DOI:
10.1039/D0RA05548D
(Paper)
RSC Adv., 2020,
10, 33196-33204
Preparation and characterization of squid pen chitooligosaccharide–epigallocatechin gallate conjugates and their antioxidant and antimicrobial activities
Received
25th June 2020
, Accepted 28th August 2020
First published on 8th September 2020
Abstract
Chitooligosaccharide (COS) and epigallocatechin-3-gallate (EGCG) at various concentrations were used for the preparation of COS–EGCG conjugates. The highest total phenolic content (TPC), representing the amount of EGCG conjugated, was obtained for 1 wt% COS together with EGCG at 0.5 wt% (C1-E0.5-conjugate) or 1.0 wt% (C1-E1.0-conjugate) (66.83 and 69.22 mg EGCG per g sample, respectively) (p < 0.05). The 2,2-diphenyl-1-picryl-hydrazyl-hydrate (DPPH) and 2,2′-azino-bis(3-ethylbenzothiazoline-6-sulfonic acid) (ABTS) radical scavenging activities (DRSA and ARSA, respectively) and ferric reducing antioxidant power (FRAP) of all the samples showed similar trends with TPC. The C1-E0.5-conjugate had higher DRSA, ARSA, FRAP and oxygen radical absorbance capacity (ORAC) values than COS (p < 0.05). Similarly, the antimicrobial activity of COS increased when conjugated with EGCG (p < 0.05). FTIR, 1H-NMR and 13C-NMR analyses confirmed the successful grafting of EGCG with COS. Therefore, 1 wt% COS and 0.5 wt% EGCG were used for the production of a conjugate with augmented antioxidant activity, which could be used to retard lipid oxidation of fatty foods.
1. Introduction
Squid (Loligo formosana) pen, which is generally discarded from the squid processing industry, is an excellent source of β-chitin.1,2 β-Chitin generally shows higher reactivity towards various solvents than α-chitin due to its looser structure. Therefore it can be easily deacetylated to chitosan, followed by hydrolysis using non-specific enzymes to produce chitooligosaccharide (COS).2,3 COS has been used in medicinal and food industries because of its non-toxicity, numerous bioactivities and higher water solubility.4 COS is known to possess profound antibacterial as well as antioxidant properties.3 Chitosan and COS have been further modified to enhance their bioactivities such as antioxidant, antimicrobial, antihypersensitive, anticancer activities, etc. Various methods such as carboxylation, methylation, sulfation, phosphorylation, etc. have been employed.3,5 Moreover, COS grafted with phenolic compounds (PCs) has been implemented for medicinal purposes. PCs are known to donate H-atom, which could enhance antioxidant activity of conjugated COS.3,5 Ngo et al. reported that gallic acid successfully enhanced various bioactivities when conjugated with commercial COS using a coupling reaction.6
Epigallocatechin gallate (EGCG) is a natural phenolic antioxidant, which is water soluble. Nevertheless, its lower solubility in lipo-philic system limits its effectiveness in fats, oils, lipid-based food or food products.7 Therefore, EGCG has been incorporated or grafted with various biological molecules such as proteins, polysaccharides, chitosan, etc. to enhance its uptake and affinity to lipid molecules or membrane as well as foods prone to lipid oxidation.7,8 Several bioactivities of conjugated compounds have been documented to augment. The chitosan–EGCG conjugate showed higher inhibition against Staphylococcus and Pseudomonas than EGCG or chitosan alone.9 Additionally, conjugation also increased antioxidant activity of chitosan.9 Lei et al. observed the improved emulsifying properties of chitosan after being conjugated with EGCG.10 However, solubility of chitosan in acidic condition limits its applications in foods. Conversely, COS is water soluble in nature, which can be implemented in various foods without affecting their quality and consumer acceptability. Moreover, COS possessed higher antioxidant and antimicrobial activity than the chitosan due to smaller size and higher availability of reactive groups such as amino and hydroxyl groups.3 Eom et al. observed an increase in β-secretase inhibitory activity when eight kinds of COS conjugates were produced with hydroxyl cinnamic and hydroxyl benzoic acids.11 EGCG, especially from green tea, possessed higher antioxidant activity than catechin, epigallocatechin, epicatechin gallate, etc.12 It could be used for conjugation with COS.
