DOI:
10.1039/D0RA06620F
(Paper)
RSC Adv., 2020,
10, 38069-38074
High-temperature resistant water-soluble polymers derived from exotic amino acids†
Received
31st July 2020
, Accepted 9th October 2020
First published on 15th October 2020
Abstract
High-performance water-soluble polymers have a wide range of applications from engineering materials to biomedical plastics. However, existing materials are either natural polymers that lack high thermostability or rigid synthetic polymers. Therefore, we design an amino acid-derived building block, 4,4′-diamino-α-truxillate dianion (4ATA2−), that induces water solubility in high-performance polymers. Polyimides containing 4ATA2− units are intrinsically water-soluble and are processed into films cast from an aqueous solution. The resulting polyimide films exhibit exceptional transparency and extremely high thermal stability. In addition, the films can be made insoluble in water by simple post-treatment using weak acid or multivalent metal ions such as calcium. The synthesized polyimide's derived from bio-based resources are useful for yielding waterborne polymeric high-performance applications.
Introduction
Water-soluble polymers have been widely used in water-related application as dispersants, aggregation agents, thickeners, moisturizers, binders and hydrogels.1–5 The majority of these materials are polysaccharides and their derivatives, proteins, or peptides, which can be used for biological applications.6–8 In addition to natural polymers, synthetic water-soluble polymers such as poly(ethylene oxide), poly(vinyl alcohol), polyacrylates, polyacrylamide and their derivatives have been employed in various industries.9 Increased environmental awareness raise have highlighted the value of water-soluble materials and expanded their applications to fields such as electronics, functional coatings and paints, special adhesions, hybridization and biomedical materials.10,11 However, the heat resistance of conventional water soluble polymers remain too poor for advanced industries that require high thermal stability while processing under harsh conditions.3,5 On the contrary, polymers possessing ultrahigh thermal stability, such as polyimides, exhibits poor solubility features. Especially, no effective molecular engineering design system exists for optimizing the water solubility features of polyimides due to their rigid polymer backbone and strong interchain interactions; thereby limits their processability, functionalization and subsequently applications as well. Therefore, exploiting the molecular design through multi-functionality in polyimide structure is expected to induce the water solubility features, which could play a game changer role in the processing of polymers possessing ultrahigh thermal stability.
The purpose of the present work is to illustrate the molecular design of a building block that induces good water-solubility in polyimides. The building block is derived from an exotic but bio-derived amino acid. Experimental results reveal that polyimides with extremely high water-solubility are produced and the processed plastics exhibit high thermomechanical and optical performance. The tunability of the thermomechanical properties based on the size of cation in synthesized polyimide, without dismantling the polymer backbone, represents a very interesting approach for polymer engineering. The importance of multifunctionality in the polyimide backbone for not only inducing the water solubility but also provides the glimpse of trajectory of further research in waterborne materials development has been discussed.
Experimental section
Materials
Dianhydrides including 1,2,3,4-tetracarboxycyclobutane dianhydride (CBDA, Tokyo Chemical Industries (TCI)), 1,2,4,5-tetracarboxycyclohexane dianhydride (CHDA) and 1,2,3,4-tetracarboxycyclopentane dianhydride (CPDA) were purified by sublimation under reduced pressure prior to use. Super dehydrated N,N-dimethylacetamide (DMAc, Kanto Chemical Corporation) employed as a polymerization solvent, and other solvents employed for solubility tests were used as received. The diamine precursor, 4-aminocinnamic acid (4ACA), was received from Dr N. Takaya (Tsukuba University, Japan) and prepared by 4ACA fermentation using genetically manipulated Escherichia coli (E. coli) from glucose according to previously reported method.12
Materials syntheses
4,4′-Diamino-α-truxillic acid (4ATA) dihydrochloride was synthesized by the drop-wise addition of 12 N hydrochloric acid solution (5.6 mL) into the acetone solution (30 mL) of 4ACA (2.0 g, 12.4 mmol) to produce 4-aminocinnamic acid hydrochloride (1.74 g, 4.35 mmol, as shown in Scheme S1†). The obtained product was subjected to irradiation by an ultraviolet LED array (Nikkiso co. Ltd, Tokyo) with a wavelength of 305 ± 5 nm and intensity of 50 mW cm−3 for 6 h to induce [2 + 2] photocycloaddition. The reaction was monitored by 1H NMR (400 MHz, DMSO-d6, δ, ppm) according to the disappearance of the peak representing olefinic protons: 3.82 (dd, 2H, J = 7.7, 9.6 Hz), 4.30 (dd, 2H, J = 7.7, 9.6 Hz), 7.33 (d, 4H, J = 7.7 Hz), 7.45 (d, 4H, J = 7.7 Hz), 10.37 (s, 6H), 12.07 (s, 2H).
