DOI:
10.1039/D0RA06564A
(Paper)
RSC Adv., 2020,
10, 38281-38286
A naphthalimide-based turn-on fluorescence probe for peroxynitrite detection and imaging in living cells†
Received
29th July 2020
, Accepted 11th October 2020
First published on 16th October 2020
Abstract
Peroxynitrite (ONOO−) is a potent biological oxidant that plays a significant role in diverse physiological and pathological processes. A novel fluorescent probe HCA-OH was developed for specific and sensitive detection of peroxynitrite, and displayed a significant fluorescence turn-on signal. With low cytotoxicity and good photostability, the probe HCA-OH could be applied in imaging ONOO− distribution in HepG2 cells and live C. elegans in real time. Therefore, the probe can be a promising tool for imaging in vivo.
Introduction
Peroxynitrite (ONOO−) is one of the most powerful oxidants among reactive nitrogen species (RNS), which is formed from the reaction between nitric oxide (NO) and superoxide radical anion (O2˙−) under physiological conditions.1–4 The highly reactive peroxynitrite can oxidize or nitrate amino acid residues of proteins, such as tyrosine, cysteine, methionine and tryptophan. Modifications of these amino acids potentially alter the protein's function, inducing various diseases.5,6 Peroxynitrite can also oxidize sugars and damage DNA.7 The production and scavenging of peroxynitrite are correlated with the energy metabolism in tumor cells.8 Therefore, robust detection of peroxynitrite in living organisms is useful for understanding its roles in disease pathophysiology.
Fluorescent technique is indispensable in biological imaging.9–16 Because of high selectivity, rapid response and low toxicity, fluorescent probes have been developed for a wide range of substrates, including ONOO−.17–23 Qian's group developed a near-infrared fluorescent probe ONP to track endogenous ONOO− in brains of kainate-induced epileptic seizures.24 Zhang et al. proposed a novel approach for developing stable NIR fluorochromic platform, which was applied in design of NIR fluorescent probes for monitoring ONOO− and evaluating hepatotoxicity.25 Xie and co-workers developed a formamide group as the special trigger for peroxynitrite.26 However, fluorescent probe for ONOO− imaging are typically restricted for cell based imaging studies. It would be a significant step forward to further develop probes for whole organism, for which C. elegans was an excellent and wide-accessible model.27–29
In this paper, a novel fluorescent probe HCA-OH is designed by tethering p-aminophenol to 1,8-naphthalimide directly (Scheme 1). The p-aminophenol moiety has been proved to be an oxidant-sensitive moiety via N-/O-dearylation.30–33 1,8-naphthalimide fluorophore was used due to its good photostability and high fluorescence quantum yield.34 Upon oxidation of ONOO−, the probe HCA-OH could release the fluorophore HCA-NH2 and the fluorescence was restored. The probe HCA-OH was readily synthesized through in two steps (Scheme 2). Its structure was confirmed by 1H- and 13C-NMR and HRMS. We also obtained the single crystal structure of the probe HCA-OH (Table S1†).
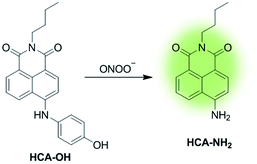 |
| Scheme 1 Structure and mechanism of HCA-OH for ONOO−. | |
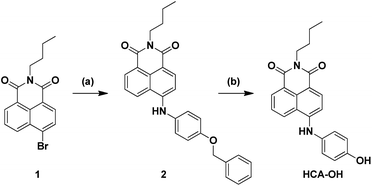 |
| Scheme 2 Synthetic route of probe HCA-OH. Reagents and conditions: (a) 4-benzyloxy aniline, BINAP, Pd (dba)3, t-BuONa, toluene, 90 °C, 12 h. (b) BBr3, CH2Cl2, 6 h. | |
Experimental section
General procedure
Chemical reagents and solvents applied in this work were purchased from commercial suppliers and directly used without further purification. High resolution mass spectra (HRMS) were recorded using a LC-MS 2010A (Shimadzu) instrument. 1H NMR and 13C NMR spectra were measured on a Bruker AV-500 NMR spectrometer. Absorption spectra were operated on a UV-2501PC spectrophotometer. Fluorescence emission spectra were measured on a RF-5301PC spectrophotometer. The fluorescence images of cells and C. elegans were obtained using ZOE™ Fluorescent Cell Imager (1450031EDU) by Bio-Rad.
