DOI:
10.1039/D0RA07428D
(Paper)
RSC Adv., 2020,
10, 36303-36316
The reaction of potassium xanthates with five-membered cyclic carbonates: selectivity of the underlying cascade reactions and mechanistic insights†
Received
29th August 2020
, Accepted 18th September 2020
First published on 1st October 2020
Abstract
In this paper we describe the reaction between various potassium xanthates (potassium O-methyl xanthate, potassium O-isobutyl xanthate, xanthate functionalized MPEGs, etc.) and common five-membered cyclic carbonates such as ethylene carbonate (EC) and propylene carbonate (PC). The reaction was carried out under catalyst-free conditions. Intensive evolution of both CO2 and COS and the simultaneous formation of a rich precipitate were found to be the characteristic features of the studied reaction. It was determined that the precipitate consists of various alkoxides, including potassium ethane-1,2-bis(olate) (EC-based reaction) and potassium propane-1,2-bis(olate) (PC-based reaction) and alkoxide-terminated sulfides. It was also demonstrated that the resulting liquid phase (the mother liquor) contains polyalkylene sulfides whose number average molecular weight (Mn) was found to be in the range 400–550 Da and 300–400 Da for EC- and PC-based oligomers, respectively. Further studies revealed that the distribution between major products varies considerably with variation in the parameters of the reaction. Thus, by applying reduced pressure conditions and a temperature equal to 90 °C, up to 95% selectivity towards the formation of alkoxide-terminated sulfide (e.g., potassium 1,1′-thiobis(propan-2-olate)) was achieved. On the other hand, selectivity towards alkoxide formation equal to 98% was achieved for the reactions carried out in the presence of water. As a general trend, it was also established that a shift in balance between products towards the formation of sulfur-containing products (sulfides) occurs with an appropriate increase in the temperature of the reaction. Based on the obtained experimental data supplemented with quantum-chemical calculations (NBO analysis and scanning of the potential energy surface), the mechanisms of the cascade reactions underlying the formation of key intermediates and final products were also proposed.
Introduction
The chemistry of xanthates occupies a special place among sulfur-containing reagents due to the central part played by a number of xanthate reactions in the fields of polymer and organic chemistry. Thus, among radical reactions one cannot but observe the huge impact of xanthates and related substances in polymer chemistry, namely in reversible addition–fragmentation chain-transfer (RAFT) polymerization processes. In this technique xanthates are used as chain-transfer agents (referred to as RAFT agents) to afford control over the generated molecular weight and polydispersity during a free-radical polymerization.1–4 Not limited to their contribution in the field of polymer chemistry, xanthates have found applications as key reagents in some other potent radical reactions, such as Barton–McCombie deoxygenation5 and the Minisci alkylation of heteroaromatic bases.6 The latter methodology has been further expanded by Zard to include not only primary, tertiary and α,α-trifluoromethylaminyl radicals7–9 but also to demonstrate some novel radical cascade reactions of xanthates.10,11 The synthesis of various functional compounds, including γ-thiolactones,12–14 pyridine alkaloid xestamines,15 thiopyranones,16 thiophenes,17 dichloroketones,18 etc., based on free-radical xanthate addition to alkenes has been described in numerous recent works by Zard and Destarac.
Ionic or radical-free reactions of xanthates were found to develop simultaneously.19–25 In this vein, copper-catalyzed approaches towards the preparation of various useful substances using xanthates as an odorless sulfur source were developed by Sekar et al.20–25 Recently, we described a new simple protocol for the catalyst- and solvent-free ring-opening polymerization of lactide using low molecular weight potassium xanthates as initiators or xanthate-functionalized methoxypolyethylene glycols (MPEGX) serving as macroinitiators, thus allowing the preparation of well-defined homopolymers and amphiphilic block copolymers with specific properties for use as pH-responsive drug-delivery systems.26 In order to expand the scope of xanthates as initiators to trigger the catalyst-free polymerization of cyclic monomers, we focused on the use of cyclic carbonates as building blocks. Possessing proven biocompatibility and biodegradability, as well as greater availability compared to lactide, cyclic carbonates such as ethylene carbonate (EC) and propylene carbonate (PC) can be used to obtain a variety of functional polymeric materials, including valuable amphiphilic copolymers for biomedicine. Anyway, unlike the smooth ring-opening of lactide initiated by functional potassium xanthates, the unexpected reaction between cyclic carbonates and xanthates was witnessed. Therefore, the goal of this paper was to determine the products of the observed new reaction between potassium O-alkyl xanthates and two commercially available monomers – PC and EC – focusing on a determination of the relationships between the conditions of the reaction and the final distribution of products. Screening of the mechanisms underlying the observed reactions using both experimental observations and computer modeling was also among the goals of the study. Therefore, the discussion of the results is organized in three sections. In the first section we outline the preliminary data gathered on the peculiarities of the studied process. In the second section we perform an experimental identification of the major products and the third section is devoted to mechanistic insights into the observed reactions.
Results and discussion
Preliminary observations and grounds for further analysis
Based on the previously obtained data on the polymerization of lactide in the presence of functional potassium xanthates,26 we first suggested that the polymerization of cyclic carbonates initiated by xanthates should demonstrate a similar mechanism, leading to the ring-opening of the monomer with subsequent stepwise growth in the molecular weight of the synthesized polymer while maintaining a narrow polydispersity index (Đ ∼ 1, a sign of “living” polymerization, Scheme 1).
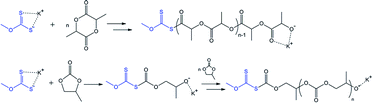 |
| Scheme 1 Top: previously described lactide polymerization initiated by xanthate-functionalized initiators26 and the proposed route for the polymerization of PC in the presence of potassium methylxanthate based on the same idea (bottom). | |
As part of the study into the polymerization of EC and PC, we carried out a series of kinetic experiments using both low molecular weight initiators such as potassium methyl xanthate (PMX), potassium butyl xanthate (PBX), and potassium isobutyl xanthate (PIBX) and macroinitiators based on methoxypolyethylene glycol (MPEG) with various degrees of polymerization (MPEG 500, MPEG 2000, MPEG 5000). In the course of our preliminary experiments, the initiator/monomer ratio and the temperature were varied, and the effect of additives, including urea and complexation agents such as 18-crown-6 ether, on the kinetics of the reaction was studied. Fig. 1 shows the conversion of EC as a function of time for the syntheses carried out at various temperatures (left) and the conversion of PC for the syntheses carried out at 80 °C and initiated with various macroinitiators (middle).
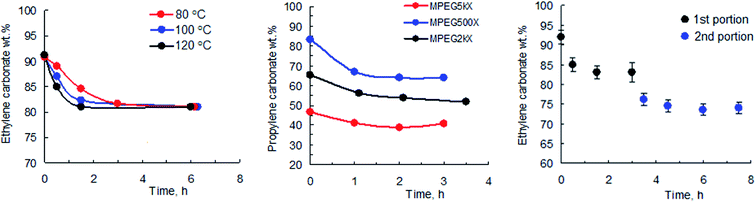 |
| Fig. 1 Left: conversion of EC initiated by PIBX as a function of time for the reactions run at various temperatures. Middle: conversion of PC as a function of time for the reactions carried out at 80 °C and initiated by functional xanthate-terminated macroinitiators. Right: consumption of EC as a function of reaction time for the process with the repeated (two-step) addition of PMX (blue dots = second portion). The synthesis was carried out at 100 °C and the initial EC/PMX ratio was equal to 16/1. | |
A characteristic feature of the first two trends is that the reaction stops upon reaching certain degrees of carbonate conversion. One cannot attribute such dependencies to the equilibration of the reaction, since regardless of the temperature and type of the initiator used (for the reactions with identical initiator to monomer ratios) the reaction terminates while reaching the same degree of monomer conversion. To prove this observation we performed a series of kinetic experiments based on fractional addition of xanthate to the carbonate solution. Indeed, Fig. 1 (right) shows that addition of a second portion of PMX triggers the reaction once again, the subsequent termination of which clearly relates to the consumption of xanthate. Similar results were obtained for the reactions of both PC and EC with various potassium xanthates. The obtained data allowed us to draw a number of conclusions indicating the difference in mechanism of the reaction of cyclic carbonates with xanthates from the previously described polymerisation of lactide in the presence of the same potassium xanthates.26 One can observe that a change in the degree of conversion of carbonates is determined primarily by the initiator to monomer ratio and is practically independent of the temperature change or type of used initiator used.
Thanks to the relatively high polarity of cyclic carbonates, even low molecular weight ionic xanthates, such as PMX, are soluble in both PC and EC. In this regard, one of the characteristic features of the reaction is the cumulative turbidity of the solution with the progress of the reaction, even for the systems involving a large excess of carbonates (100/1). Subsequent scaling of the synthetic procedure (up to 25 gram-scale syntheses with respect to carbonates were applied) with a simultaneous increase in xanthate content in the reaction mixture (from 100/1 to 11/1) allowed one to observe the abundant precipitation of the products (Fig. 2). It was also established that the amount of precipitate isolated correlates with the amount of xanthates used. Scaling of the synthetic protocols also allowed us to establish that the reaction is accompanied by intensive gas evolution. To determine the nature of the gases released in the course of the reaction, a series of additional experiments on trapping the off-gases were carried out using both dimethyl sulfoxide (DMSO) and toluene as solvents (chemisorption media). A glass tube with a height to internal diameter ratio equal to 30 was used for these purposes (see the Experimental section for details). Fig. 2 shows fragments of the GC traces and mass spectra of the corresponding substances trapped in toluene. The analysis performed revealed that the first peak corresponds to carbon dioxide (CO2), and the second peak corresponds to carbonyl sulfide (COS). Therefore, the preliminary observations described here, including kinetic trends and the defined turbidity of the reaction system with subsequent rich precipitation of the final products accompanied by intensive gas evolution, indicate that the reaction between the five-membered cyclic carbonates studied and potassium xanthates proceeds through an unusual and to the best of our knowledge undescribed mechanism different from the one proposed in Scheme 1.
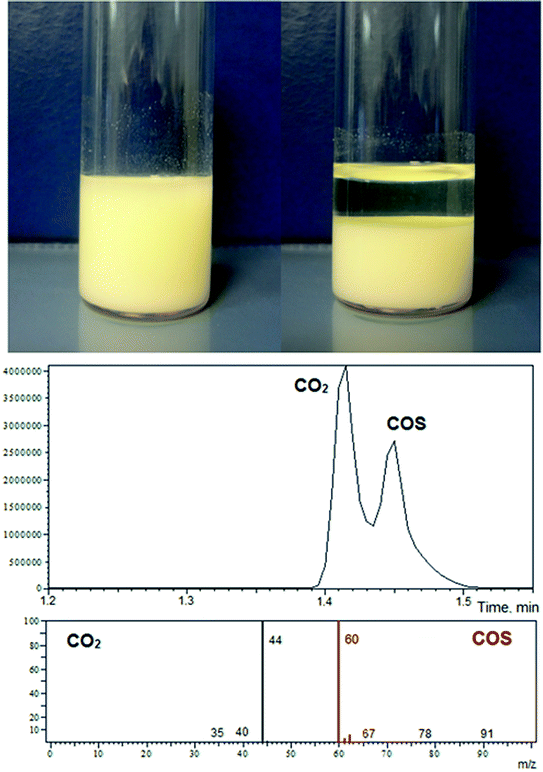 |
| Fig. 2 Top: images of the slurry containing solid products and residual PC captured immediately after the completion of the reaction between PC and PIBX (PC/PIBX = 25/1) carried out at 100 °C (left) and after a few hours of relaxation. Bottom: GC traces for the gases evolved in the course of the reaction between PC and PIBX and mass-spectra of the identified substances. | |
Determination of the reaction products
Analysis of the content of precipitate. The precipitated products were washed with acetone (see the Experimental part) and a preliminary analysis of the solubility in basic organic solvents was undertaken. At this stage, it was determined that the powdered product is soluble in water and partially soluble in hot DMSO. On the other hand, the precipitate was found to be insoluble in most low-polar and non-polar organic solvents. However, additional experiments showed that the precipitate can be homogenized even in chloroform when specific potassium ion complexation agents, such as 18-crown-6 ether, were added. This finding indicates that substances constituting the precipitate possess an ionic structure bearing potassium counterion atoms. In line with this observation, further GC analysis of the prepared solutions of precipitate in either DMSO or chloroform (in the presence of 18-crown-6 ether) demonstrated that the resulting chromatograms do not contain any intensive signals corresponding to the volatile products. On the other hand, GC analysis of the powdered product dissolved in water showed that, in contrast to organic solutions based on DMSO and chloroform + 18-crown-6 ether mixture, the aqueous solution contains three well-defined signals. Assuming the retention times established for these signals, one can predict the high-boiling nature of the corresponding substances. This indicates that some sort of exchange reaction is observed in excess water, which is more likely to be the protonation of the starting ionic compounds, making them volatile and therefore suitable for GC analysis. Thus the obtained volatile products were extracted from the aqueous phase with chloroform and separated via preparative chromatography using a dry column vacuum chromatography method to give individual compounds (see the Experimental section) prior to the spectral analysis. Fig. 3 shows fragments of the 1H NMR spectra of the precipitate dissolved in deuteroxide and one of the major products extracted from aqueous solution (protonated form), isolated and dissolved in CDCl3. In conjunction with GC-MS analysis of the product mixture, the chemical shifts of the substance centered at 3.79 ppm (CH-group), 3.46 and 3.35 ppm (protons of the CH2-group) and the doublet at around 1.05 ppm (CH3-group) unequivocally confirmed that the substance corresponds to 1,3-propylene glycol, which precipitates in the form of the corresponding alkoxide (potassium propane-1,2-bis(olate)) in the course of the reaction between PC and xanthates. The spectrum of the second substance (Fig. 3) shows a similar pattern in heavy water, although one can observe that the chemical shifts previously assigned (for 1,3-propylene glycol) to methylene protons were found to show an upfield shift, thus locating in the region 2.52–2.65 ppm. Additional correlation HSQC NMR analysis confirmed that these protons indeed belong to the single carbon atom (Fig. 3, right). The observed shielding of the methylene group indicates the adjacency of the group to a heteroatom, which is the sulfur atom in this particular case as it is known that chemical shifts of methylene protons of sulfides and related compounds are typically observed in the region 2.4–2.6 ppm. The spectrum of the isolated product dissolved in CDCl3 indicates that the system contains signals of labile acidic protons attributed to OH-groups (a broad signal at 3.7 ppm, see Fig. 3). The IR spectrum confirms this finding as one can observe a characteristic broad band in the region of stretching vibrations of OH-groups (3371 cm−1). IR analysis also demonstrated the absence of the characteristic band of the thiol group basically located in the region 2500–2600 cm−1. This observation excludes variants of the structures comprising SH-groups. Summarizing the obtained spectral data, it was established that the obtained substance is a sulfur-containing symmetric diol, namely 1,1′-thiobis(propan-2-ol), and therefore the structure of the powdered product obtained in the course of the reaction between PC and xanthates was determined to be the corresponding alkoxide i.e., potassium 1,1′-thiobis(propan-2-olate). Subsequent GC-MS analysis confirmed the structure of the titled sulfur-containing diol compound since the spectrum contained the molecular ion peak [M+] equal to 152. It is worth mentioning that the intensity of the molecular ion was found to be low, which complicated the initial prediction of the structure of the isolated sulfide 1,1′-thiobis(propan-2-ol).
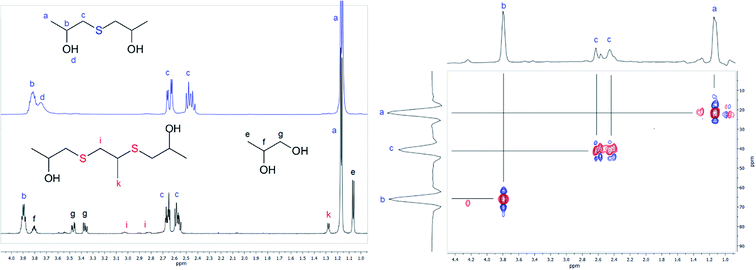 |
| Fig. 3 Left: 1H NMR spectra of 1,1′-thiobis(propan-2-ol) (blue) dissolved in CDCl3 and powdered precipitate depicted in Fig. 2 dissolved in D2O. Right: HSQC correlation spectra for the sulfide 1,1′-thiobis(propan-2-ol) dissolved in CDCl3. Spectra were recorded at 25 °C. | |
The third substance constituting the obtained precipitate demonstrates a downfield shift for both methyl and methylene protons with respect to the analogous signals observed for 1,1′-thiobis(propan-2-ol). Thus, the proton chemical shift of the CH3-group was determined to be located at around 1.28 ppm and protons of the CH2-group were observed in the region 2.8–3.0 ppm (markers k and i in Fig. 3). Considering the results of the GC-MS analysis, the discussed compound was attributed to 3,6-dithia-1,5,8-trimethyl-1,8-octanediol (disulfide). Analogous results were observed for the synthesis based on the reaction between EC and xanthates, giving 2,2′-thiodiethanol and symmetrical 3,6-dithia-1,8-octanediol as sulfide and disulfide respectively.
Analysis of the mother liquor. Along with decoding the composition of the precipitate formed in the course of the reaction between cyclic carbonates and xanthates, the composition of the liquid phase (the mother liquor) collected after the completion of the reaction was also studied. Thanks to the initial proposition of the possibility of the polymerization of cyclic carbonates in the presence of xanthates, we performed a large series of molecular weight distribution analyses for the resulting solutions via gel permeation chromatography (GPC) to verify this assumption. Fig. 4 shows GPC traces typical for oligomeric products extracted from the mother liquor solutions after the completion of the reactions between either PC or EC with low molecular weight xanthates. As can be seen, a set of GPC columns designed to study polymers with low molecular weight characteristics (up to 2k Da) allowed us to obtain GPC traces with relatively high resolution and therefore to analyze the molecular weight distribution of individual oligomeric chains. It can be seen that each of the individual peaks differs from the neighboring peaks by a constant value, indicating the homogeneity of the structure of the polymer chains. Our initial proposition on the possible formation of polycarbonates via the xanthate-initiated ring-opening polymerization of cyclic carbonates (Scheme 1) was rejected as no characteristic chemical shifts for CH2- and CH-groups (3.45–3.55 and 4.9 ppm respectively27) were observed in the recorded 1H NMR spectra of the extracted oligomers. On the other hand, chemical shifts located in the region 2.4–2.9 ppm and attributed previously to methylene groups adjacent to sulfur atoms (sulfide and disulfide molecules, Fig. 3) were found to be the dominant chemical shifts in 1H NMR spectra of the synthesized oligomers. Fig. 4 (right) shows a fragment of the 1H NMR spectrum of one of the obtained oligomers in the region of the CH3-groups, indicating the presence of two chemical shifts, namely a multiplet at 1.28–1.31 ppm corresponding to methyl protons of the propylene fragments allocated between two sulfur atoms (k, Fig. 3 and 4) and a doublet at 1.17 ppm corresponding to the methyl protons of the terminal propylene units (a, Fig. 3 and 4). It was observed that in contrast to the isolated disulfide molecule, the multiplet at 1.28–1.31 ppm depicted in Fig. 4 possesses higher integral intensity in comparison with the integral intensity of the doublet at 1.17 ppm (k/a = 0.5 for the disulfide molecule and a 1.79 ratio was established for the discussed oligomer). These data were used to calculate the number average molecular weight (Mn) of the obtained oligomers. It was determined that Mn for polypropylene sulfide-based oligomers was typically in the range 400–550 Da and Mn for polyethylene sulfide-based oligomers was in the range 300–400 Da.
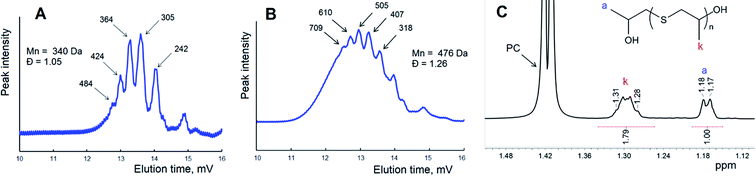 |
| Fig. 4 GPC traces of oligomers synthesized in the course of the reaction between EC (A) and PC (B) with PMX carried out in bulk at 100 °C and a fragment of the 1H NMR spectrum (right) of polypropylene sulfide-based oligomers recorded in CDCl3 at 25 °C. | |
Influence of the reaction conditions on distribution of the obtained products. Kinetic experiments accompanying the simultaneous separation and analysis of the formed precipitates allowed one to notice that the distribution of the three major products (potassium alkoxides and alkoxide-terminated sulfides and disulfides) varies with a change in the temperature of the synthesis. Therefore, further experiments focused on an investigation of the influence of the reaction conditions on the balance of the alkoxides in the precipitated product mixture. The study of the product distribution was based on an analysis of the 1H NMR spectra of the separated and purified powdered product in the region of the methyl protons (see the Experimental section for details). This type of chemical shift was found to be well resolved and no overlapping of the relative signals was observed. Fig. 5 shows fragments of the spectra recorded for several representative powdered products extracted and analyzed immediately after the completion of the reaction.
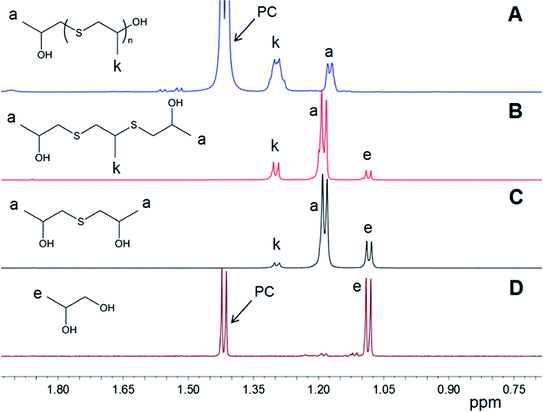 |
| Fig. 5 Fragments of 1H NMR spectra in the region of methyl protons for polypropylene sulfide in CDCl3 (A); precipitates obtained in the course of the reaction between PC and PMX in bulk at 100 °C (B) and 80 °C (C) and the precipitate formed in the course of the reaction between PC and PMX at 90 °C in the presence of water (10%). Spectra (B–D) were recorded in D2O at 25 °C. | |
Summarizing the data gathered from over a hundred experiments, it can be stated that as a general trend the fraction of sulfur-containing products increased with an increase in the temperature of the synthesis. In particular, for the reaction of PMX with PC carried out in bulk at 50 °C, the ratio between diol, sulfide and disulfide was equal to 1/1/0.025. Raising the temperature of the synthesis to 80 °C and eventually to 100 °C changes the ratio of products in the precipitate to 1/1.7/0.28 and 1/4/2.5, respectively. A further increase in the temperature up to 130 °C leads to something of a decrease in the fraction of sulfur-containing products in the powdered mixture (the ratio is equal to 1/2/1.4). However, one can observe that in contrast with the synthesis which proceeded at 100 °C, the proportion between sulfide and disulfide changed towards an increase in the fraction of disulfide. The content of the disulfide molecules in the system was found to correlate with the formation of polypropylene sulfide-based molecules, which indicates that similar mechanisms underlie both disulfide formation and polymerization reactions. Taking into account that the reaction between cyclic carbonates and xanthates is accompanied by intensive evolution of CO2 and COS gases, we decided to conduct a set of experiments for the same reaction system (PC + PMX in bulk, 90 °C) but under reduced pressure conditions. Maintaining the residual pressure in the range 2–4 mm Hg allowed us to achieve a huge shift in the balance between components of the precipitate towards sulfide formation (propanediol/sulfide/disulfide = 1/33.5/0.77). The obtained data demonstrate the remarkable (up to 95%) selectivity of the process towards the preparation of potassium 1,1′-thiobis(propan-2-olate) via manipulation of the reaction temperature and application of the reduced pressure conditions. Next, we turn to a study of the effect of solvents or additives on the selectivity of the reaction. It was shown that addition of aprotic polar solvents, such as DMSO (carbonate/DMSO = 1/1 v/v), does not lead to a substantial change in the distribution of the three major products (propanediol/sulfide/disulfide = 1/3.5/2.2). On the other hand, very different results were obtained for the systems with the addition of water. Fig. 5D shows the 1H NMR spectrum of the precipitate formed in the course of the reaction between PC and PMX carried out at 90 °C in the presence of water (PC/water = 9/1 v/v). It can be seen that addition of water leads to the selective preparation of potassium propane-1,2-bis(olate) (detected as 1,3-propanediol in D2O solution). The ratio between propanediol and sulfide determined by 1H NMR was equal to 1/0.025 and no disulfide was found to be formed in the course of the reaction. The obtained ratio and evidence of absence of disulfide in the resulting mixture correspond to the achieved selectivity of the reaction towards the formation of potassium propane-1,2-bis(olate) equal to 98%. The discussed results are also summarized in Table 1.
Table 1 Conditions and results of the reactions between PC and PMX
Initial system |
Temperature, °C |
Pressure, mm Hg |
Product distributionc |
PMX conversion, % |
Isolated yield of the precipitate, gd |
DMSO/PC = 1/1 v/v. PC/water = 9/1 v/v. Distribution between alkoxides presented in Fig. 5(B–D) in a protic form: propanediol/sulfide/disulfide. Yield of the isolated and purified precipitate per 1 gram of added PMX. |
PC/PMX |
50 |
760 |
1/1/0.025 |
100 |
0.41 |
PC/PMX |
80 |
760 |
1/1.7/0.28 |
100 |
0.62 |
PC/PMX |
100 |
760 |
1/4/2.5 |
100 |
0.71 |
PC/PMX |
130 |
760 |
1/2/1.4 |
100 |
0.64 |
PC/PMX |
90 |
2–4 |
1/33.5/0.77 |
100 |
0.7 |
PC/PMX/DMSOa |
100 |
760 |
1/3.5/2.2 |
100 |
0.49 |
PC/PMX/waterb |
90 |
760 |
1/0.025/— |
100 |
0.32 |
Mechanisms underlying the observed reactions
One can find that an important peculiarity of the discussed processes is the absence of a requirement to use any additional activators of the reaction, such as traditional metal-based catalysts or even active organic molecules (organocatalysts). Thus, in a typical example, the system consists of only two components – pure xanthate (e.g., PMX, PIBX, or PBX) and one of the commercial five-membered cyclic carbonates also serving as a medium to carry out the synthesis (bulk reaction conditions). As was discussed in a previous section, no polycarbonate-based products, the formation of which was assumed in Scheme 1, were detected in the reaction systems after the completion of the process. Taking into account the observed quantitative xanthate conversion, one can suggest an alternative to ROP reactions triggered by active nucleophilic products including the product obtained after the first step of nucleophilic addition of xanthates to cyclic carbonates. As can be seen from Scheme 1, the process is ionic in nature, which means that the growing chains are represented by alkoxide-terminated molecules capable of initiating the subsequent transformations in the studied systems. According to the discussed data on characterization of the product mixture, these transformations lead to the production of various low molecular weight alkoxides instead of the proposed polycarbonate macromolecules. The high electrophilicity of the carbon atoms (marked with * in Scheme 2) of both xanthate and thiocarbonate groups is the characteristic feature of linear thiocarbonate intermediate 1 formed after the initial attack of xanthate on the carbonyl carbon atom of the cyclic carbonate accompanied by subsequent ring-opening of the monomer. Analysis of the natural bond orbitals (NBO) of the key C–O and C–S bonds of the linear thiocarbonate 1 performed in the framework of this study showed that the resulting linear products are characterized by lower energies of antibonding (acceptor) orbitals of the key bonds in comparison with the corresponding orbitals of the initial cyclic carbonates. This trend provokes the preferential attack of terminal alkoxide groups on C
O and C
S carbon atoms of the growing chain. This phenomenon is recognized as a back-biting reaction,28,29 which in our case most likely proceeds through the reaction between two separated intermediates, as depicted in Scheme 2(A and B).
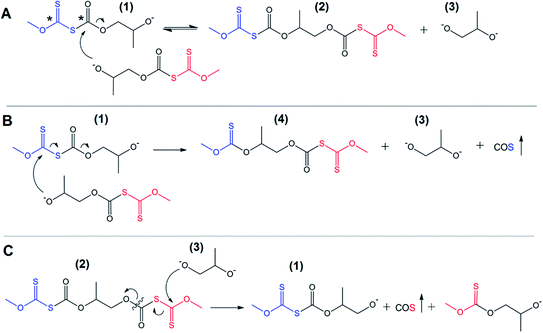 |
| Scheme 2 (A and B) Interactions of two thiocarbonates (growing chains, 1) via chain termination reactions with the subsequent formation of neutral species (2 and 4) and alkoxide 3. (C) Attack of alkoxide propane-1,2-bis(olate) on the C S carbon of the xanthate group of 2 accompanied by COS release and formation of initial thiocarbonate 1 and intermediate 1-((methoxycarbonothioyl)thio)propan-2-olate. Potassium ions are omitted for clarity. | |
This scheme shows the chain termination reaction caused by the interaction between two active thiocarbonates 1 (see also Scheme 1). In the first two cases (Scheme 2A and B) the reaction proceeds through the formation of dixanthates (2 and 4) and potassium alkoxide 3. The latter was determined to be one of the major products, at least for the reaction proceeding in the presence of water. In spite of the fact that alkoxide 3 shows poor solubility in carbonate solution, small portions of this substance should remain in a liquid phase, therefore leading to the transformation (analogues of the back-biting reactions) depicted in Scheme 1C. This formal process also gives rise to the formation of 1-((methoxycarbonothioyl)thio)propan-2-olate. Fig. 6 shows GPC traces for the products obtained in the course of the reaction between macroinitiator MPEG2KX and PC.
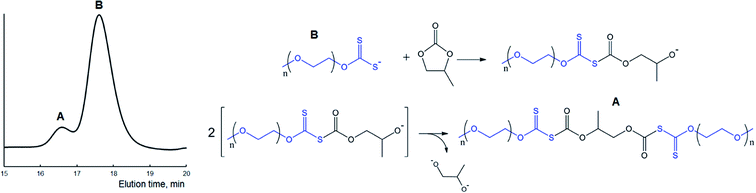 |
| Fig. 6 GPC trace of polymer (A) obtained in the course of the reaction between MPEG2KX (B) and PC at 100 °C in bulk and a scheme displaying the proposed reactions (see also Scheme 2A). Potassium ions are omitted for clarity. | |
It was demonstrated in our previous paper that chromatograms of amphiphilic block-copolymers MPEG-b-PLA synthesized in the course of the reaction between lactide and xanthate-functionalized MPEGs (e.g., MPEG2KX) are characterized exclusively with a unimodal molecular mass distribution. In contrast to these data, one can clearly observe the bimodal character of the molecular mass distribution for polymers extracted after the completion of the reaction between cyclic carbonates and macroinitiators. It was determined that regardless of the macroinitiators (MPEG5KX, MPEG2KX or MPEG500X) used, the Mn value of the first mode (e.g., 5700 Da, spike A, Fig. 6) was found to be precisely twice as large as the Mn value of the second mode (2570 Da, basic signal of macroinitiator, spike B, Fig. 6). It was also established that the addition of pure MPEG prior to the reaction does not lead to any increase in the area of the first mode (A, Fig. 6). These experimental data clearly verify the reorganizations (chain termination reactions) depicted in Scheme 2 and Fig. 6, leading to the formation of various charged and uncharged products.
It is common knowledge that an attack of nucleophiles on methylene carbon is typical for the reactions of five-membered cyclic carbonates.30–34 This type of transformation is characterized by CO2 evolution (decarboxylation reaction) accompanied by the subsequent formation of intermediates such as 1-((methoxycarbonothioyl)thio)propan-2-olate I, as shown in Scheme 3.
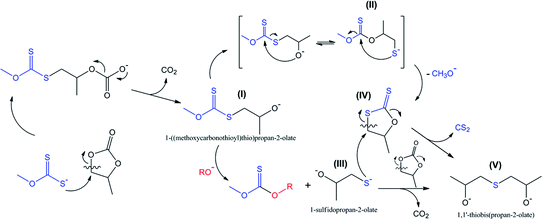 |
| Scheme 3 Cascade reactions between PMX and PC leading to the formation of sulfide V. | |
This type of reaction underlies the cascade process leading to the formation of sulfide V (1,1′-thiobis(propan-2-olate)), which was determined to be one of the major products of the reaction between PC and potassium xanthates. The results of scanning the reaction coordinate along the newly formed S–C bond (attack of xanthate on the methylene carbon atom of PC) via m06-2x/6-311++G(d,p)/PCM quantum-chemical calculations demonstrate the spontaneous rupture of the C–O bond of PC (Scheme 3) when the distance between the S atom of xanthate and the C atom of PC reaches a certain value (see Fig. 7). Further reaction of I with any of the alkoxides present in the system (the formation of such molecules was discussed above, see Scheme 2) leads to the formation of intermediate 1-sulfidopropan-2-olate (III). The high nucleophilicity of 1-sulfidopropan-2-olate was proved by NBO analysis, as it was calculated that the energy of the localized lone pair orbital of the sulfur atom of III E(LPS-) was equal to −0.692 au. The value of the lone pair orbital determined for the oxygen atom of alkoxide 3 (see Scheme 2) E(LPO-) was equal −0.764 au, indicating the lower energy gap between the occupied donor NBO of nucleophile III and the unoccupied acceptor NBO of electrophilic reagents, which in turn facilitates the kinetics of the appropriate reaction. This circumstance leads to the preferential attack of the thiolate sulfur atom on the methylene group of either residual PC or dithiocarbonate molecule IV, which can be formed in situ via the alternative route depicted in Scheme 3. Dithiocarbonate IV was independently synthesized by our group (see the Experimental section for details) and used as a standard in the GC-MS experiments to verify the formation of IV in the course of the studied reaction. It was demonstrated that IV was indeed formed in the course of the reaction, as both IV and simultaneously formed CS2 were detected in the reaction systems. The final attack of III on either PC or IV proceeds through the same scenario as for the attack of the initial xanthate on cyclic carbonate, accompanied by the release of CO2 or CS2 depending on the type of carbonate involved in the last step of the discussed cascade reaction.
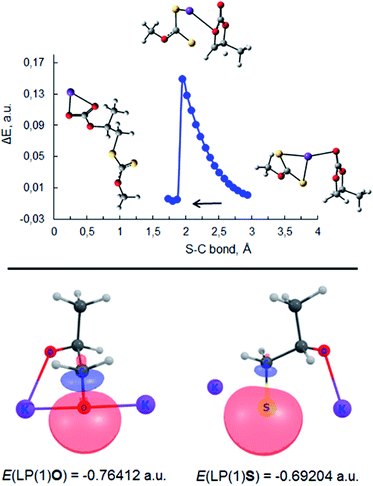 |
| Fig. 7 Top: scan of total energy along the reaction coordinate for the attack of S− of PMX on the methylene carbon atom of PC (S–C bond formation) calculated at the m06-2x/6-311++G(d,p)/PCM level of theory (sulfur – yellow, potassium – purple, oxygen – red). Bottom: images and energies of donor NBO of C1 oxygen of potassium propane-1,2-bis(olate) (3, Scheme 2) and sulfur atom of potassium 1-sulfidopropan-2-olate (III, Scheme 3) calculated at the same level of theory. | |
The proposed scheme does not explain the route for the preparation of the third product (disulfide, see Fig. 5B), the formation of which was found to be promoted at elevated temperatures. GC-MS analysis of the reaction mixtures for the synthesis carried out at temperatures above 80 °C explicitly shows the presence of propylene sulfide alongside the previously discussed CS2 and dithiocarbonate IV in the mother liquors. In accordance with these findings, we turned to a study of possible routes towards the in situ formation of propylene sulfide. Fig. 8 shows one of the proposed reactions leading to the formation of propylene sulfide in situ. The proposed process is based on the preliminary formation of the key intermediate I (see also Scheme 3) in the course of the reaction between initial potassium xanthate and any of the studied cyclic carbonates. However, in contrast with the proposed process in Scheme 3 leading to the further formation of dithiocarbonate IV, the sulfur atom of I attacks the methyne carbon rather than the thiocarbonyl carbon (C
S). This type of intramolecular cyclization reaction accompanied by the subsequent formation of thiirane and ionic potassium O-methyl carbonothioate was studied via quantum-chemical calculations. This time we also used the discussed previous scanning of the potential energy surface along the newly formed S–C bond at the m06-2x/6-311++G(d,p)/PCM level of theory. It is remarkable that, as in the case of the previously described attack of xanthate on the methylene carbon atom of PC, the reaction is accompanied by the spontaneous rupture of the C–O bond followed by the release of two new molecules (Fig. 8). The details of the theoretical method used (total relaxation of all atoms of II except for the sulfur and carbon atoms of the newly formed S–C bond) and consequently obtained energetic profile indicate the smooth character of this type of transformation. The next stage is characterized by the attack of thiolate III (the in situ formation of which is analogous to that described in Scheme 3) on the methylene carbon of propylene sulfide. This reaction is accompanied by the ring-opening of the thiirane molecule, yielding intermediate VI. The subsequent reaction of VI with the residual PC in a decarboxylation fashion leads to the final formation of disulfide VII.
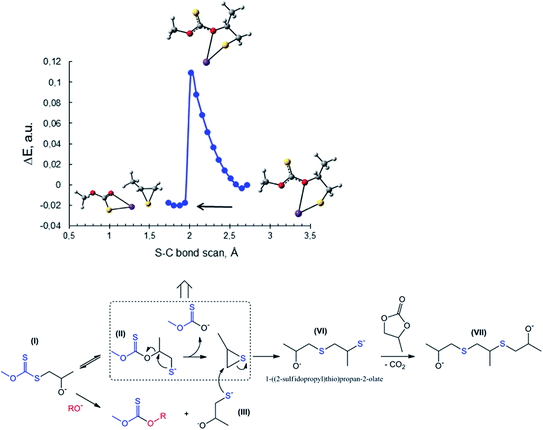 |
| Fig. 8 Top: scan of total energy along the reaction coordinate for the intramolecular attack of S− on the methyne carbon atom of the key intermediate II (S–C bond formation via intramolecular cyclization) calculated at the m06-2x/6-311++G(d,p)/PCM level of theory (sulfur – yellow, potassium – purple, oxygen – red). Bottom: schematic representation of the cascade reactions leading to the preparation of disulfide VII. | |
The variation in the last step of the discussed cascade reaction is shown in Scheme 4. The proposed scheme underlies the experimentally confirmed (NMR and GPC, Fig. 4) formation of polypropylene sulfide-based molecules. In this case, thiolate-functionalized analogues of VI repeatedly attack propylene sulfide molecules, thus providing the progressive growth of the oligomer chain until the terminal thiolate group reacts with a residual carbonate molecule.
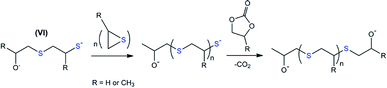 |
| Scheme 4 The proposed route for the chain growth of polyalkylene sulfides based on intermediate VI. R = H for EC-based and CH3 for PC-based oligomers. | |
In light of the proposed cascade reactions, one can now explain the experimentally achieved selectivity towards the preparation of sulfide V under the conditions of reduced pressure (see Table 1). As was suggested, the formation of dithiocarbonate IV depicted in Scheme 3 is a reversible process. The same applies to the formation of propylene sulfide. Thus, under normal conditions, these two processes are competitive, since intermediate II is the key starting structure for both reactions. When the reduced pressure is applied, the CS2 (side-product) released in the course of dithiocarbonate formation is constantly removed from the reaction system, shifting the equilibrium towards dithiocarbonate formation. The latter possesses a relatively high boiling point (114–115 °C at 2 mm Hg) which facilitates the progress of the reaction towards final sulfide V formation. In contrast to IV and similar to CS2, propylene sulfide possesses a relatively low boiling point (72–75 °C at 760 mm Hg) causing the continuous removal of propylene sulfide from the reaction system (under reduced pressure conditions), minimizing the possibility of attack by thiolates III and VI (Schemes 3 and 4, respectively) on propylene sulfide. These circumstances explain the unusual distribution between the three major products in the precipitate obtained in the course of the reactions carried out at 2–4 mm Hg (Table 1).
Experimental
Materials
Commercial 18-crown-6 (1,4,7,10,13,16-hexaoxacyclooctadecane) ≥ 99%, anhydrous carbon disulfide (CS2) ≥ 99.5%, propylene carbonate (PC) ≥ 99%, ethylene carbonate (EC) 99%, propylene oxide ≥ 99%, methanol (HPLC grade) ≥ 99.9%, potassium hydroxide (reagent grade) 90%, ethyl acetate (HPLC grade) ≥ 99.7%, acetone (HPLC grade) ≥ 99.8%, toluene (HPLC grade) 99.9%, and hexane (HPLC grade) ≥ 95% were used without further purification. Sodium methoxide solution (30 wt% in methanol) was purchased from a local supplier and used as received. Tetrahydrofuran (THF) was distilled over potassium hydroxide prior to use. Methoxypolyethylene glycol (MPEG) with average molecular weight (Mw) equal to 5000 and 2000 g mol−1 was purchased from “Clariant” and MPEG with Mw equal to 500 g mol−1 was obtained from a local supplier “Norchem” and used as received. Commercial potassium O-isobutyl xanthate (PiBX) with average purity ≥ 98% was used as received. Commercial potassium O-ethyl xanthate (PEX) was recrystallized from ethanol and dried under reduced pressure prior to the experiments. Potassium O-methyl xanthate (PMX) and xanthate-functionalized MPEG macroinitiator (MPEGX) were synthesized and purified in accordance with the procedure described previously.26
Devices and general analysis
NMR spectra were recorded using a Bruker Avance spectrometer operated at 600 MHz for 1H. Chemical shifts (δ) are given in parts per million (ppm) relative to residual solvent signals (DMSO-d6: 1H – 2.5 ppm; CDCl3: 1H – 7.25 ppm, 13C – 77.16).35 Spectra were recorded at 25 °C. The distribution of the major products in the collected precipitate was determined based on the integral intensity values of the characteristic chemical shifts of the methyl protons of these substances. The ratio of disulfide VII to potassium propane-1,2-bis(olate) was calculated as Sd/Sa, where Sd corresponds to the integral intensity of the protons of the CH3-group located between the two sulfur atoms of disulfide VII (1.18–1.31 ppm) and Sa corresponds to the integral intensity of the methyl group of potassium propane-1,2-bis(olate) located in the region 1.05–1.1 ppm.
Taking into account the fact that each of the methyl groups of disulfide VII located in the region 1.18–1.31 ppm correspond to two methyl groups of the terminal propylene units (chemical shifts are observed around 1.17 ppm) and also the fact that the chemical shifts of the protons of the terminal methyl groups for both sulfide and disulfide are identical, the integral intensity of the terminal CH3 protons of sulfide V can be calculated as ΣSsd − 2Sd. Here ΣSsd denotes the combined integral intensity of the terminal CH3 protons for both sulfide V and disulfide VII. Given that the two terminal groups of sulfide V correspond to the one central CH3-group of disulfide VII, the ratio of disulfide VII to sulfide V was calculated according to the relationship 2Sd/(ΣSsd − 2Sd).
The same principle was applied to calculate the number of propylene sulfide units constituting corresponding polypropylene sulfides (degree of polymerization). Therefore, the number of units was calculated as 2Sc/St, where Sc corresponds to the integral intensity of the CH3 protons of the chain (propylene sulfide) units located in the region 1.18–1.31 ppm and St corresponds to the integral intensity of the terminal CH3 protons (1.15–1.2 ppm).
FT-IR spectra were recorded at a resolution of 0.5 cm−1 on a Shimadzu IRAffinity-1 spectrometer at 25 °C and 20 scans were accumulated. The spectra of the crystalline products were recorded as a KBr pellet. Liquid materials were analyzed as a drop squeezed between two ZnSe crystals.
UV-visible spectra of the studied compounds dissolved in either distilled water or THF were recorded with a Shimadzu 1800 spectrophotometer. The absorption spectra were recorded at room temperature in the range 190–500 nm using cuvettes of 1 cm path length.
The purity of the starting chemicals and carbonate consumption in the course of the polymerization reaction were determined by gas chromatography using a Chromos GC-1000 chromatograph equipped either with a VertiBond AqWAX capillary column (length 60 m, I.D. 0.32 mm, film 0.50 μm) or a ValcoBond VB-1701 capillary column (length 60 m, I.D. 0.32 mm, film 0.50 μm) and a flame ionization detector. The temperatures of the flame ionization detector and sample injector were equal to 250 °C. For measurements of monomer consumption in the course of the reaction, the initial temperature was maintained at 100 °C for 5 min, after which the temperature was elevated to 200 °C at a speed of 10 °C min−1. The final temperature was maintained for an additional 23.3 minutes.
Mass spectra were recorded on a GC/MS-QP2010 (Shimadzu) equipped with ZB-FFAP (Phenomenex) and VB-1701 (VICI AG Int.) capillary columns. The temperatures of the flame ionization detector, sample injector and temperature control mode for the columns were identical to those described above for the traditional GC analysis.
Gel permeation chromatography (GPC) was used to determine the molecular weights and Mw/Mn ratio for the synthesized polymers on a Chromos LC-301 equipped with an isocratic HPLC pump Alpha-10 and refractive index detector (Waters). THF was used as a mobile phase. The flow rate was equal to 1 ml min−1. Columns and detector were thermostated at 30 °C. Phenogel 5 μm 500 Å (300 × 7.8 mm) and Phenogel 5 μm 10 × 105 Å (300 × 7.8 mm) were used as GPC columns to measure the molecular weights of the polymers. Linear polystyrene standards with molecular weights equal to 162, 580, 1470, 3250, 8500, 50
000 and 110
000 Da were used for the calibration.
Precise concentration of the potassium hydroxide was specified via standard isothermal potentiometric titration.
Quantum chemical calculations
Quantum mechanics calculations were performed using the Gaussian 03 program.36 The Minnesota M062X/6-31+G(d,p) functional was used for local minima calculations. Molecular systems were optimized by a condensed-phase simulation using a conductor-like polarizable continuum model37–40 with tetrahydrofuran as solvent, as implemented in Gaussian. A relaxed potential energy scan was employed at the same level of theory. Vibrational frequency calculations were conducted to confirm the local minima (all vibrational frequencies are positive). The scope and possibilities of the natural bond orbital (NBO) analysis applied in this study have been described by Weinhold et al.41–46 The mathematical and historical background of NBO methods can be found elsewhere.45 Examples of the use of this method to test the donor–acceptor ability of individual molecules and molecular complexes can be found in some of our papers.47–49
Synthetic protocols
Typical procedure for the preparation of potassium 1,1′-thiobis(propan-2-olate) and potassium propane-1,2-bis(olate) via the reaction of potassium xanthate with PC. A jacketed glass reactor (25 ml) containing a stirring bar was charged with 10 g of PC. The solution was degassed and filled with nitrogen three times, after which the preliminary preheated oil was allowed to fill the jacket. When the desired temperature of 100 °C was reached and the system had been thermostated for an additional 10 minutes PMX (1 g) was added in small portions. The addition of xanthate was accompanied by gas evolution, so each new portion was introduced after gas evolution had ceased. When the last portion had been added and the evolution of gas had stopped, the synthesis was carried out for another 30 minutes. When the resulting heterogeneous mixture had cooled to 25–30 °C, acetone (5–10 ml) was added to decrease the viscosity of the mixture and therefore to facilitate decantation of the precipitate. When the powdered solid had been separated via filtration on a synthetic (nylon) filter, the crude product was washed with small portions of either acetone or THF and dried under reduced pressure at 50 °C, giving 0.71 g of a mixture of propanolates: potassium 1,1′-thiobis(propan-2-olate) 1H NMR (600 MHz, D2O) δ 3.88 (dd, J = 12.0, 6.1 Hz, 1H), 2.65 (dt, J = 13.5, 4.5 Hz, 1H), 2.59–2.52 (m, 1H), 1.16 (d, J = 6.2 Hz, 3H) ppm; potassium propane-1,2-bis(olate) 1H NMR (600 MHz, D2O) 3.79 (dd, J = 10.6, 6.5 Hz, 1H), 3.46 (dd, J = 11.6, 3.9 Hz, 1H), 3.35 (dd, J = 11.6, 6.8 Hz, 1H), 1.05 (d, J = 6.4 Hz, 3H) ppm. The procedure for the preparation of potassium 2,2′-thiodiethanolate and potassium ethane-1,2-bis(olate) based on the reaction of EC with xanthates was identical except for the degassing procedure which was performed after the system was preheated to 40 °C and the final addition of acetone which was performed after the system had cooled to 40–50 °C.
Typical procedure for the preparation and isolation of 1,1′-thiobis(propan-2-ol) from potassium 1,1′-thiobis(propan-2-olate). A powdered mixture of propanolates was dissolved in distilled water (1/10 w/w). When the solute had completely dissolved in water, an equivalent volume of chloroform was added to the system. After the extraction, the organic (bottom) layer was collected. The residual organic substances were extracted via the repeated addition of small portions of chloroform. The combined organic phase was dried with calcium sulfate, filtered and evaporated on a rotary evaporator under reduced pressure. The crude mixture of 1,1′-thiobis(propan-2-ol) and 1,2-propanediol was separated via DCV chromatography.50 Gradient elution 300 ml (EtOAc/Hex = 1/4 v/v) – 100 ml (EtOAc/Hex = 1/3 v/v) – 100 ml EtOAc was applied, giving 1,1′-thiobis(propan-2-ol) as a colorless oil. 1H NMR (600 MHz, CDCl3) δ 3.86–3.75 (m, 1H), 2.63 (dd, J = 13.8, 3.5 Hz, 1H), 2.50–2.41 (m, 1H), 1.15 (d, J = 6.3 Hz, 3H) ppm; 13C NMR (151 MHz, CDCl3) (S,S-isomer): δ 22.2 (CH3), 41.71 (CH2), 66.25 (CH); (S,R-isomer): δ 22.3 (CH3), 42.19 (CH2), 66.9 (CH) ppm. FTIR (squeezed drop, ZnSe): 3371 (br, OH), 2969, 2925, 2876, 1127, 1073, 939 cm−1. MS (EI) m/z: 150 [M]+ (low intensity), 106, 132, 117, 106, 99, 88, 75, 73, 62.
Synthesis of 5-methyl-1,3-oxathiolane-2-thione (dithiocarbonate IV). To a solution of propylene oxide (1.53 g, 26.34 mmol, 1 equiv.) in CS2 (5 g, 65.7 mmol, 2.5 equiv.) 0.3 g (1.7 mmol, 0.063 equiv.) of sodium methoxide in methanol was added. The mixture was heated to 30 °C and stirred for the next 8 hours. The precipitate soluble in acetonitrile was filtered off and the filtrate was concentrated to half on a rotary evaporator. The resulting mixture was diluted with 10 ml of brine and the obtained emulsion was extracted with EtOAc (3 × 10 ml). The combined organic phases were dried over sodium sulfate, concentrated under reduced pressure and the viscose mixture was separated via the DCVC technique50 giving the desired dithiocarbonate IV as a caramel colored oil. 1H NMR (600 MHz, CDCl3) δ 5.27–5.15 (m, 1H), 3.67–3.58 (m, 1H), 3.34 (t, J = 10.0 Hz, 1H), 1.59 (d, J = 5.6 Hz, 3H) ppm; 13C NMR (101 MHz, CDCl3) δ 212.32 (s), 88.12 (s), 40.88 (s), 19.33 (s) ppm. FTIR (squeezed drop, ZnSe): 2981, 2932, 2868, 1439, 1383, 1344, 1328, 1244, 1200, 1148 cm−1. MS (EI) [M]+ m/z: 134.
Capturing and analysis of the released gases. To establish the nature of the gases released in the course of the reaction between cyclic carbonates and potassium xanthates, a series of additional experiments was carried out aimed at collecting followed by qualitative analysis of the trapped gases. Toluene and DMSO were chosen as solvents. A glass tube with a ratio of height to inner diameter equal to 30 was used as a chemisorption vessel. Cyclic carbonate placed in a conical flask equipped with a stirring bar and a glass tap and sorbent-toluene placed in a test tube were purged with nitrogen for 30 minutes prior to the reaction run. After that, a prescribed amount of potassium xanthate was added under stirring to homogenize the reaction system. The induction period took from a few seconds to a minute, depending on the free volume of the system and the amount of xanthate added. The released gases were bubbled through a test tube filled with toluene or DMSO using a capillary outlet. The resulting mixture was analyzed with GC-MS.
Conclusions
The peculiarities in kinetics described in this paper of the reaction between five-membered cyclic carbonates (ethylene carbonate and propylene carbonate) and various potassium xanthates and the established precipitation of the final products accompanied by intensive gas evolution indicate the unusual and to the best of our knowledge undescribed mechanism of the studied reaction. It was determined that the distribution between three major products (potassium alkoxides and alkoxide-terminated sulfides and disulfides) varies with a change in the temperature of the synthesis. Summarizing the obtained data, it was found that the fraction of sulfur-containing products increased with an increase in the temperature of the synthesis. As a striking result, a substantial shift in the balance between the components of the precipitate towards sulfide formation (propanediol/sulfide/disulfide = 1/33.5/0.77) under reduced pressure conditions (2–4 mm Hg) was achieved, demonstrating the remarkable selectivity (up to 95%) of the process towards the preparation of potassium 1,1′-thiobis(propan-2-olate). On the other hand, selective preparation (98%) of another product, potassium propane-1,2-bis(olate), was achieved for reactions carried out in the presence of water. The reactions underlying the formation of each of the products were also proposed based on the set of experimental observations supplemented with quantum chemical calculations performed at the m06-2x/6-311++G(d,p)/PCM level of theory. Based on the proposed cascade reactions, the experimentally determined shift in distribution between the products under reduced pressure conditions was attributed to the formation of volatile side-products, such as CS2 and COS, the constant removal of which redirects the reaction towards the selective formation of sulfide (potassium 1,1′-thiobis(propan-2-olate)) molecules. Considering the relatively high reactivity of cyclic sulfides (e.g., propylene sulfide) in ring-opening reactions, the described reaction between propylene carbonate and potassium xanthate could also serve as a valuable tool for the preparation of polysulfides if one could succeed in shifting the balance between products towards the formation of cyclic sulfide. The extent of the shift should lead to the preparation of sulfide-enriched polymeric materials (from homo-to copolymers) with various degrees of sulfide polymerization.
Conflicts of interest
There are no conflicts to declare.
Acknowledgements
The presented results were obtained in the framework of the state task in the field of scientific activity (theme no FSWE-2020-0008).
Notes and references
- D. J. Keddie, A guide to the synthesis of block copolymers using reversible-addition fragmentation chain transfer (RAFT) polymerization, Chem. Soc. Rev., 2014, 43, 496–505 RSC.
- J. Yeow, R. Chapman, A. J. Gormley and C. Boyer, Up in the air: oxygen tolerance in controlled/living radical polymerisation, Chem. Soc. Rev., 2018, 47, 4357–4387 RSC.
- M. R. Hill, R. N. Carmean and B. S. Sumerlin, Expanding the Scope of RAFT Polymerization: Recent Advances and New Horizons, Macromolecules, 2015, 48, 5459–5469 CrossRef CAS.
- M. Destarac, D. Matioszek, X. Vila, J. Ruchmann-Sternchuss and S. Z. Zard, in Reversible Deactivation Radical Polymerization: Mechanisms and Synthetic Methodologies, American Chemical Society, 2018, ch. 13, vol. 1284, pp. 291–305 Search PubMed.
- D. H. R. Barton and S. W. McCombie, A new method for the deoxygenation of secondary alcohols, J. Chem. Soc., Perkin Trans. 1, 1975, 1574–1585, 10.1039/p19750001574.
- F. Coppa, F. Fontaria, F. Minisci, G. Pianese, P. Tortoreto and L. Zhao, A novel radical reaction of alkyl xantates useful for the selective substitution of heteroaromatic bases, Tetrahedron Lett., 1992, 33, 687–690 CrossRef CAS.
- Q. Huang and S. Z. Zard, Inexpensive Radical Methylation and Related Alkylations of Heteroarenes, Org. Lett., 2018, 20, 1413–1416 CrossRef CAS.
- V. L. Revil-Baudard, J.-P. Vors and S. Z. Zard, Xanthate-Mediated Incorporation of Quaternary Centers into Heteroarenes, Org. Lett., 2018, 20, 3531–3535 CrossRef CAS.
- M.-G. Braun, G. Castanedo, L. Qin, P. Salvo and S. Z. Zard, Introduction of Trifluoroethylamine as Amide Isostere by C–H Functionalization of Heteroarenes, Org. Lett., 2017, 19, 4090–4093 CrossRef CAS.
- A. A. Mikhaylov and S. Z. Zard, Extension to the Silyl-Tethered Radical Cyclization: Cyclohex-2-en-1-oxy Vinyl Silanes in Stereoselective Radical Addition/Cyclization Cascades, Org. Lett., 2017, 19, 1866–1869 CrossRef CAS.
- S. Z. Zard, The Xanthate Route to Ketones: When the Radical Is Better than the Enolate, Acc. Chem. Res., 2018, 51, 1722–1733 CrossRef CAS.
- M. Langlais, O. Coutelier and M. Destarac, Thiolactone-Functional Reversible Deactivation Radical Polymerization Agents for Advanced Macromolecular Engineering, Macromolecules, 2018, 51, 4315–4324 CrossRef CAS.
- M. Langlais, O. Coutelier and M. Destarac, Scope and Limitations of Xanthate-Mediated Synthesis of Functional γ-Thiolactones, ACS Omega, 2018, 3, 17732–17742 CrossRef CAS.
- M. Langlais, I. Kulai, O. Coutelier and M. Destarac, Straightforward Xanthate-Mediated Synthesis of Functional γ-Thiolactones and Their Application to Polymer Synthesis and Modification, Macromolecules, 2017, 50, 3524–3531 CrossRef CAS.
- M. Corbet, M. de Greef and S. Z. Zard, A Highly Conjunctive β-Keto Phosphonate: Application to the Synthesis of Pyridine Alkaloids Xestamines C, E, and H, Org. Lett., 2008, 10, 253–256 CrossRef CAS.
- M. Corbet and S. Z. Zard, Facile and Efficient One-Pot Synthesis of Highly Functionalized Thieno[2,3-b]thiopyran-4-ones from β-Keto ε-Xanthyl Phosphonates, Org. Lett., 2008, 10, 2861–2864 CrossRef CAS.
- H. Jullien, B. Quiclet-Sire, T. Tétart and S. Z. Zard, Flexible Routes to Thiophenes, Org. Lett., 2014, 16, 302–305 CrossRef CAS.
- L. Anthore-Dalion and S. Z. Zard, Chemoselective Reduction: Xanthates as Traceless Precursors of Polyfunctionalized α,α-Dichloroketones, Org. Lett., 2017, 19, 5545–5548 CrossRef CAS.
- P. Ostrovskis, A. A. Mikhaylov and S. Z. Zard, Sigmatropic Rearrangement-Based Synthesis of 4-Alkenyl-1,3-dithiol-2-ones, Org. Lett., 2019, 21, 3726–3729 CrossRef CAS.
- S. Sangeetha and G. Sekar, Synthesis of 2-Acylbenzo[b]Thiophenes via Cu-Catalyzed α-C–H Functionalization of 2-Halochalcones Using Xanthate, Org. Lett., 2017, 19, 1670–1673 CrossRef CAS.
- N. Sundaravelu and G. Sekar, Domino Synthesis of Thioflavones and Thioflavothiones by
Regioselective Ring Opening of Donor–Acceptor Cyclopropane Using In-Situ-Generated Thiolate Anions, Org. Lett., 2019, 21, 6648–6652 CrossRef CAS.
- S. Sangeetha and G. Sekar, Copper-Catalyzed One-Pot Synthesis of 2-Arylthiochromenones: An In Situ Recycle of Waste Byproduct as Useful Reagent, Org. Lett., 2019, 21, 75–79 CrossRef.
- P. Muthupandi, N. Sundaravelu and G. Sekar, Domino Synthesis of Thiochromenes through Cu-Catalyzed Incorporation of Sulfur Using Xanthate Surrogate, J. Org. Chem., 2017, 82, 1936–1942 CrossRef CAS.
- D. J. C. Prasad and G. Sekar, Cu-Catalyzed One-Pot Synthesis of Unsymmetrical Diaryl Thioethers by Coupling of Aryl Halides Using a Thiol Precursor, Org. Lett., 2011, 13, 1008–1011 CrossRef CAS.
- S. Sangeetha, P. Muthupandi and G. Sekar, Copper-Catalyzed Domino Synthesis of 2-Arylthiochromanones through Concomitant C–S Bond Formations Using Xanthate as Sulfur Source, Org. Lett., 2015, 17, 6006–6009 CrossRef CAS.
- M. Rumyantsev, S. Rumyantsev, S. I. Kamorina, I. Y. Kalagaev and O. A. Kazantsev, Xanthate functionalized MPEGs as new macro-initiators for the catalyst-, solvent-, and PLA-free controlled synthesis of core-shell forming amphiphilic block copolymers, React. Funct. Polym., 2019, 141, 33–41 CrossRef CAS.
- R. Abdul-Karim, A. Hameed and M. I. Malik, Ring-opening polymerization of propylene carbonate: Microstructural analysis of the polymer and selectivity of polymerization by 2D-NMR techniques, Eur. Polym. J., 2018, 105, 95–106 CrossRef CAS.
- X. Lou, C. Detrembleur, P. Lecomte and R. Jérôme, Two-Step Backbiting Reaction in the Ring-Opening Polymerization of γ-Acryloyloxy-ε-caprolactone Initiated with Aluminium Isopropoxide, Macromol. Rapid Commun., 2002, 23, 126–129 CrossRef CAS.
- S. J. Jeon, M.-y. Jung and J. Y. Do, Anionic ring-opening polymerization of cyclic 1,3-dithiocarbonate and thermal depolymerization, React. Funct. Polym., 2016, 100, 37–43 CrossRef CAS.
- K. Tezuka, K. Komatsu and O. Haba, The anionic ring-opening polymerization of five-membered cyclic carbonates fused to the cyclohexane ring, Polym. J., 2013, 45, 1183–1187 CrossRef CAS.
- J.-C. Lee and M. H. Litt, Ring-Opening Polymerization of Ethylene Carbonate and Depolymerization of Poly(Ethylene Oxide-co-Ethylene Carbonate), Macromolecules, 2000, 33, 1618–1627 CrossRef CAS.
- G. Kapiti, H. Keul and M. Möller, Functional PEG building blocks via copolymerization of ethylene carbonate and tert-butyl glycidyl ether, Polym. Chem., 2016, 7, 5050–5059 RSC.
- S. Kéki, J. Török, G. Deák and M. Zsuga, Ring-Opening Oligomerization of Propylene Carbonate Initiated by the Bisphenol A/KHCO3 System: A Matrix-Assisted Laser Desorption/Ionization Mass Spectrometric Study of the Oligomers Formed, Macromolecules, 2001, 34, 6850–6857 CrossRef.
- G. Kapiti, H. Keul and M. Möller, Organocatalytic polymerization of ethylene carbonate, Mater. Today Commun., 2015, 5, 1–9 CrossRef CAS.
- H. E. Gottlieb, V. Kotlyar and A. Nudelman, NMR Chemical Shifts of Common Laboratory Solvents as Trace Impurities, J. Org. Chem., 1997, 62, 7512–7515 CrossRef CAS.
- M. J. Frisch, G. W. Trucks, H. B. S. Schlegel, G. E. Scuseria, M. A. Robb, J. R. Cheeseman, G. Scalmani, V. Barone, B. Mennucci, G. A. Petersson, et al., Gaussian 03 R. B., Gaussian I., Pittsburgh, PA, 2003 Search PubMed.
- B. Mennucci, J. Tomasi, R. Cammi, J. R. Cheeseman, M. J. Frisch, F. J. Devlin, S. Gabriel and P. J. Stephens, Polarizable Continuum Model (PCM) Calculations of Solvent Effects on Optical Rotations of Chiral Molecules, J. Phys. Chem. A, 2002, 106, 6102–6113 CrossRef CAS.
- B. Mennucci, Polarizable continuum model, Wiley Interdiscip. Rev.: Comput. Mol. Sci., 2012, 2, 386–404 CAS.
- J. Tomasi, B. Mennucci and R. Cammi, Quantum Mechanical Continuum Solvation Models, Chem. Rev., 2005, 105, 2999–3094 CrossRef CAS.
- Y. Takano and K. N. Houk, Benchmarking the Conductor-like Polarizable Continuum Model (CPCM) for Aqueous Solvation Free Energies of Neutral and Ionic Organic Molecules, J. Chem. Theory Comput., 2005, 1, 70–77 CrossRef.
- A. E. Reed and F. Weinhold, Natural bond orbital analysis of near-Hartree–Fock water dimer, J. Chem. Phys., 1983, 78, 4066–4073 CrossRef CAS.
- A. E. Reed, R. B. Weinstock and F. Weinhold, Natural population analysis, J. Chem. Phys., 1985, 83, 735–746 CrossRef CAS.
- E. D. Glendening, C. R. Landis and F. Weinhold, Natural bond orbital methods, Wiley Interdiscip. Rev.: Comput. Mol. Sci., 2012, 2, 1–42 CAS.
- F. Weinhold, C. R. Landis and E. D. Glendening, What is NBO analysis and how is it useful?, Int. Rev. Phys. Chem., 2016, 35, 399–440 Search PubMed.
- F. L. Weinhold and C. R. Landis, Valency and Bonding: A Natural Bond Orbital Donor-Acceptor Perspective, Cambridge University Press, Cambridge, 2005 Search PubMed.
- F. Weinhold and R. A. Klein, What is a hydrogen bond? Resonance covalency in the supramolecular domain, Chem. Educ. Res. Pract., 2014, 15, 276–285 RSC.
- M. Rumyantsev, O. A. Kazantsev, S. Rumyantsev and I. Y. Kalagaev, Quantum chemical study of the impact of protective association on the chemoselective synthesis of carboxybetaine from 2-(dimethylamino)ethanol and acrylic acid, Comput. Theor. Chem., 2018, 1129, 16–25 CrossRef CAS.
- M. Rumyantsev, N. S. Sitnikov and N. V. Somov, Hydrogen-Bond-Assisted Organocatalytic Acetalization of Secondary Alcohols: Experimental and Theoretical Studies, J. Phys. Chem. A, 2015, 119, 4108–4117 CrossRef CAS.
- M. Rumyantsev, S. Rumyantsev and I. Y. Kalagaev, Effect of Water on the Activation Thermodynamics of Deep Eutectic Solvents Based on Carboxybetaine and Choline, J. Phys. Chem. B, 2018, 122, 5951–5960 CrossRef CAS.
- D. S. Pedersen and C. Rosenbohm, Dry Column Vacuum Chromatography, Synthesis, 2001, 2001, 2431–2434 Search PubMed.
Footnote |
† Electronic supplementary information (ESI) available. See DOI: 10.1039/d0ra07428d |
|
This journal is © The Royal Society of Chemistry 2020 |
Click here to see how this site uses Cookies. View our privacy policy here.