods, 2019, 61, 103502 CrossRef CAS.
- C. Tang, J. Sun, B. Zhou, C. Jin, J. Liu, Y. Gou and N. Zhang, Immunomodulatory effects of polysaccharides from purple sweetpotato on lipopolysaccharide treated RAW 264.7 macrophages, J. Food Biochem., 2018, 42, e12535 CrossRef.
- S. Gao, Y. Wang, D. Li, Y. Guo, M. Zhu, S. Xu and G. Fan, TanshinoneIIA alleviates inflammatory response and directs macrophage polarization in lipopolysaccharide-stimulated RAW264. 7 cells, Inflammation, 2019, 42, 264–275 CrossRef CAS.
- E. H. Kim, Y. S. Choi and Y. M. Kim, Antioxidative and anti-inflammatory effect of Phellinus igniarius on RAW 264.7 macrophage cells, J. Exerc. Rehabil., 2019, 15, 2–7 CrossRef.
- M. Zhang, Y. Zhao, N. Wu, Y. Yao, M. Xu and H. Du, The anti-inflammatory activity of peptides from simulated gastrointestinal digestion of preserved egg white in DSS-induced mouse colitis, Food Funct., 2018, 9, 6444–6454 RSC.
- L. Wang, X. Yu, X. Yang, Y. Li, Y. Yao, E. M. K. Lui and G. Ren, Structural and anti-inflammatory characterization of a novel neutralpolysaccharide from North American ginseng (Panax quinquefolius), Int. J. Biol. Macromol., 2015, 74, 12–17 CrossRef CAS.
- J. Wang, H. Wang, H. Zhang, Z. Liu, C. Ma and W. Kang, Immunomodulation of ADPs-1a and ADPs-3a on RAW264.7 cells through NF-κB/MAPK signaling pathway, Int. J. Biol. Macromol., 2019, 132, 1024–1030 CrossRef CAS.
- Y. Liu, C. M. Ho and A. Mak, Interleukin (IL)-6, tumour necrosis factor alpha (TNF-α) and soluble interleukin-2 receptors (sIL-2R) are elevated in patients with major depressive disorder: A meta-analysis and meta-regression, J. Affect. Disord., 2012, 139, 230–239 CrossRef CAS.
- L. Peng, A. Ai-lati, Z. Ji, S. Chen and J. Mao, Polyphenols extracted from huangjiu have anti-inflammatory activity in lipopolysaccharide stimulated RAW264. 7 cells, RSC Adv., 2019, 9, 5295–5301 RSC.
- M. Jiang, W. Huang, Z. Wang, F. Ren, L. Luo, J. Zhou and L. Tang, Anti-inflammatory effects of Ang-(1–7) via TLR4-mediated inhibition of the JNK/FoxO1 pathway in lipopolysaccharide-stimulated RAW264.7 cells, Dev. Comp. Immunol., 2019, 92, 291–298 CrossRef CAS.
- C. Tang, J. Sun, J. Liu, C. Jin, X. Wu, X. Zhang, H. Chen, Y. Gou, J. Kan, C. Qian and N. Zhang, Immune-enhancing effects of polysaccharides from purple sweetpotato, Int. J. Biol. Macromol., 2019, 123, 923–930 CrossRef CAS.
- S. Ullah, A. A. Khalil, F. Shaukat and Y. Song, Sources, extraction and biomedical properties of polysaccharides, Foods, 2019, 8, 304 CrossRef CAS.
- J. L. Stow, P. C. Low, C. Offenhauser and D. Sangermani, Cytokine secretion in macrophages and other cells: Pathways and mediators, Immunobiology, 2009, 214, 601–612 CrossRef CAS.
- M. Liu, X. Li, S. Zhou, T. T. Y. Wang, S. Zhou, K. Yang, Y. Li, J. Tian and J. Wang, Dietary fiber isolated from sweetpotato residues promotes a healthy gut microbiome profile, Food Funct., 2020, 11, 689–699 RSC.
- Z. Wang, J. Xie, Y. Yang, F. Zhang, S. Wang, T. Wu and M. Xie, Sulfated Cyclocarya paliurus polysaccharides markedly attenuates inflammation and oxidative damage in lipopolysaccharide-treated macrophage cells and mice, Sci. Rep., 2017, 7, 40402 CrossRef CAS.
- D. Zou, K. Zhang and A. Xie, Protective effect of Camellia nitidissima polysaccharides on immunological liver injury in themice induced by BCG combined LPS, West China, J. Pharm. Sci., 2014, 29, 525–527 CAS.