Synthesis of compound 2
A mixture of compound 1 (700.0 mg, 2.11 mmol), 4-benzyloxy aniline (400.0 mg, 2.0 mmol), BINAP (65.0 mg, 0.11 mmol), Pd(dba)3 (18.0 mg, 0.02 mmol) and sodium tertiary butoxide (960.0 mg, 9.99 mmol) were dissolved in 20 mL toluene in a single necked flask. The reaction mixture was heated to 90 °C and stirred for 12 h. After cooling to room temperature, the solution was removed under reduced pressure. The residue was dissolved in ethyl acetate and washed with brine tree times, then dried over Na2SO4. After filtered and concentrated, the crude product was purified by flash silica gel chromatography (ethyl acetate/petroleum ether = 1/6) to obtain compound 2 (710 mg, 75%). 1H NMR (500 MHz, CDCl3) δ 8.60 (dd, J = 8.6, 1.0 Hz, 1H), 8.37 (d, J = 8.4 Hz, 1H), 8.26 (dd, J = 8.4, 1.0 Hz, 1H), 7.68 (dd, J = 7.78, 4.16 Hz, 1H), 7.47 (d, J = 7.0 Hz, 2H), 7.43–7.40 (m, 2H), 7.37–7.34 (m, 1H), 7.25 (d, J = 2.0 Hz, 1H), 7.24 (d, J = 2.0 Hz, 1H), 7.06 (d, J = 2.0 Hz, 1H), 7.05 (d, J = 2.2 Hz, 1H), 7.02 (d, J = 8.42 Hz, 1H), 6.78 (s, 1H), 5.11 (s, 2H), 4.17–4.14 (m, 2H), 1.74–1.67 (m, 2H), 1.46 (dd, J = 15.2, 7.5 Hz, 2H), 0.99 (t, J = 7.4 Hz, 3H). 13C NMR (125 MHz, CDCl3) δ 164.60, 164.07, 154.61, 153.09, 144.86, 137.05, 132.68, 131.54, 131.07, 130.29, 128.71, 128.14, 127.62, 126.68, 125.63, 123.29, 122.31, 118.77, 117.20, 116.03, 70.54, 43.30, 40.22, 30.41, 20.55, 14.00.
Synthesis of probe HCA-OH
Compound 2 (30.0 mg, 0.067 mmol) was dissolved in 3 mL of CH2Cl2, then BBr3 (50.0 mg, 0.2 mmol) was slowly added to the mixture. The reaction was stirred in an ice-water bath for 6 h and monitored by TLC analysis. After all starting material got consumed, water was added to quench the reaction, and filtered. Compound HCA-OH was obtained as a red solid (20.0 mg, 83%).
1H NMR (500 MHz, DMSO-d6) δ 9.54 (s, 1H), 9.20 (s, 1H), 8.76 (d, J = 8.0 Hz, 1H), 8.40 (d, J = 7.0 Hz, 1H), 8.14 (d, J = 8.5 Hz, 1H), 7.68 (t, J = 8.0 Hz, 1H), 7.18 (d, J = 8.5 Hz, 2H), 6.87 (d, J = 8.5 Hz, 2H), 6.82 (d, J = 8.5 Hz, 1H), 3.96–3.95 (m, 2H), 1.58–1.52 (m, 2H), 1.34–1.27 (m, 2H), 0.89 (t, J = 7.5 Hz, 3H). 13C NMR (125 MHz, DMSO-d6) δ 163.65, 162.77, 155.21, 149.69, 133.65, 130.77, 129.48, 128.67, 126.39, 124.61, 121.87, 120.56, 116.15, 109.32, 105.99, 39.02, 29.79, 19.84, 13.74 HRMS (ESI) (m/z): [M + H]+ calcd for C22H21N2O3 361.1547; found, 361.1543.
General procedure for ONOO− detection
All fluorescence measurements were performed in 10 mM PBS buffer (pH 7.4, containing 1% DMSO). Fluorescence titration studies were performed by addition of an aliquot stock solution of various interfering species into HCA-OH solution. The mixture solution was incubated at 37 °C for 20 min before tested. Samples for absorption and fluorescence measurements were contained in 1 cm × 1 cm quartz cuvettes (3.5 mL volume). Excitation wavelength was 460 nm. The excitation and emission slits were 5 nm.
Culture of cell and C. elegans
HepG2 cells were cultured in Dulbecco's modified Eagle's medium (DMEM) supplemented with 10% fetal bovine serum (FBS) at 37 °C in a humidified atmosphere of 95% air (20% O2) and 5% CO2. For probe loading, the cells were washed with D-Hanks and then incubated with 3 μM HCA-OH (containing 1% DMSO in serum-free DMEM) for 45 min at 37 °C. For imaging the exogenous ONOO−, the cells were pre-treat with ONOO− solutions (15 and 30 μM, respectively) for 1 h in DMEM medium at 37 °C and washed three times with pre-warmed PBS buffer, then incubated with probe HCA-OH (3 μM) 1 h at 37 °C. Last, cells were washed with pre-warmed PBS buffer three times for cell imaging.
The larval stage 4 (L4) C. elegans was first washed with M9 three times by centrifugation at 3200 rpm for 3 min. Then the C. elegans was divided into three groups, every group was incubated with 1000 μL M9 and HCA-OH (final concentration 3 μM) at 20 °C for 2 h. To expose the C. elegans to ONOO−, different amounts of ONOO− were added to each group and the groups incubated for another 2 h. Before imaging, the C. elegans were washed three times with M9, then centrifuged at 3200 rpm for 3 min.
Cytotoxicity assay of probe
HepG2 cells (5 × 104 cells per mL) were planted in 96-well plates in DMEM with 10% FBS for 24 h (5% CO2/95% air, 37 °C). Next, plates were incubated in different concentrations of the probe from 0 μM to 320 μM (containing 1% DMSO in 100 μL DMEM). Subsequently, MTT solution (5.0 mg mL−1, PBS) was added to each well and diluted 10 times before added. Then cells were incubated for 4 h under the same conditions and 150 μL DMSO was added in each well. After 10 min of shaking, the absorbance was measured at 490 nm using a microplate reader.
Results and discussion
Fluorescence detection of ONOO−
The reactivity of HCA-OH toward ONOO− were carried out in PBS (10 mM, pH 7.4, 1% DMSO). Without ONOO−, the free probe HCA-OH was almost non-fluorescent. Upon addition of ONOO−, the probe HCA-OH showed a dramatic fluorescent enhancement at 548 nm, and the absorption maxima blue-shifted from 460 nm to 431 nm (Fig. 1a and S2†). This suggested that the p-aminophenol moiety of the probe was oxidized and N-dearylated and the fluorophore HCA-NH2 was generated.
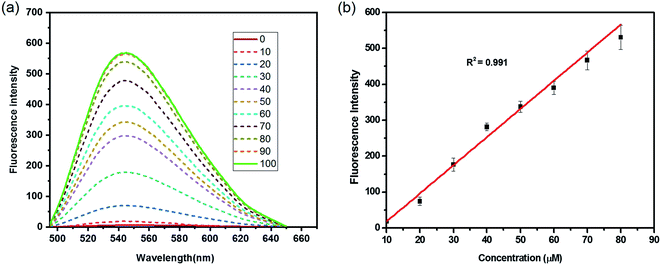 |
| Fig. 1 (a) Fluorescence spectra of HCA-OH (10 μM) with ONOO− titration (0–100 μM). (b) Linear–correlation between fluorescence intensity of HCA-OH at 548 nm and concentrations of ONOO− (0–80 μM). The excitation wavelength was 460 nm. | |
The dose-dependent spectral response between the concentration of ONOO− and fluorescence intensity at 548 nm was also studied (Fig. 1b). Fluorescence response of HCA-OH to ONOO− displayed a linear relationship (R2 = 0.991) in the range of 10 to 80 μM. And the limit of detection (LOD), calculated according to the 3σ/K, where K is the slope of the calibration curve and σ is the standard deviation (n = 11), is estimated to be 49.7 nM. The probe also showed a time dependent fluorescent enhancement. Fluorescence intensity increased immediately after the addition of ONOO− and reached the plateau within 12 min (Fig. 2), which remained stable in further 170 nm (Fig. S3†). These results indicated that the probe HCA-OH could sensitively detect ONOO− under physiological conditions.
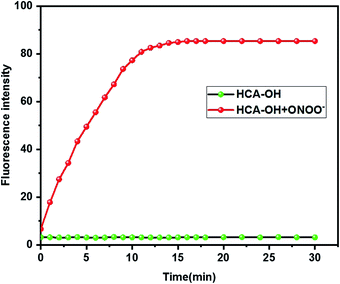 |
| Fig. 2 Time-dependent changes in the fluorescence intensity of HCA-OH (black line: 0 μM ONOO−, red line: 30 μM ONOO−). The excitation and emission wavelength were 460 nm and 548 nm, respectively. | |
Mechanism study
LC-MS was used to explore the mechanism of HCA-OH oxidation (Fig. S4†). The pure probe HCA-OH and fluorophore HCA-NH2 were tested as control, which showed peaks with retention time at 1.63 min and 3.87 min, respectively. The reaction solutions of the probe HCA-OH and ONOO− were mixed and incubated for 10 min and 20 min respectively, before injection for analysis. With the extension of reaction time, the peak of HCA-OH in 1.63 min decreased and the peak of HCA-NH2 in 3.87 min increased gradually. The MS spectroscopy showed appearance of a new peak of at m/z 269.2, which was consistent with the [HCA-NH2 + H]+. These results confirmed that the fluorophore HCA-NH2 was released from the probe by the oxidation of ONOO−.
To further study the nature of the electronic transitions and electronic structures of HCA-OH, time-dependent density functional theory (TD-DFT) calculations on molecular orbital distribution of optimized structures of HCA-OH were carried out at B3LYP/6–311++g(d.p) (Fig. 3 and Table S2†). The calculation results of the S0 states indicated that the major low-energy absorptions were predominantly involved in the electronic transitions from the HOMO to LUMO. The HOMO for the calculated compound were mainly localized on the naphthalimide nucleus and phenol, and the LUMO were populated on the naphthalimide moiety. The proportion of the S0 state's phenol moiety in the LUMO increased up to 70% compared to that in the HOMO, indicating that the low-energy electronic transitions for the HCA-OH originated from π–π* electronic transitions. Furthermore, the theoretical results for the S1 optimized structures of this compound showed that the major contribution to the low-energy emission was associated with a HOMO → LUMO transition. These results also revealed the π–π* transitions to exhibit considerable ICT character, which were identical to the phenomena observed for the S0 optimized structures of this compound. The difference in the HOMO energies between S0 state and S1 state is 1.584 eV, whereas the difference in the LUMO energies are 3.333 eV. The energy gap in S0 state is 6.797 eV, whereas it is only 1.880 eV in S1 state.
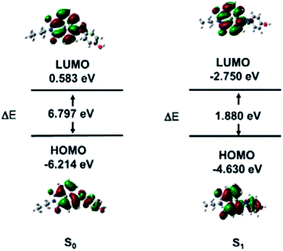 |
| Fig. 3 HOMO and LUMO of HCA-OH obtained by DFT method. | |
In addition, the theoretical calculation results exhibited that the contributions of the –NH– to HOMO levels as well as the electronic effects of phenol to LUMO levels distinctly demonstrate that fluorescence color of compound can be systematically tuned.
Selectivity of probe HCA-OH and the effect of pH
The selectivity of probe HCA-OH was investigated. The probe HCA-OH was treated with various potentially interfering species in PBS buffer (10 mM, pH 7.4, 1% DMSO) at 37 °C. As shown in Fig. 4, ONOO− induced a significant fluorescence enhancement at 548 nm. In contrast, other species, including Na+, K+, Mg2+, Cu2+, Fe2+, Fe3+, Ca2+, Cl−, S2O32−, SO42−, HSO3−, NO3−, Cys, HCy, GSH, ˙OH, t-BuOOH, 1O2, NO, NO2−, H2O2, O2˙−, ClO−, induced nearly no fluorescence changes even after 20 min at 37 °C.
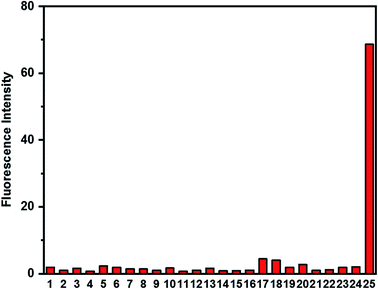 |
| Fig. 4 Fluorescence responses of HCA-OH toward 100 μM analytes unless otherwise noted: (1) blank, (2) Na+, (3) K+, (4) Mg2+, (5) Cu2+, (6) Fe2+, (7) Fe3+, (8) Ca2+, (9) Cl−, (10) S2O32−, (11) SO42−, (12) HSO3−, (13) NO3−, (14) Cys (5 mM), (15) HCy (5 mM), (16) GSH (5 mM), (17) ˙OH, (18) t-BuOOH, (19) 1O2, (20) NO, (21) NO2−, (22) H2O2, (23) O2˙−, (24) ClO−, (25) ONOO− (30 μM). The final concentration of HCA-OH was 10 μM. All experiments data were collected in PBS buffer (10 mM, pH 7.4) with 1% DMSO at 37 °C. The excitation and emission wavelength were 460 nm and 548 nm, respectively. | |
These results showed that the probe could be used to specially detect ONOO− in presence of other biological species. The effect of pH on the response of the probe to ONOO− was also investigated (Fig. S5†). The fluorescence intensity of the probe HCA-OH at 548 nm remained weak from the pH range of 2.0 to 11.0. And a bright fluorescence of the reaction product between the probe and ONOO− was also insensitive to pH in the range of 2 to 11.0, indicating that the detection of ONOO− by the probe could not be effected by the pH value. The excellent selectivity and pH-insensitivity verified that the probe HCA-OH has great potential in living imaging.
Fluorescence imaging of exogenous ONOO− in HepG2
We evaluated the cytotoxicity of the probe HCA-OH (0–320 μM) in HepG2 cells. The MTT assay showed that the probe HCA-OH displayed low cytotoxicity (Fig. S6†). Then, the probe HCA-OH was applied to detect intracellular ONOO− in HepG2 cells. As showed in Fig. 5d, HepG2 cells incubated only with the probe HCA-OH (3 μM) displayed ignorable fluorescence. In contrast, HepG2 cells pretreated with ONOO− (15 μM and 30 μM, respectively) showed a remarkable fluorescence enhancement (Fig. 5e and f). An average emission value of fluorescent photos at different concentrations of ONOO− were obtained. The emission intensity of cells was promoted by 30 μM ONOO− than 15 μM ONOO− (Fig. 4g). These results demonstrated that HCA-OH could be used for detection of ONOO− in living cells.
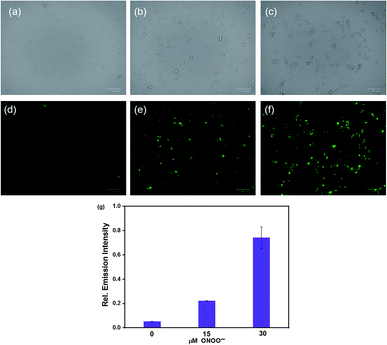 |
| Fig. 5 Fluorescent imaging of exogenous ONOO− in HepG2 cells. Top: bright field images. Bottom: fluorescent images. (a and d) Cells were incubated with probe HCA-OH (3 μM) as control (b and e) Cells were incubated with the probe HCA-OH (3 μM) and ONOO− (15 μM). (c and f) Cells were incubated with the probe HCA-OH (3 μM) and ONOO− (30 μM). (g) Mean fluorescence intensity of images. The excitation wavelength was 463–497 nm, and the fluorescent emissions were collected from 430 to 495 nm window. | |
Imaging of ONOO− in C. elegans
The excellent fluorescence properties of HCA-OH prompted us to test its potential application for imaging ONOO− in living C. elegans. C. elegans were incubated with HCA-OH (3 μM) for 2 h, a weak fluorescence was observed in the green channel (Fig. 6d). Whereas, addition of ONOO− leaded the appearance of fluorescence signals. With the increase of ONOO− concentration, the fluorescence became much brighter (Fig. 6e and f), indicating that HCA-OH could be absorbed by the C. elegans and distributed throughout the whole organism. We quantified the relative fluorescence intensity of images at different ONOO− concentration. A dose-dependent fluorescence intensity enhancement was clearly exhibited (Fig. 6g). These results revealed that HCA-OH could be a suitable probe to monitor ONOO− in C. elegans.
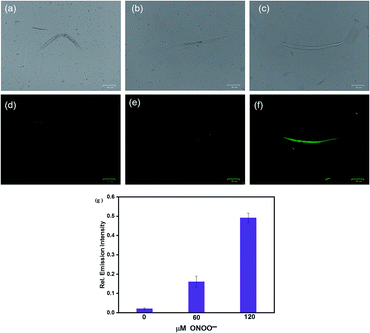 |
| Fig. 6 Fluorescent imaging of ONOO− in C. elegans. Top: bright field images. Bottom: fluorescent images. (a and d) C. elegans were incubated with probe HCA-OH (3 μM). (b and e) C. elegans were incubated with probe HCA-OH (3 μM) and ONOO− (60 μM). (c and f) C. elegans were incubated with probe HCA-OH (3 μM) and ONOO− (120 μM). (g) Mean fluorescence intensity of images. The excitation wavelength was 463–497 nm, and the fluorescent emissions were collected from 430 to 495 nm window. | |
Conclusions
In summary, we have developed a novel naphthallimide-based fluorescent probe HCA-OH for ONOO−. The good stability of its fluorescence towards the pH from 2.0 to 11.0 made the probe can be used in physiological condition. With a dramatic fluorescent turn-on signal, the fluorescent probe could sensitively detect ONOO− over other ROS/RNS. In addition, the probe HCA-OH, possessing good cell permeability and low cytotoxicity, was suitable for monitor ONOO− in C. elegans and HepG2 cells. Therefore, the probe HCA-OH has potential application for imaging in living organisms.
Conflicts of interest
There are no conflicts to declare.
Acknowledgements
The work was supported by the National Natural Science Foundation of China (grant number 21602181, 21702171) and China Postdoctoral Science Foundation (grant number 2016M602628).
Notes and references
- C. Szabo, H. Ischiropoulos and R. Radi, Nat. Rev. Drug Discovery, 2007, 6, 662–680 CrossRef CAS
. - A. Kamm, P. Przychodzen, A. Kuban-Jankowska, D. Jacewicz, A. M. Dabrowska, S. Nussberger, M. Wozniak and M. Gorska-Ponikowska, Nitric Oxide, 2019, 93, 102–114 CrossRef CAS
. - R. Radi, Proc. Natl. Acad. Sci. U. S. A., 2018, 115, 5839–5848 CrossRef CAS
. - P. Pacher, J. S. Beckman and L. Liaudet, Physiol. Rev., 2007, 87, 315–424 CrossRef CAS
. - J. S. Beckman and W. H. Koppenol, Am. J. Physiol., 1996, 271, C1424–C1437 CrossRef CAS
. - R. Radi, J. Biol. Chem., 2013, 288, 26464–26472 CrossRef CAS
. - L. J. Ignarro and B. A. Freeman, Nitric Oxide: Biology and Pathobiology, Academic Press Ltd-Elsevier Science Ltd, London, 3th edn, 2017 Search PubMed
. - J. C. Pestoni, S. Klingeman Plati, O. D. Valdivia Camacho, M. A. Fuse, M. Onatunde, N. A. Sparrow, M. A. Karajannis, C. Fernandez-Valle and M. C. Franco, J. Biol. Chem., 2019, 294, 11354–11368 CrossRef CAS
. - Q. Gong, W. Shi, L. Li and H. Ma, Chem. Sci., 2016, 7, 788–792 RSC
. - C. Y. Wang, S. P. Zhang, J. H. Huang, L. Cui, J. Hu and S. Y. Tan, RSC Adv., 2019, 9, 21572–21577 RSC
. - J. Lv, F. Wang, J. Qiang, X. Ren, Y. Chen, Z. Zhang, Y. Wang, W. Zhang and X. Chen, Biosens. Bioelectron., 2017, 87, 96–100 CrossRef CAS
. - L. Wu, A. C. Sedgwick, X. Sun, S. D. Bull, X.-P. He and T. D. James, Acc. Chem. Res., 2019, 52, 2582–2597 CrossRef CAS
. - T. C. Pham, Y. K. Kim, J. B. Park, S. Jeon, J. Ahn, Y. Yim, J. Yoon and S. Lee, ChemPhotoChem, 2019, 3, 1133–1137 CrossRef CAS
. - Y. Chen, T. Wei, Z. Zhang, W. Zhang, J. Lv, T. Chen, B. Chi, F. Wang and X. Chen, Chin. Chem. Lett., 2017, 28, 1957–1960 CrossRef CAS
. - C. Chang, F. Wang, J. Qiang, Z. Zhang, Y. Chen, W. Zhang, Y. Wang and X. Chen, Sens. Actuators, B, 2017, 243, 22–28 CrossRef CAS
. - J. Lv, Y. Chen, F. Wang, T. Wei, Z. Zhang, J. Qiang and X. Chen, Dyes Pigm., 2018, 148, 353–358 CrossRef CAS
. - X. Chen, X. Tian, I. Shin and J. Yoon, Chem. Soc. Rev., 2011, 40, 4783–4804 RSC
. - X. Chen, F. Wang, J. Y. Hyun, T. Wei, J. Qiang, X. Ren, I. Shin and J. Yoon, Chem. Soc. Rev., 2016, 45, 2976–3016 RSC
. - M. Zhu, H. Zhou, D. Ji, G. Li, F. Wang, D. Song, B. Deng, C. Li and R. Qiao, Dyes Pigm., 2019, 168, 77–83 CrossRef CAS
. - Z. Wang, L. Wu, Y. Wang, M. Zhang, Z. Zhao, C. Liu, Q. Duan, P. Jia and B. Zhu, Anal. Chim. Acta, 2019, 1049, 219–225 CrossRef CAS
. - W. Shu, Y. Wu, T. Shen, J. Cui, H. Kang, J. Jing and X. Zhang, Dyes Pigm., 2019, 170, 107609 CrossRef CAS
. - Y. Shen, M. Li, M. Yang, Y. Zhang, H. Li and X. Zhang, Spectrochim. Acta, Part A, 2019, 222, 117230 CrossRef CAS
. - Z. Li, C. Liu, C. Yu, Y. Chen, P. Jia, H. Zhu, X. Zhang, Y. Yu, B. Zhu and W. Sheng, Analyst, 2019, 144, 3442–3449 RSC
. - J. S. Hu, C. Shao, X. Wang, X. Di, X. Xue, Z. Su, J. Zhao, H. L. Zhu, H. K. Liu and Y. Qian, Adv. Sci., 2019, 6, 1900341 CrossRef
. - D. Cheng, J. Peng, Y. Lv, D. Su, D. Liu, M. Chen, L. Yuan and X. Zhang, J. Am. Chem. Soc., 2019, 141, 6352–6361 CrossRef CAS
. - X. Xie, G. Liu, X. Su, Y. Li, Y. Liu, X. Jiao, X. Wang and B. Tang, Anal. Chem., 2019, 91, 6872–6879 CAS
. - A. Miranda-Vizuete and E. A. Veal, Redox Biol., 2017, 11, 708–714 CrossRef CAS
. - C. F. Labuschagne and A. B. Brenkman, Ageing Res. Rev., 2013, 12, 918–930 CrossRef CAS
. - D. Shi, S. Chen, B. Dong, Y. Zhang, C. Sheng, T. D. James and Y. Guo, Chem. Sci., 2019, 10, 3715–3722 RSC
. - R. K. Jackson, Y. Shi, X. Yao and S. C. Burdette, Dalton Trans., 2010, 39, 4155–4161 RSC
. - T. Peng, N.-K. Wong, X. Chen, Y.-K. Chan, D. H.-H. Ho, Z. Sun, J. J. Hu, J. Shen, H. El-Nezami and D. Yang, J. Am. Chem. Soc., 2014, 136, 11728–11734 CrossRef CAS
. - X. Li, J. Hou, C. Peng, L. Chen, W. Liu and Y. Liu, RSC Adv., 2017, 7, 34287–34292 RSC
. - X. Song, W. Hu, D. Wang, Z. Mao and Z. Liu, Analyst, 2019, 144, 3546–3551 RSC
. - R. M. Duke, E. B. Veale, F. M. Pfeffer, P. E. Kruger and T. Gunnlaugsson, Chem. Soc. Rev., 2010, 39, 3936–3953 RSC
.
Footnote |
† Electronic supplementary information (ESI) available. CCDC 1974831. For ESI and crystallographic data in CIF or other electronic format see DOI: 10.1039/d0ra06564a |
|
This journal is © The Royal Society of Chemistry 2020 |