The obtained 4,4′-diamino-α-truxillic acid (4ATA) dihydrochloride was dissolved in water and neutralized by 1 N NaOH solution to obtain 4,4′-diamino-α-truxillic acid (1.29 g, 3.64 mmol). The obtained product was dried and re-crystallized to obtain crystal needles. The structure was confirmed by 1H NMR (400 MHz, DMSO-d6, δ, ppm).
The crystals of 4,4′-diamino-α-truxillic acid, (0.20 g, 0.5647 mmol) were dissolved in DMAc (0.8 mL, 9.6 mmol) under a nitrogen atmosphere followed by the addition of dianhydride, CBDA (0.11 g, 0.5647 mmol) (as shown in Scheme 1). The reaction mixture was stirred at room temperature for 48 h to produce a light yellow viscous poly(amic acid) (PAA) solution. The PAA solution was diluted in DMAc, then added slowly to a methanol/water mixture to precipitate PAA fibers, which were filtered and dried in a vacuum at 60 °C for 12 h. The PAA film with a thickness approximately 20 μm was cast from a PAA homogeneous DMAc solution onto a glass plate and heated at 75 °C. The obtained PAA films were subjected to thermal imidization in an oven under reduced pressure by stepwise heating at 100, 150, 200, and 250 °C for 1 h at each step to obtain polyimide films. The procedure was followed with other dianhydrides to synthesize a series of polyimides.
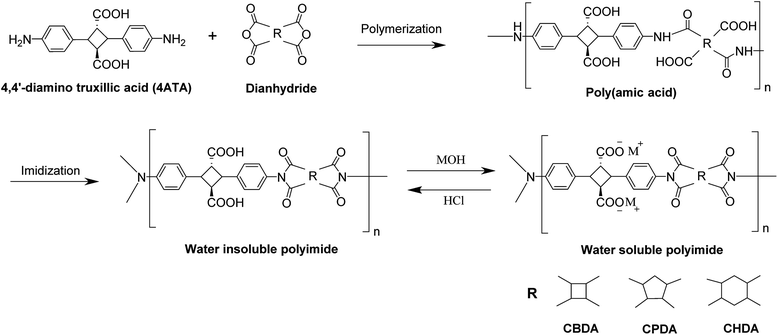 |
| Scheme 1 Synthesis of polyimides derived from 4,4′-diaminotruxillic acid and various dianhydrides and their ionization by subsequent treatments with metal/ammonium hydroxides. | |
The obtained polyimide films were dissolved in an aqueous solution of equimolar amounts of metal/ammonium hydroxide and 4ATA carboxylic acids. The resultant aqueous viscous solution was recast into a flexible and transparent film of polyimide salt.
Characterization
The number averaged molecular weight (Mn), weight averaged molecular weight (Mw), and the molecular weight distribution (PDI) were determined by gel permeation chromatography (GPC, concentration 5 g L−1, DMF eluent, Shodex SB-800HQ, Japan) after calibration with Pullulan Standards. 1H-NMR and 13C-NMR were performed on a Bruker Biospin AG 400 MHz spectrometer using DMSO-d6 as a solvent at 23.1 °C with 16 accumulation scans, using the proton resonance of residual non-deuterated DMSO as an internal standard (2.55 ppm). Fourier transform infrared spectra (FT-IR) of the polymers were recorded in a wave-number range of 4000 to 400 cm−1 on a Perkin-Elmer Spectrum using a diamond-attenuated total reflection (ATR) accessory. Thermogravimetric analysis (TGA) was performed on STA 7200 (Hitachi, Japan) at a heating rate of 10 °C min−1 under a nitrogen atmosphere. The polymer specimens were dried at 100 °C for 1 h to remove any absorbed moisture before TGA measurement. Ultraviolet-visible (UV-Vis) optical absorption spectra were recorded on a Perkin-Elmer, Lambda 25 UV/Vis spectrophotometer at room temperature over the range of 200–800 nm. X-ray diffraction (XRD) was conducted using a monochromated CuKα beam (λ = 0.15418 nm) with an acceleration voltage of 40 kV (30 mA) by 2θ scanning from 5–80° in reflection mode using a rotor X-ray emitter (RINT 2000; Rigaku Smart Lab); this was performed to determine the degree of crystallization. Tensile measurements were carried out at an elongation speed of 0.5 mm min−1 on a tensiometer (Instron 3365) at room temperature. The data of five specimens were averaged to obtain the experimental results.
Results and discussion
Molecular design
Three types of main functional groups are widely utilized to impart water-solubility to polymers; (1) polar groups, (2) a block of poly(ethylene oxide) or its oligomers, and (3) ionic groups.13 Of these, ionic groups have a hydration capability that most efficiently induces the water-solubility into rigid backbones. However, strongly dissociative sulfate groups are easily detached from polymer backbones and do not match the thermoresistant polymers.14 Therefore, carboxylates were selected in this study because of their higher thermostability compared to sulfate and a pH-responsive ionization. 4,4′-diamino-α-truxillic acid (4ATA) has two carboxylic acid side groups and polymerizable amines attached to both ends of rigid triple rings, 1,4-diphenylcyclobutane. The cyclobutane is a rigid yet deformable ring with not very high strain energy and thereby the thermal stability is largely maintained.15,16 V-type structure of cyclobutane resulted in polymer backbone adopting slight zig-zag architecture, where the angle of “V” is around 156° (Fig. 1A) between those of para- and meta-aromatic linkage, which can induce both good solubility and high thermal performances in polymers.
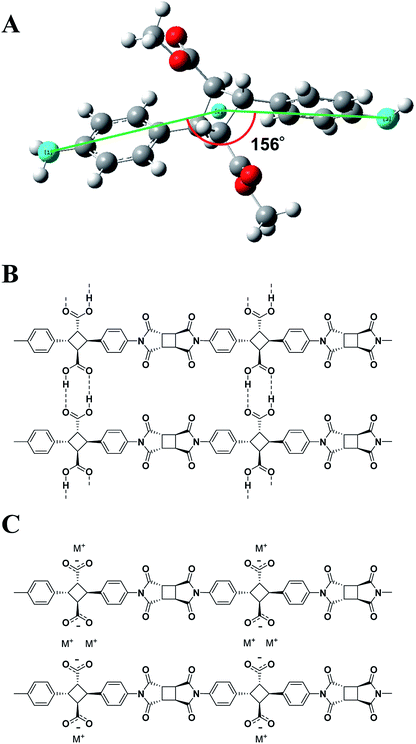 |
| Fig. 1 (A) 4,4′-Diamino-α-truxillic acid showing the angle around cyclobutane core obtained by DFT calculation (Gaussion16). (B) Structures of polyimide chains with an H-type side group and (C) polyimide structure with carboxylate salt side group. | |
Syntheses
4ATA was synthesized by simple photo-coupling, [2 + 2] cycloaddition of 4-amino-trans-cinnamic acid hydrochloride salt (Fig. S1–S3†) with ultraviolet irradiation (λ > 260 nm) and subsequent neutralization (Scheme S1†). Product formation was confirmed by 1H NMR, which indicated the disappearance of the olefinic protons of 4ACA (δ 7.44 to 7.40 and 6.15 to 6.12 ppm) and the appearance of cyclobutane proton signals (δ 4.36 to 3.80 ppm) (Fig. S3†). The photoreaction occurred stoichiometrically and the yield was over 99 wt%. Polycondensation was conducted in a 1
:
1 solution of 4ATA and dianhydride in the super dehydrated DMAc. The increase in viscosity as the reaction progressed indicated the formation of poly(amic acid) (PAA). FT-IR analysis of the PAA fibrils formed as a result of reprecipitation showed a broad signal around 2600–3600 cm−1 (O–H, stretching), two sharp absorption bands at 1720 cm−1 (C–O stretching, carboxylic and ester) and 1670 cm−1 (C–O stretching, amide), and aromatic peaks at 1525 cm−1 and 1432 cm−1 (C–H first overtone, aromatic) (Fig. S4†). Mn and PDI values of the PAA were sufficiently high (4.9 × 105 Da and 2.1, respectively) for all the samples. PAA films were processed by casting from DMAc. Then, successive stepwise annealing yielded polyimide films. The chemical structure of the PI was confirmed by FT-IR. Signals observed at 1375 cm−1 and 1175 cm−1 (C–N stretching, imide) confirmed imide ring formation during the annealing process. Furthermore, the spectra exhibited two peaks for carbonyl at 1785 cm−1 (C
O, asymmetric stretching) and 1716 cm−1 (C
O symmetric stretching) (Fig. S5†).