Numerous methods such as enzymatic, carbamide, redox reactions, etc. have been employed for grafting of chitosan or COS with PCs.13 Due to lower toxicity and low cost, ascorbic acid (AcA) in conjugation with H2O2 have been used widely.13 Moreover, the reaction can be conducted at room temperature, which could retard the degradation and oxidation of various PCs. Nevertheless, no information has been available for EGCG conjugated with COS, especially from squid pen chitosan, which is beta-form. Due to high antioxidant and antimicrobial activity of COS-plant polyphenol conjugates,3 squid pen COS–EGCG can be used as the novel or alternative additives, especially in fatty foods, to prevent lipid oxidation as well as to retard the microbial spoilage of foods.
Therefore, the aim of the study was to optimize the conditions for COS–EGCG conjugation via a free radical grafting method. Additionally, antioxidant and antibacterial activities of the selected COS–EGCG conjugate against Escherichia coli (Gram-negative) and Listeria monocytogenes (Gram-positive) bacteria were evaluated.
2. Materials and methods
2.1. Chemicals and bacterial strains
All chemicals were of analytical grade and procured from Sigma-Aldrich, Inc. (St. Louis, MO, USA). All microbial media were obtained from Oxoid (Thermo Fisher, Hampshire, United Kingdom). Ascorbic acid (AcA) and H2O2 (35 vol%) were obtained from Loba Chemi (Mumbai, Maharashtra, India). EGCG was acquired from Chengdu Biopurify Phytochemicals Ltd. (Sichuan, China).
Bacterial strains including L. monocytogenes F2365, and E. coli DMST 4212, were obtained from Food Safety Laboratory, Cornell University, Ithaca, NY, USA.
2.2. Preparation of chitooligosaccharides (COS)
One gram of chitosan extracted from squid (Loligo formosana) pen (degree of deacetylation: 87%) was firstly dissolved in 100 mL of 1 vol% acetic acid. Thereafter, the pH was adjusted to 5.0 using 6 M NaOH. The mixture was added with 8 wt% lipase and hydrolysis was conducted for 12 h at 50 °C as per the method of Singh et al.14 The prepared COS had the viscosity-average molecular weight (MW) of 76 kDa, intrinsic viscosity of 0.39 dL g−1 and water solubility of 50%.14
2.3. Preparation of COS–EGCG conjugate
The COS–EGCG conjugates were synthesized by a free radical grafting method with minor modifications.15 In this method, AcA and H2O2 were used as a redox pair of initiator system (Fig. 1). In this reaction system, amino or hydroxyl groups of glucosamine unit of COS were oxidized by hydroxyl radicals (˙OH) generated by the reaction of redox pair and resulted in the formation of macroradical.13 The radicals localized on COS then reacted with EGCG via covalent bond and resulted in the formation of COS–EGCG conjugate. Firstly, COS at different concentrations (1 and 2 wt%) was oxidized using 0.2 wt% AcA and 0.1 M H2O2 for 2 h at 25 °C under dark condition. Then, solutions were added with 0.1, 0.5 and 1.0 wt% EGCG and the mixtures were stirred for 24 h in dark at 25 °C. The obtained mixtures were dialyzed against distilled water at 25 °C for 48 h using a dialysis bag (MW cut-off: 1000 Da). The dialysis buffer was changed until no free EGCG was detected. The free and bound EGCG was determined by measuring total phenolic content (TPC) using the Folin–Ciocalteu reagent.16 The TPC was expressed as mg EGCG equivalent per g sample, which represented the amount of EGCG incorporated into COS. The dialysate mixtures or conjugates were subjected to determination of antioxidant activities.
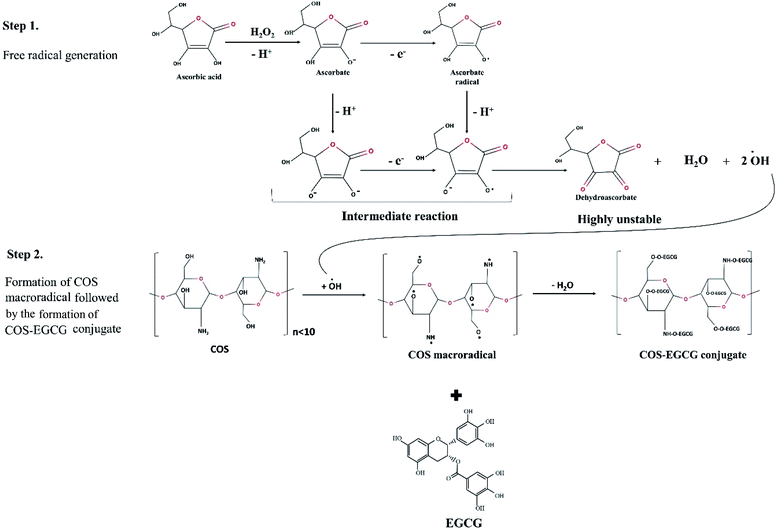 |
| Fig. 1 Proposed mechanism for synthesis of chitooligosaccharide (COS) and epigallocatechin gallate (EGCG) conjugate via ascorbic acid/H2O2 redox pair mediated free radical grafting method. | |
2.3.1. Antioxidant activities. DPPH radical scavenging activity (DRSA) and ABTS radical scavenging activity (ARSA) and ferric reducing antioxidant power (FRAP) were determined by the methods given by Chotphruethipong et al.16 In brief, for DRSA, 100 μL of sample was mixed with 900 μL of 0.06 mM DPPH (dissolved in methanol) and mixed vigorously followed by incubation at 25 °C in the dark for 1 h. Then the optical density (OD) of mixture was measured at 515 nm using a microplate reader (Thermo Scientific Varioskan® Flash Multimode Reader, Fisher Scientific UK Ltd., Leicestershire, UK). The distilled water was used for blank preparation instead of sample. A standard curve was prepared using Trolox in the range of 10–60 μM.For ARSA, ABTS radical was produced by reacting 7.4 mM ABTS stock solution with 2.6 mM potassium persulphate at a ratio of 1
:
1 (v/v) for 12 h at room temperature. Thereafter, radical solution was diluted with methanol to obtain an absorbance of 1.1 (±0.02) at 734 nm. To initiate the reaction, 50 μL of sample was mixed with 950 μL of radical solution and incubated at room temperature for 2 h in dark and OD was then read at 734 nm using microplate reader. Trolox standard curve (0–600 μM) was prepared. Distilled water was used instead of the sample and prepared in the same manner to obtain the blank.
FRAP assay was carried out as described by Sae-Laew et al.17 Briefly, 2 mL of working FRAP reagent (0.01 M 2,4,6-tri(2-pyridyl)-s-triazine (TPTZ) in 0.04 M HCl, 0.02 M FeCl3·6H2O and 0.3 M acetate buffer), freshly prepared, was mixed with 1 mL of sample and incubated for 45 min in the dark. The OD was measured at 593 nm. A standard curve was prepared using Trolox in the range of 20–100 μM. DRSA, ARSA and FRAP activities were reported as mmol Trolox equivalents (TE) per g sample.
The conjugate prepared using 1 wt% COS and 0.5 wt% EGCG showing the highest antioxidant activity was lyophilized using a freeze-dryer (Scanvac Model Coolsafe 55, Coolsafe, Lynge, Denmark) and the resulting powder named “C1-E0.5-conjugate” was stored in a ziplock bag and subjected to further characterization in comparison with “COS”. The yield and conjugation efficiency of C1-E0.5-conjugate were 17.1 and 75.84%, respectively, as determined following the method of Lei et al.10
2.4. Characterization of the selected C1-E0.5-conjugate in comparison with COS
2.4.1. Antioxidant activities. DRSA, ARSA, and FRAP were determined as described previously. Oxygen radical absorbance capacity (ORAC) was performed as described by Sae-Leaw et al.17 The samples were dissolved in 75 mM phosphate buffer (pH 7.0) to obtain a final concentration of 0.1 mg mL−1. The prepared sample (25 μL) was loaded onto a black polystyrene, nontreated 96-well microplate (Costar Corning Inc., Corning, NY, USA). Only the internal wells of the microplate were used. Fifty μL of 0.04 μM fluorescein dissolved in 75 mM phosphate buffer (pH 7.0) were added to each sample. The loaded microplate was allowed to equilibrate at 37 °C for 20 min in a microplate reader. The reaction was initiated by the addition of 100 μL of 221 mM AAPH. The reaction was performed at 37 °C. The fluorescence intensity was measured every 5 min for 90 min with excitation and emission filters of 485 and 535 nm, respectively. The control was prepared in the same manner, except that 75 mM phosphate buffer (pH 7.0) was used instead of the sample. The area under the fluorescence decay curve (AUC) of the samples was calculated by the normalized curves with the following equation:
AUC = AUC = 0.5 + (f2/f1) + (f3/f1) + (f4/f1) + ⋯ + 0.5(fn/f1) |
where f1 is the fluorescence reading at the initiation of the reaction and fn is the last measurement. The net AUC was obtained by subtracting the AUC of the blank from that of a sample or standard. Trolox (0–100 μM) was used as the standard. The ORAC was expressed as μmol Trolox equivalents (TE) per g sample.The metal chelating activity (MCA) was also determined using the method of Singh et al.14 Briefly, 1 mL of sample solution was mixed with 3.7 mL of distilled water. The mixture was then reacted with 0.1 mL of 2 mM FeCl2 and 0.2 mL of 5 mM 3-(2-pyridyl)-5,6-bis(4-phenyl-sulfonic acid)-1,2,4-triazine (ferrozine) for 20 min at room temperature. The OD was read at 562 nm. The control was prepared in the same manner except that distilled water was used instead of the sample. MCA was reported as mmol EDTA equivalent (EE) per g sample.
2.4.2. Antibacterial activity. Antibacterial activity of COS and C1-E0.5-conjugate was determined by calculating minimum inhibitory concentration (MIC) and minimum bactericidal concentration (MBC) against L. monocytogenes and E. coli as per the method of Singh and Benjakul.18
2.4.3. Fourier transform infrared (FTIR) analysis. FTIR spectra of COS, EGCG and C1-E0.5-conjugate were elucidated using an attenuated total reflection (ATR)-Fourier transform infrared (FTIR) spectroscopy with a Bruker Model Equinox 55 FTIR spectrometer (Bruker Co., Ettlingen, Germany) by the method described by Singh et al.2 The absorption of IR in the region of 400–4000 cm−1 was determined using 32 scans with a resolution of 4 cm−1. These spectra were subtracted from the reference spectrum of air.
2.4.4. Proton NMR (1H NMR) and carbon NMR (13C NMR) spectral analyses. 1H NMR and 13C NMR spectra of COS and C1-E0.5-conjugate were recorded in D2O and chemical shifts were expressed as parts per million (ppm) following the method of Huang et al.19
2.5. Statistical analysis
All data were measured in triplicate and applied to the analysis of variance. Duncan's multiple range test from SPSS package (SPSS 22 for Windows, SPSS Inc., Chicago, IL, USA) was used for comparison of means.
3. Results and discussion
3.1. Effect of COS and EGCG at different concentrations on total phenolic content (TPC) and antioxidant activities of COS–EGCG conjugates
3.1.1. Total phenolic content (TPC). TPC of conjugates derived from 1 and 2 wt% COS at various EGCG concentrations was in the range of 15.59–69.22 and 20.16–63.88 mg EGCG per g sample, respectively (Table 1). Irrespective of COS concentrations, TPC of conjugates was increased with increasing EGCG concentrations from 0.1 to 1.0 wt% (p < 0.05). At higher EGCG concentrations, EGCG showed higher binding efficiency towards oxidized COS. Generally, ