DOI:
10.1039/D0RA06439D
(Paper)
RSC Adv., 2020,
10, 34910-34915
Alcohol amine-catalyzed CO2 conversion for the synthesis of quinazoline-2,4-(1H,3H)-dione in water†
Received
24th July 2020
, Accepted 31st August 2020
First published on 21st September 2020
Abstract
The conversion of CO2 to high value-added chemicals in water using environment-friendly and cost-effective catalysts is a very significant topic. In this work, a green method for the conversion of CO2 catalyzed by alcohol amines has been developed. Alcohol amines showed considerable activating ability to CO2 in the cyclization with 2-aminobenzonitrile to quinazoline-2,4(1H,3H)-dione in water. Notably, when diethanolamine (DEA) was used as the catalyst, 94% yield of quinazoline-2,4-(1H,3H)-dione could be achieved. A plausible mechanism has been proposed based on the 1H NMR, FT-IR analysis and DFT calculation. The excellent catalytic performance is attributed to the combined effect of both the secondary amine and hydroxyl groups on alcohol amines with the assistance of water in the formation of carbamate. Water plays a bi-functional role of solvent and co-catalyst in this catalytic process. Catalysts can be easily recovered and reused five times without significant loss of activity.
Introduction
Carbon dioxide (CO2) chemistry, including CO2 capture and conversion, is regarded as one of the most attractive research hotspots in chemical and environmental sciences.1,2 The utilization of CO2 as an economical C1 building block for producing chemicals and materials meets the requirement of environment protection and sustainable development.3,4 Numerous processes and corresponding catalysts have been developed in the utilization of CO2 for the production of chemical feedstock carbonates and methanol, such as medical intermediate oxazolidinone and quinazolinone.5 Despite these significant advances, the design and development of new catalysts for the conversion of CO2 is still challenging due to its high thermodynamic stability.
Quinazoline-2,4(1H,3H)-dione and its derivatives are important intermediates as key building blocks in the synthesis of remedial drugs for heart disease and hypertension.6,7 They are usually prepared with toxic and environment-unfriendly reagents, such as phosgene,8 potassium cyanate9 and isocyanate.10 Recently, it has been reported that quinazoline-2,4(1H,3H)-dione could be prepared via the coupling of CO2 and 2-aminobenzonitriles. This avoids the use of noxious reagents and improves the atom economy.11–13 Diverse catalysts, such as organic bases,14–16 inorganic bases,17 ionic liquids,18–24 NHC,25 amidato lanthanide complexes26 and heterogeneous catalysts,27–30 have been developed to promote this reaction. Among various catalysts, organic bases have attracted much attention due to their high efficiency, simplicity and commercial availability. Mizuno et al. demonstrated that nitrogen-containing organic bases were effective in catalyzing this reaction, especially those organic alkalis with steric hindrance, such as 1,8-diazabicyclo[5.4.0]undec-7-ene (DBU) and 1,5,7-triazabicyclo[4.4.0]dec-1-ene (TBD).13 Quinazoline-2,4(1H,3H)-diones could also be prepared using N-methyltetrahydropyrimidine (MTHP) or its polymer as a catalyst at the atmospheric pressure. When poly(amidine) was used as a catalyst, it could be easily recycled and reused for several times owing to its heterogeneity. However, it should be pointed out that all these results were obtained in organic solvents.14 It is of great academic and economic importance if a process proceeds in an organic-solvent-free system. Based on this consideration, He et al. used guanidines as catalysts for the chemical fixation of carbon dioxide with 2-aminobenzonitrile under solvent-free conditions. The catalyst worked well for a variety of 2-aminobenzonitriles.15 Han et al. reported the reaction of CO2 with 2-aminobenzonitrile by the coupling of carbonic acid and substrate in water using choline hydroxide as the catalyst. This process was carried out at 160 °C and 14 MPa.31 All these achievements in the CO2 utilization are quite enlightening. However, organic solvents, expensive catalysts and severe conditions are still required. Developing reasonable and effective catalysts for the chemical fixation of CO2 through the carbonylation and cyclization to prepare quinazoline-2,4(1H,3H)-diones is still a desirable work, which is a great challenge.
Ethanolamines, which are commercially-available, environment-friendly and cost-effective, are widely used as effective acid gas absorbents in the effluent treatment system of modern industrial processes.32,33 The abundant hydroxyl groups of ethanolamines decrease the vapor pressure and amine groups help in absorbing CO2 and SO2 rapidly.34–37 In this manuscript, the CO2-philic and cost-effective ethanolamines were used as catalysts for the reaction of CO2 and 2-aminobenzonitrile to prepare quinazoline-2,4(1H,3H)-dione with water as the solvent (Scheme 1). Among the selected ethanolamines, DEA showed excellent catalytic ability and good recyclability under mild conditions. Water, as the optimum solvent, played an important role in the catalytic process. A plausible mechanism was proposed based on reaction results and DFT calculations.
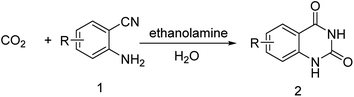 |
| Scheme 1 Reaction of CO2 and 2-aminobenzonitrile to produce quinazoline-2,4(1H,3H)-dione. | |
Experimental
Materials and instrumentation
CO2 with the purity of 99.99% was supplied by Shanghai Pujiang special gas company. Diethanolamine, 1,1,3,3-tetramethylguanidine and 1,4-diazabicyclo[2.2.2]octane were purchased from TCI. Various derivatives of 2-aminobenzonitrile were purchased from Alfa Aesar. Other organic bases and solvents were purchased from Sinopharm Chemical Reagent Co., Ltd. All reagents were directly used without further purification.
The structures of the products were identified by NMR and were consistent with those reported in the literature. 1H NMR and 13C NMR studies were carried out with a Bruker DRX500 spectrometer at 500 MHz in DMSO-d6 with TMS as an internal standard. The characterization data of the products (2a–2e) are listed in the ESI.† IR spectra were recorded on a Nicolet NEXUS 670 infrared spectrometer.
Typical procedure for the synthesis of quinazoline-2,4(1H,3H)-diones
In a typical experiment, diethanolamine (3 mmol), 2-aminobenzonitrile (5 mmol) and water (3 mL) were placed in a 25 mL stainless-steel autoclave equipped with a magnetic stirrer. CO2 was then charged into the autoclave to remove the pre-existing air. The autoclave was placed on a heating equipment and heated to a specified temperature. Then, CO2 was adjusted to the desired pressure, and the reaction continued with stirring for 6–18 h. After completion of the reaction, the autoclave was placed in ice water for 30 min, and then the residual CO2 was released. By adding 10 mL water, the light-yellow product was precipitated and the catalyst was dissolved. The product was washed with water (3 × 25 mL) and tert-butyl methyl ether (3 × 15 mL) to remove impurities. Finally, the mass of the dried product was determined by an electronic balance.
To investigate the reusability of the catalyst, DEA was separated from the product by filtration, and then recovered by evaporating the water and drying at 60 °C under vacuum. After one cycle, the amount of the substrate and water was as much as the first reaction.
Results and discussion
Various organic bases as catalysts
Various organic bases were used to catalyze the reaction of CO2 and 2-aminobenzonitrile under mild conditions, and the results are listed in Table 1. When diethylamine (DA) and triethanolamine (TEA) were used, similar yields of 26% were obtained for quinazoline-2,4(1H,3H)-dione (2a) (Table 1, entries 1–2). The yield of 2a improved to 39% and 70% respectively, when 1,1,3,3-tetramethylguanidine and (TMG) 1,8-diazabicyclo[5.4.0]undec-7-ene (DBU) were used (Table 1, entries 3–4). This is in accordance with previous reports where the catalytic performance was positively related with the alkalinity of catalysts and the strongest base usually gave the most active catalytic performance.15 Excellent yields of 2a were observed on catalysts 1,4-diazabicyclo[2.2.2]octane (DABCO) and DEA. The high activity of strong base DABCO could be easily ascribed to its tridimensional rigid structure, which made it easier to desorb from intermediates.30 Herein, DEA as a catalyst should be highlighted. Although the simple linear DEA with secondary amine and hydroxyl groups possessed weaker alkalinity compared to some of the bases mentioned above, it exhibited equivalent performance as the DABCO (Table 1, entries 5–6). It should be pointed out that although both DA possessing only the secondary amine group, and TEA possessing solo hydroxyl groups, exhibited the same linear structure, they provided much lower yields than DEA (Table 1, entries 1–2). This indicated that –NH and –OH might have a cooperative effect on the incorporation of reactants, leading to a great improvement in the catalytic performance.
Table 1 Reaction of 2-aminobenzonitrile with CO2 catalyzed by various organic basesa
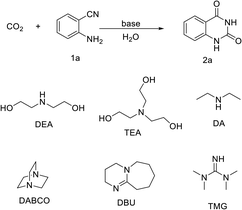
|
Entry |
Catalyst |
Yieldb (%) |
Reaction conditions: 2-aminobenzonitrile 5 mmol, catalyst amounts: 1 mmol, solvent: water 3 mL, CO2 1 MPa, 12 h, 100 °C. Isolated yield. |
1 |
DA |
26 |
2 |
TEA |
26 |
3 |
TMG |
39 |
4 |
DBU |
70 |
5 |
DABCO |
93 |
6 |
DEA |
88 |
Screening of optimum conditions
The influence of the DEA amount, temperature, CO2 pressure and reaction time on the yield of the product was investigated, and the results are summarized in Table 2. The isolated yield of 2a increased from 88% to 94% with the amount of catalyst increasing from 1 mmol to 3 mmol (entries 1–3). Further increase in the catalyst amount made a negligible change on the product yield (entry 4). When the reaction temperature was raised from 80 °C to 100 °C, the yield of quinazoline-2,4(1H,3H)-dione increased significantly from 40% to 94% (entries 3, 5). Further increase in the reaction temperature led to a slight decrease in the yield (entries 6 and 7). High temperatures could speed up the reaction of CO2 with 2-aminobenzonitrile. However, simultaneously, the CO2 solubility and DEA reactivity decreased and water at temperatures higher than its boiling point was mostly vaporized, leading to decrease in the product yield at high temperatures. The CO2 pressure had a positive influence on the yield. When the CO2 pressure increased from 0.2 MPa to 1 MPa, the yield of quinazoline-2,4(1H,3H)-dione grew dramatically from 45% to 94% (entries 1, 8 and 9). The increase in the yield slowed down with further increase in the CO2 pressure (entries 10–11). Under the optimized temperature and CO2 pressure, the influence of the reaction time on the product yield was investigated. The isolated yield of the product increased gradually with the reaction time from 6 h to 12 h. No notable yield fluctuation with further extension of the reaction time was detected (entries 12–15). In all, the optimal condition was 3 mmol catalysts (for 5 mmol 2-aminobenzonitrile), 100 °C, 1 MPa and 12 h, which is milder and more efficient than that of the previously reported base catalysts.9
Table 2 Screening of optimum conditions for the reaction of 2-aminobenzonitrile and CO2a
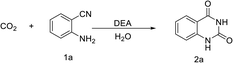
|
Entry |
Catalyst amounts (mmol) |
T (°C) |
P (MPa) |
t (h) |
Yieldb (%) |
Reaction conditions: 2-aminobenzonitrile 5 mmol, catalyst: DEA, solvent: water 3 mL. Isolated yield. |
1 |
1 |
100 |
1 |
12 |
88 |
2 |
2 |
100 |
1 |
12 |
90 |
3 |
3 |
100 |
1 |
12 |
94 |
4 |
4 |
100 |
1 |
12 |
95 |
5 |
3 |
80 |
1 |
12 |
40 |
6 |
3 |
120 |
1 |
12 |
89 |
7 |
3 |
140 |
1 |
12 |
90 |
8 |
3 |
100 |
0.2 |
12 |
45 |
9 |
3 |
100 |
0.5 |
12 |
86 |
10 |
3 |
100 |
1.5 |
12 |
92 |
11 |
3 |
100 |
2 |
12 |
94 |
12 |
3 |
100 |
1 |
6 |
58 |
13 |
3 |
100 |
1 |
9 |
89 |
14 |
3 |
100 |
1 |
15 |
94 |
15 |
3 |
100 |
1 |
18 |
95 |
Effect of solvents
The solvent effect was also investigated under optimized conditions (Table 3). 2a was obtained with a 94% yield using 3 mL water as the solvent under 1 MPa CO2 pressure at 100 °C (entry 1). The reaction in organic solvents of acetonitrile, toluene and N,N-dimethylformamide afforded quinazoline-2,4(1H,3H)-dione in 27%, 57% and 45% yield, respectively (entries 2–4), suggesting that neither polar nor non-polar organic solvents can perform at par with water. When ethanol was used as the solvent, the lowest yield of 10% was obtained, which might have resulted from the low solubility of CO2 in ethanol (entry 5). The cyclization efficiency was also highly dependent on the amount of solvent. It gave an isolated 2a yield of 26% under a solvent-free condition (entry 6). When 1 mL and 3 mL of water was used, high yields of 92% and 94% were observed. Further, on increasing the amount of water to 5 mL and 7 mL, the isolated yield decreased to 88% and 62%, respectively. This might have resulted from the dilution of the catalyst and reactants (entries 8–9). All the above results demonstrate that this process depends strongly on the presence of water and it proceeds efficiently with a relatively small quantity of water. This might be ascribed to the fact that water participated in the cyclization of CO2 and 2-aminobenzonitrile. Furthermore, water was a good solvent for CO2 and 2-aminobenzonitrile but not for quinazoline-2,4(1H,3H)-dione, which was beneficial for the purification of the product. It is worth mentioning that DEA as a catalyst for the CO2-participated cyclization avoided the need of organic solvents and the reaction conditions were mild. This process is greener, more efficient and environment-friendly than that reported previously (Table S1 in ESI†).
Table 3 The influence of solventsa
Entry |
Solvent |
Solvent amount (mL) |
Yieldb (%) |
Reaction conditions: 2-aminobenzonitrile 5 mmol, catalyst amounts: 3 mmol, CO2 1 MPa, 12 h, 100 °C. Isolated yield. |
1 |
H2O |
3 |
94 |
2 |
CH3CN |
3 |
27 |
3 |
PhCH3 |
3 |
57 |
4 |
DMF |
3 |
45 |
5 |
CH3CH2OH |
3 |
10 |
6 |
Solvent free |
0 |
26 |
7 |
H2O |
1 |
92 |
8 |
H2O |
5 |
88 |
9 |
H2O |
7 |
62 |
Reaction of CO2 with different substrates
To demonstrate the generality of DEA as a catalyst in the synthesis of quinazoline-2,4(1H,3H)-diones, various 2-aminobenzonitrile substrates with electron-withdrawing or electron-donating substituents were studied using DEA as the catalyst under optimized reaction conditions (Table 4). All reactions proceeded smoothly with different substrates, producing the corresponding quinazoline-2,4(1H,3H)-diones in excellent isolated yields. The yields of products when 2-amino-5-fluorobenzonitrile, 2-amino-5-chlorobenzonitrile and 2-amino-5-bromobenzonitrile were used as substrates were 94%, 93% and 88%, respectively (entries 1–3). The results showed that the halogen substituents of 2-aminobenzonitriles had little effect on the reaction. 4-Methyl-2-aminobenzonitrile provided a 70% yield of 7-methyl-quinazoline-2,4(1H,3H)-dione, which was probably due to the steric effect (entry 5). Owing to both the electron-withdrawing effect and the steric effect, the yield of 7-chloro-quinazoline-2,4(1H,3H)-dione from 2-amino-4-chlorobenzonitrile was only 62% even after prolonging the reaction time to 24 h (entry 4). The presence of electron-donating groups has a slight positive influence on the reaction of the substrate with CO2, yield of the product from 2-amino-4,5-dimethoxybenzonitrile was 93% (entry 6).
Table 4 Synthesis of various quinazoline-2,4(1H,3H)-dionesa
Entry |
Substrate |
Product |
Yieldb (%) |
Reaction conditions: substrate 5 mmol, catalyst: DEA 3 mmol, solvent: water 3 mL, CO2 1 MPa, 12 h, 100 °C. Isolated yield. Reaction time: 24 h. |
1 |
 |
 |
94 |
2 |
 |
 |
93 |
3 |
 |
 |
88 |
4 |
 |
 |
62c |
5 |
 |
 |
70 |
6 |
 |
 |
93 |
Catalyst recyclability
It should be highlighted that the DEA catalyst could be easily recovered from the mixture after the reaction by filtration, washing with water and concentration because DEA is easily soluble in water, while quinazoline-2,4(1H,3H)-diones are insoluble. The recovered DEA was further used to test the reusability of catalysts. As shown in Fig. 1, similar high yield products were obtained in five reaction cycles under optimized reaction conditions, which demonstrated excellent reusability of the catalyst. The simple post-process and reuse without any pollution made it a green process suitable for mass production.
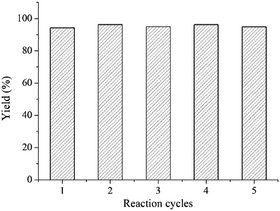 |
| Fig. 1 Recycling of DEA in the reaction of 2-aminobenzonitrile and CO2. | |
A plausible reaction mechanism
Density functional theory (DFT) is an effective and powerful tool to investigate the possible mechanism of typical reactions.38,39 In this study, the interaction between reactants and catalysts was investigated via the DFT method. The reaction coordinate calculations of CO2 and 2-aminobenzonitrile catalyzed by DEA in H2O were carried out using the M06-2X functional combined with the 6-31+G* basis set in the Gaussian 09 package, as shown in Fig. 2. The three curves denoted different pathways from the substrate to the carbamic acid intermediate as mentioned in the proposed mechanism. The blue curve describes that the activated 2-aminobenzonitrile combines with CO2 to form a four-member ring transition state, and the calculated energy of TS was 41.9 kcal mol−1. Six-member ring transition states were obtained by adding a molecule of H2O or DEA in the other two pathways. Lower corresponding energy barriers of 19.9 and 18.3 kcal mol−1 could be observed. Furthermore, for the intermediates produced, the hydrous one stays at a lower energy level than that containing the dual states of DEA. The calculated result indicated that H2O not only served as a solvent but also as the catalytic species reducing activation energy, and the hydroxyl groups on DEA is indispensable for the substrate activation and intermediate formation in the plausible reaction mechanism.
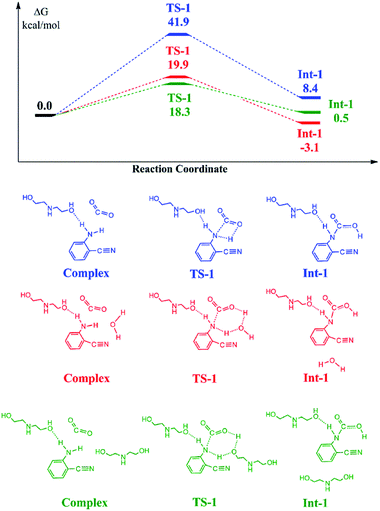 |
| Fig. 2 The free energy ΔG profiles and crucial transition states and intermediates of different pathways for the activation of CO2 and 2-aminobenzonitrile. | |
The interactions in the mixed system were further examined using 1H NMR and FT-IR techniques. Fig. 3 shows the 1H NMR spectrum of pure 2-aminobenzonitrile and the mixture of 2-aminobenzonitrile and DEA. The signal at 6.04 ppm, which is attributed to the hydrogen atom in the amine group of 2-aminobenzonitrile, shifted to 5.92 ppm in the mixture, suggesting that N–H bonds of 2-aminobenzonitrile could be activated by forming hydrogen bonds with hydramine in the presence of DEA for the following CO2 fixation. This is similar to that reported by Zhao et al. where an alkalogenic anion activates 2-aminobenzonitrile as well as CO2 in the initial activation stage.20 Additionally, the FT-IR analysis was carried out for the mixture before and after the addition of CO2 (Fig. S1 in ESI†). After continuous stirring in the CO2 atmosphere, new bands at 1265, 1336 and 1568 cm−1 could be observed, assigned to the stretching vibration of the C
O bond of CO2. Meanwhile, signal peaks that appeared at 1639 cm−1 and 3230 cm−1 could be attributed to the C–N bond and N–H bond, respectively. This confirmed the formation of carbamate from the substrate (Scheme 2).
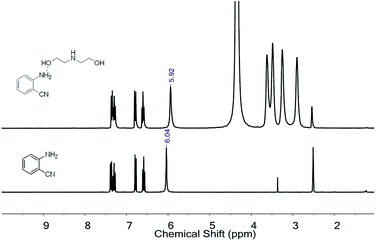 |
| Fig. 3 1H NMR spectrum of 2-aminobenzonitrile, with and without DEA (DMSO-d6, 298 K). | |
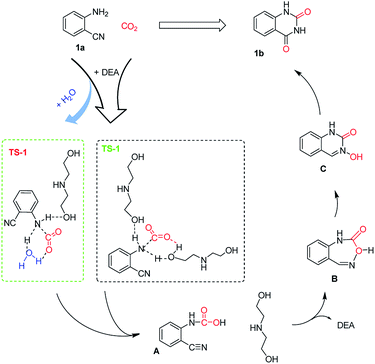 |
| Scheme 2 Plausible mechanism for the DEA-catalyzed cyclization of 2-aminobenzonitrile and CO2 in water. | |
Conclusions
In summary, the cost-effective commercial DEA was used as the catalyst under mild conditions to promote the chemical combination of CO2 and 2-aminobenzonitrile. The amine and hydroxyl groups on DEA, which activated the 2-aminobenzonitrile and CO2 simultaneously, are crucial for the activation of reactants. Water was used as the solvent and co-catalyst. During the formation of transition state, water participated in the formation of a novel the six-member ring and promoted the reaction through a suitable pathway with a lower energy barrier. It should be noted that all reagents used in this process are commercial and environment-friendly. The catalyst can be easily recovered and reused for at least five cycles. This protocol is green and cost-effective, and possesses considerable potential applications in CO2 utilization and green synthesis chemistry.
Conflicts of interest
There are no conflicts to declare.
Acknowledgements
This work is supported by the National Natural Science Foundation of China (21573073), the National Key Research and Development Program of China (2017YFA0403102) and Shanghai Leading Academic Discipline Project (B409).
Notes and references
- J. Rogelj, M. den Elzen, N. Höhne, T. Fransen, H. Fekete, H. Winkler, R. Schaeffer, F. Sha, K. Riahi and M. Meinshausen, Nature, 2016, 534, 631–639 CrossRef CAS.
- M. He, Y. Sun and B. Han, Angew. Chem., Int. Ed., 2013, 52, 9620–9633 CrossRef CAS.
- M. Aresta, A. Dibenedetto and A. Angelini, Chem. Rev., 2014, 114, 1709–1742 CrossRef CAS.
- A. Otto, T. Grube, S. Schiebahn and D. Stolten, Energy Environ. Sci., 2015, 8, 3283–3297 RSC.
- T. Sakakura, J.-C. Choi and H. Yasuda, Chem. Rev., 2007, 107, 2365–2387 CrossRef CAS.
- D. Wang and F. Gao, Chem. Cent. J., 2013, 7, 95 CrossRef.
- O. O. Ajani, D. V. Aderohunmu, E. N. Umeokoro and A. O. Olomieja, Bangladesh J. Pharmacol., 2016, 11, 716–733 CrossRef.
- M. Michman, S. Patai and Y. Wiesel, Org. Prep. Proced. Int., 1978, 10, 13–16 CrossRef CAS.
- H. Vorbruggen and K. Krolikiewicz, Tetrahedron, 1994, 50, 6549–6558 CrossRef CAS.
- F. Nikpour and T. Paibast, Chem. Lett., 2005, 34, 1438–1439 CrossRef CAS.
- T. Mizuno, N. Okamoto, T. Ito and T. Miyata, Tetrahedron Lett., 2000, 41, 1051–1053 CrossRef CAS.
- T. Mizuno and Y. Ishino, Tetrahedron, 2002, 58, 3155–3158 CrossRef CAS.
- T. Mizuno, T. Iwai and Y. Ishino, Tetrahedron Lett., 2004, 45, 7073–7075 CrossRef CAS.
- D. Nagai and T. Endo, J. Polym. Sci., Part A: Polym. Chem., 2009, 47, 653–657 CrossRef CAS.
- J. Gao, L.-N. He, C.-X. Miao and S. Chanfreau, Tetrahedron, 2010, 66, 4063–4067 CrossRef CAS.
- W. Lu, J. Ma, J. Hu, Z. Zhang, C. Wu and B. Han, RSC Adv., 2014, 4, 50993–50997 RSC.
- T. Kimura, H. Sunaba, K. Kamata and N. Mizuno, Inorg. Chem., 2012, 51, 13001–13008 CrossRef CAS.
- Y. P. Patil, P. J. Tambade, K. M. Deshmukh and B. M. Bhanage, Catal. Today, 2009, 148, 355–360 CrossRef CAS.
- W. Lu, J. Ma, J. Hu, J. Song, Z. Zhang, G. Yang and B. Han, Green Chem., 2014, 16, 221–225 RSC.
- Y. Zhao, B. Yu, Z. Yang, H. Zhang, L. Hao, X. Gao and Z. Liu, Angew. Chem., Int. Ed., 2014, 53, 5922–5925 CrossRef CAS.
- H. Zheng, X. Cao, K. Du, J. Xu and P. Zhang, Green Chem., 2014, 16, 3142–3148 RSC.
- X.-D. Lang, S. Zhang, Q.-W. Song and L.-N. He, RSC Adv., 2015, 5, 15668–15673 RSC.
- V. B. Saptal and B. M. Bhanage, ChemCatChem, 2016, 8, 244–250 CrossRef CAS.
- X.-D. Lang, Y.-C. Yu, Z.-M. Li and L.-N. He, J. CO2 Util., 2016, 15, 115–122 CrossRef CAS.
- Y. Xiao, X. Kong, Z. Xu, C. Cao, G. Pang and Y. Shi, RSC Adv., 2015, 5, 5032–5037 RSC.
- Q. Wang, C. Lu, B. Zhao and Y. Yao, Eur. J. Org. Chem., 2016, 2555–2559 CrossRef CAS.
- S.-i. Fujita, M. Tanaka and M. Arai, Catal. Sci. Technol., 2014, 4, 1563–1569 RSC.
- Y.-N. Zhao, B. Yu, Z.-Z. Yang and L.-N. He, RSC Adv., 2014, 4, 28941–28946 RSC.
- S. M. Sadeghzadeh, Catal. Sci. Technol., 2016, 6, 1435–1441 RSC.
- B. Sarmah and R. Srivastava, Ind. Eng. Chem. Res., 2017, 56, 8202–8215 CrossRef CAS.
- J. Ma, B. Han, J. Song, J. Hu, W. Lu, D. Yang, Z. Zhang, T. Jiang and M. Hou, Green Chem., 2013, 15, 1485–1489 RSC.
- T. Damartzis, A. I. Papadopoulos and P. Seferlis, J. Cleaner Prod., 2016, 111, 204–216 CrossRef CAS.
- M. Mannisto, P. Uusi-Kyyny, D. Richon and V. Alopaeus, J. Chem. Eng. Data, 2017, 62, 2261–2271 CrossRef CAS.
- A. Aroonwilas and A. Veawab, Ind. Eng. Chem. Res., 2004, 43, 2228–2237 CrossRef CAS.
- A. Garcia-Abuin, D. Gomez-Diaz, A. B. Lopez, J. M. Navaza and A. Rumbo, Ind. Eng. Chem. Res., 2013, 52, 13432–13438 CrossRef CAS.
- H. Gao, B. Xu, H. Liu and Z. Liang, Energy Fuels, 2016, 30, 7481–7488 CrossRef CAS.
- F. Barzagli, F. Mani and M. Peruzzini, Environ. Sci. Technol., 2016, 50, 7239–7246 CrossRef CAS.
- W. Li, N. Yang and Y. Lyu, Org. Chem. Front., 2016, 3, 823–835 RSC.
- J. Ma, J. Hu, W. Lu, Z. Zhang and B. Han, Phys. Chem. Chem. Phys., 2013, 15, 17333–17341 RSC.
Footnote |
† Electronic supplementary information (ESI) available. See DOI: 10.1039/d0ra06439d |
|
This journal is © The Royal Society of Chemistry 2020 |
Click here to see how this site uses Cookies. View our privacy policy here.