Solubilization
When the polyimide film was treated by an alkaline solution such as KOH aq, the film became soluble in water. Signals observed at 1375 cm−1 and 1175 cm−1 (C–N stretching, imide) in FT-IR revealed the presence of an imide ring (Fig. S5†). Furthermore, the 13C NMR of the polyimide recorded in deuterium hydroxide exhibited only two peaks at δ 179.6 and 180.5 ppm in the range for carbonyl peaks (Fig. S6†), which were assigned to the imide ring and carboxylate salt, respectively. It is noteworthy that amic acid signals did not re-appear to prevent the ring-opening reaction of imides under this condition. Therefore, we conclude that the carboxylic acid side group of the polyimides was converted into carboxylate anions by alkaline solution treatment of the film (Fig. 1B and C). Solubility of the polyimide in water was very high, at over 2 g of polymer per mL of water (>66 wt%), above which it is impossible to judge the solubility due to the high viscosity. This solubility value was unexpectedly high because polymer containing aromatic rings typically exhibit low solubility. Water-dissolution videos of the films for neat polyimide and its potassium salt are shown in a ESI Video (Fig. 2 and Video S1†). The polyimide K-salts were casted from the aqueous solution to obtain transparent films. Film surface analyses by EDX revealed that the K element was uniformly distributed over the polyimide film, similarly to C, N, and O elements, indicating no contamination of non-ionized PI chains (Fig. S7†). In similar manner, other hydroxide salts such as LiOH, NaOH, CsOH, NH4OH were employed as well to obtain the water-soluble polyimides.
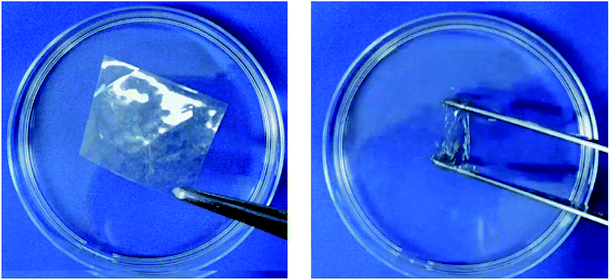 |
| Fig. 2 Water-solubilization of the polyimide film derived from 4,4′-diamino-truxillate potassium salts. Left: transparent polyimide film, right: polyimides immersed in water. | |
The crystallinity was investigated by X-ray diffraction (XRD) (Fig. S8†). The XRD pattern of the polyimide revealed two main diffraction peaks at 2θ = 6° and 17°, which overlapped with a broad amorphous halo. The XRD diagrams of untreated polyimides indicated partial crystallization (crystallization degree of 19%). The polyimide crystallinity was reduced by side chain ionization to 10–14%, decreases with increasing cation size. Typically, in polymers a crystalline structure is formed based by strong interchain interactions such as hydrogen bonding, which is broken by ionization. These results may be envisaged to the greater separation between the polymer layers with increase in the cation size, which disrupts the degree of crystallization.
Physical properties
Thermal stabilities of the polyimides were measured by thermogravimetry, from 25–800 °C at a heating rate of 5 °C min−1 under nitrogen (Fig. S9,† and Table 1). The polyimides without alkaline treatment showed a very high temperature corresponding to 10 wt% (Td10) of 406 °C. Ionization of polyimide side chains reduced Td10 values to 349–366 °C, which decreased with increasing radii of incorporated cations. The reduction of Td10 values by ionization is due to the weakened interchain interactions, as described in the discussion of crystallinity. The polyimide metal salts revealed an ash amount of at least 60 wt% at 800 °C, which was much higher than that of the untreated polyimide (8%) and presumably due to the residual metal oxide. The ash content generally corresponds to self-extinguishment characteristics of the polymers.17 Although we attempted to verify the glass transition temperature of the water-soluble polyimides by DSC, no inflection points were detected below degradation temperatures. Thus, thermoresistance of the water-soluble polyimides was regarded as the thermal degradation temperature, which was much higher than that for conventional water-soluble polymers (PEG: 265 °C,18 PVA: 250–310 °C),19 PNIPAM: <200 °C,20 polysaccharides such as carboxymethyl cellulose and xanthan gum: <300 °C.21
Table 1 Thermo-mechanical and optical properties of the polyimides and their metal/ammonium ion hybrids
Dianhydrides |
Counter cations |
Td5a (°C) |
Td10a (°C) |
Young's moduli (MPa) |
Tensile strengthb (MPa) |
Elongation at breakb (%) |
Yellow indexc (ASTM D1925) |
Measured by TGA. Measured by tensile testing machine. Determined using spectrophotometer, N/A represents brittle film. |
CBDA |
H |
383 |
406 |
3330 |
109 |
3.6 |
3.21 |
Li |
341 |
366 |
1910 |
42 |
8.1 |
3.15 |
Na |
343 |
365 |
1110 |
37 |
10.1 |
3.30 |
K |
350 |
362 |
952 |
29 |
10.9 |
3.22 |
Cs |
332 |
349 |
833 |
21 |
12.4 |
3.49 |
NH4 |
358 |
375 |
N/A |
N/A |
N/A |
N/A |
Al |
330 |
355 |
221 |
22 |
0.081 |
11.47 |
CPDA |
K |
343 |
363 |
N/A |
N/A |
N/A |
N/A |
CHDA |
354 |
374 |
582 |
32 |
0.1 |
3.37 |
The mechanical properties of film specimens for polyimide metal salts were investigated in the elongation mode (Fig. S10†). It was confirmed that the untreated polyimide had high tensile strength (109 MPa) but quite a low degree of elongation at break. In contrast, the polyimide metal salts exhibited lower tensile strength in the range of 21–42 MPa. Moreover, the tensile strength decreased with increase in metal size, due to the reduced intermolecular interactions, as discussed above. However, the degree of elongation was improved to 8.1–12.4% by ionization, which resulted in the film becoming more flexible. It is notable that the degree of elongation improved substantially with an increase in the cationic radii.
Optical properties such as transparency (Fig. S11†) and the yellow index of the polyimide were also investigated. Interestingly, high optical transparency (>82%) was observed even after introduction of the cation to the polyimide films, regardless of cation species. Furthermore, very low yellow indices were recorded (by ASTM method D1925) of approximately 3.2. Monovalent counter ions can be replaced with other metals in the presence of a large amount of corresponding chlorides or nitrates such as CaCl2. Multivalent metal ions such as aluminum resulted in the polyimides becoming water insoluble again to maintain their high performance and transparency (Table 1), indicating an ideal system for water-insoluble film processing over an aqueous solution. When the dianhydrides were changed into CPDA and CHDA, it was possible to obtain the film from the aqueous solutions. The polyimide K-salt film obtained from CHDA also exhibited high thermoresistance, transparency and good water solubility; however, the film obtained from CPDA was too brittle to measure its mechanical or optical properties. Finally, we used the 4ATA2− building block to prepare various polyimides from pyromellitic anhydride (PMDA), oxydiphthalic acid (ODPA), diphenylsulfonetetracarboxylic dianhydrides (DSDA), meso-butane-1,2,3,4-tetracarboxylic dianhydride (mBTDA), and found that all polyimides were water-soluble. Thus 4ATA2− is a very effective building block for designing water-soluble polyimides.
Conclusions
Water-soluble polyimide films with very high thermal performances were prepared using an amino acid-derived diamine monomer with two carboxylate side groups. Another structural characteristic of the monomer is the cyclobutanyl central group, which bends the corresponding polymer backbone slightly. After polymerization of the diamine with dianhydrides such as 1,2,3,4-cyclobutane tetracarboxylic dianhydride, the resulting poly(amic acid)s was imidized by subsequent thermal treatment. The carboxyl side chains of the polyimides were ionized by aqueous solutions of metal hydroxide such as KOH. The polyimide potassium salts were dissolved in water and the film was cast from this aqueous solution to obtain an optically clear film. Other alkaline metals or ammonium hydroxides also induced water solubility to the film. The films of polyimide salts produced with alkaline metals showed high Td10 values of 366 °C although the degradation temperatures were slightly reduced by ionization. Softening temperatures were not detected. Furthermore, the polyimide salt films made with heavier alkaline ions were more ductile. Alkaline metal counter ions can be replaced with the multivalent metal ions and thereby resulting the formation of water insoluble polyimide. A preliminary study into polyurea and polyamide including 4ATA2− units also exhibited water-solubility, which indicates the wide versatility of this building block. The polymers synthesized in this study are attractive soft materials that can be utilized in the fields of such as electronics, functional coatings and paints, special adhesives, and biomedical materials.
Conflicts of interest
All authors have given approval to the final version of the manuscript and have no conflict of interests.
Acknowledgements
The research was financially supported by Advanced Low Carbon Technology Research and Development Program (JST ALCA, JPMJAL1010), Tokyo, Japan.
References
- V. G. Kadajji and G. V. Betageri, Polymers, 2011, 3, 1972–2009 CrossRef CAS.
- N. M. Kumar, K. Varaprasad, K. M. Rao, A. S. Babu, M. Srinivasulu and S. V. Naidu, J. Polym. Environ., 2012, 20, 17–22 CrossRef.
- E. Jalavandi and A. Shavandi, Eur. Polym. J., 2018, 109, 43–54 CrossRef.
- Z. Li and X. J. Loh, Chem. Soc. Rev., 2015, 44, 2865–2879 RSC.
- V. Vajihinejad, S. P. Gumfekar, B. Bazoubandi, Z. R. Najafabadi and J. B. P. Soares, Macromol. Mater. Eng., 2019, 304, 1800526 CrossRef.
- G. Q. Chen, Chem. Soc. Rev., 2009, 38, 2434–2446 RSC.
- G. Q. Chen and Q. Wu, Biomaterials, 2005, 26, 6565–6578 CrossRef CAS.
- B. Hazel and A. Steinbüchel, J. Microbiol. Biotechnol., 2007, 74, 1–12 CrossRef.
- S. Roweton, S. J. Huang and G. Swift, J. Environ. Polym. Degrad., 1997, 5, 175–181 CAS.
- H. Cao, X. Ma, S. Sun, H. Su and T. Tan, Polym. Bull., 2010, 64, 623–632 CrossRef CAS.
- S. D. Alexandratos, Ind. Eng. Chem. Res., 2009, 48, 388–398 CrossRef CAS.
- P. Suvannasara, S. Tateyama, A. Miyasato, K. Matsumura, T. Shimoda, T. Ito, Y. Yamagata, T. Fujita, N. Takaya and T. Kaneko, Macromolecules, 2014, 47, 1586–1593 CrossRef CAS.
- B. L. Rivas, E. D. Pereira and I. Moreno-Villoslada, Prog. Polym. Sci., 2003, 28, 173–208 CrossRef CAS.
- Y. L. Zhao, W. H. Jones, F. Monnat, F. Wudl and K. N. Houk, Macromolecules, 2005, 38, 10279–10285 CrossRef CAS.
- S. Dwivedi and T. Kaneko, Polymers, 2018, 10, 368 CrossRef.
- S. Dwivedi and T. Kaneko, J. Appl. Polym. Sci., 2018, 135, 46709 CrossRef.
- S. Chatterjee, K. Shanmugunathan and G. Kumaraswamy, ACS Appl. Mater. Interfaces, 2017, 51, 44864–44872 CrossRef.
- S. Karaman, A. Karaipekli, A. Sari and A. Biçer, Sol. Energy Mater. Sol. Cells, 2011, 95, 1647–1653 CrossRef CAS.
- Z. Peng and L. X. A. Kong, Polym. Degrad. Stab., 2007, 92, 1061–1071 CrossRef CAS.
- K. Bauri, S. G. Roy, S. Arora, R. K. Dey, A. Goswami, G. Madras and P. De, J. Therm. Anal. Calorim., 2013, 111, 753–761 CrossRef CAS.
- J. Scheirs, G. Camino and W. Tumiatti, Eur. Polym. J., 2001, 37, 933–942 CrossRef CAS.
Footnote |
† Electronic supplementary information (ESI) available. See DOI: 10.1039/d0ra06620f |
|
This journal is © The Royal Society of Chemistry 2020 |