- M. Liu, S. Li, X. Wang, Y. Zhu, J. Zhang, H. Liu and L. Jia, Characterization, anti-oxidation and anti-inflammation of polysaccharides by Hypsizygus marmoreus against LPS-induced toxicity on lung, Int. J. Biol. Macromol., 2018, 111, 121–128 CrossRef CAS.
- T. Hu, Q. Lin, T. Guo, T. Yang, W. Zhou, X. Deng and F. Luo, Polysaccharide isolated from Phellinus linteus mycelia exerts anti-inflammatory effects via MAPK and PPAR signaling pathways, Carbohydr. Polym., 2018, 200, 487–497 CrossRef CAS.
- I. Rjeibi, F. Hentati, A. Feriani, N. Hfaiedh, C. Delattre, P. Michaud and G. Pierre, Novel antioxidant, anti-α-amylase, anti-inflammatory and antinociceptive water-soluble polysaccharides from the aerial part of Nitraria retusa, Foods, 2020, 9, 28 CrossRef CAS.
- H. Chen, J. Sun, J. Liu, Y. Gou, X. Zhang, X. Wu and C. Jin, Structural characterization and anti-inflammatory activity of alkali-soluble polysaccharides from purple sweetpotato, Int. J. Biol. Macromol., 2019, 131, 484–494 CrossRef CAS.
- G. Bist, N. T. Pun, T. B. Magar, A. Shrestha, H. J. Oh and A. Khakurel, Inhibition of LPS-stimulated ROS production by fluorinated and hydroxylated chalcones in RAW 264.7 macrophages with structure-activity relationship study, Bioorg. Med. Chem. Lett., 2017, 27, 1205–1209 CrossRef CAS.
- C. H. Chiu, C. C. Chyau, C. C. Chen, C. H. Lin, C. H. Cheng and M. C. Mong, Polysaccharide extract of Cordyceps sobolifera attenuates renal injury in endotoxemic rats, Food Chem. Toxicol., 2014, 69, 281–288 CrossRef CAS.
- F. Wang, J. Liu, L. Zhou, G. Pan, Z. Li and F. Cheng, Senescence-specific change in ROS scavenging enzyme activities and regulation of various SOD isozymes to ROS levels in psf mutant rice leaves, Plant Physiol. Biochem., 2016, 109, 248–261 CrossRef CAS.
- H. Manafikhi, G. Drummen, M. Palmery and I. Peluso, Total antioxidant capacity in beta-thalassemia: A systematic review and meta-analysis of case control studies, Crit. Rev. Oncol. Hematol., 2017, 110, 35–42 CrossRef.
- M. Liu, W. Yao, Y. Zhu, H. Liu, J. Zhang and L. Jia, Characterization, antioxidant and antiinflammation of mycelia selenium polysaccharides from Hypsizygus marmoreus SK-03, Carbohydr. Polym., 2018, 201, 566–574 CrossRef CAS.
- C. Hou, L. Chen, L. Yang and X. Ji, An insight into anti-inflammatory effects of natural polysaccharides, Int. J. Biol. Macromol., 2020, 153, 248–255 CrossRef CAS.
- C. Tang, R. Ding, J. Sun, J. Liu, J. Kan and C. Jin, The impacts of natural polysaccharides on intestinal microbiota and immune responses–a review, Food Funct., 2019, 10, 2290–2312 RSC.
- X. Sun, M. Duan, Y. Liu, T. Luo, N. Ma, S. Song and C. Ai, The beneficial effects of Gracilaria lemaneiformis polysaccharides on obesity and the gut microbiota in high fat diet-fed mice, J. Funct. Foods, 2018, 46, 48–56 CrossRef CAS.
- J. A. Arcila, S. A. Weier and D. J. Rose, Changes in dietary fiber fractions and gut microbial fermentation properties of wheat bran after extrusion and bread making, Food Res. Int., 2015, 74, 217–223 CrossRef CAS.
- M. Andriamihaja, C. Chaumontet, D. Tome and F. Blachier, Butyrate metabolism in human colon carcinoma cells: Implications concerning its growth-inhibitory effect, J. Cell. Physiol., 2009, 218, 58–65 CrossRef CAS.
- R. Agans, A. Gordon, D. L. Kramer, S. Perez-Burillo, J. A. Rufián-Henares and O. Paliy, Dietary fatty acids sustain the growth of the human gut microbiota, Appl. Environ. Microbiol., 2018, 84, e01525 CrossRef CAS.
- M. G. Rooks and W. S. Garrett, Gut microbiota, metabolites and host immunity, Nat. Rev. Immunol., 2016, 16, 341–352 CrossRef CAS.
- F. Javier, R. Saúl, G. Ignacio, M. M. Elisa, J. V. Claudio and L. Felipe, Colon microbiota fermentation of dietary prebiotics towards short-chain fatty acids and their roles as anti-inflammatory and antitumour agents: A review, J. Funct. Foods, 2016, 25, 511–522 CrossRef.
- J. Wu, H. Shao, J. Zhang, Y. Ying, Y. Cheng, D. Zhao and P. Ling, Mussel polysaccharide α-D-glucan (MP-A) protects against non-alcoholic fatty liver disease via maintaining the homeostasis of gut microbiota and regulating related gut-liver axis signaling pathways, Int. J. Biol. Macromol., 2019, 130, 68–78 CrossRef CAS.
- T. D. Luerce, A. C. Gomes-Santos, C. S. Rocha, T. G. Moreira, D. N. Cruz and L. Lemos, Anti-inflammatory effects of Lactococcus lactis NCDO 2118 during the remission period of chemically induced colitis, Gut Pathog., 2014, 6, 33–40 CrossRef.
- S. Li, Y. Qi, L. Chen, D. Qu, Z. Li, K. Gao, J. Chen and Y. Sun, Effects of Panax ginseng polysaccharides on the gut microbiota in mice with antibiotic-associated diarrhea, Int. J. Biol. Macromol., 2019, 124, 931–937 CrossRef CAS.
- X. Wang, W. Wang, L. Wang, C. Yu, G. Zhang, H. Zhu and Y. Liu, Lentinan modulates intestinal microbiota and enhances barrier integrity in a piglet model challenged with lipopolysaccharide, Food Funct., 2019, 10, 479–489 RSC.
- N. D. Schwalm and E. A. Groisman, Navigating the gut buffet: control of polysaccharide utilization in Bacteroides spp, Trends Microbiol., 2017, 25, 1005–1015 CrossRef CAS.
- X. Ji, C. Hou, Y. Gao, Y. Xue, Y. Yan and X. Guo, Metagenomic analysis of gut microbiota modulatory effects of jujube (Ziziphus jujuba Mill.) polysaccharides in a colorectal cancer mouse model, Food Funct., 2020, 11, 163–173 RSC.
- Z. Lai, C. H. Tseng, H. J. Ho, C. K. Y. Cheung, J. Y. Lin, Y. J. Chen and C. Y. Wu, Fecal microbiota transplantation confers beneficial metabolic effects of diet and exercise on diet-induced obese mice, Sci. Rep., 2018, 8, 15625 CrossRef.
- O. Kanauchi, Y. Matsumoto, M. Matsumura, M. Fukuoka and T. Bamba, The beneficial effects of micromicrobiota, especially obligate anaerobes, and their products on the colonic environment in inflammatory bowel disease, Curr. Pharm. Des., 2005, 11, 1047–1053 CrossRef CAS.
- K. Wang, M. Liao, N. Zhou, L. Bao, K. Ma, Z. Zheng and H. Liu, Parabacteroides distasonis alleviates obesity and metabolic dysfunctions via production of succinate and secondary bile acids, Cell Rep., 2019, 26, 222–235 CrossRef CAS.
- C. Zheng, R. Liu, B. Xue, J. Luo, L. Gao, Y. Wang and X. Peng, Impact and consequences of polyphenols and fructooligosaccharide interplay on gut microbiota in rats, Food Funct., 2017, 8, 1925–1932 RSC.
- Y. Li, J. Li, Q. Su and Y. Liu, Sinapine reduces non-alcoholic fatty liver disease in mice by modulating the composition of the gut microbiota, Food Funct., 2019, 10, 3637–3649 RSC.
- C. G. Scanes and E. Braun, Avian metabolism: its control and evolution, Front. Biol., 2013, 8, 134–159 CrossRef CAS.
- X. Li, Z. Zheng, J. Pan, D. Jiang, Y. Tian and Y. Huang, Influences of melatonin and endotoxin lipopolysaccharide on goose productive performance and gut microbiota, Br. Poult. Sci., 2019, 61, 1466–1799 Search PubMed.
Footnote |
† These authors contributed equally to this work. |
|
This journal is © The Royal Society of Chemistry 2020 |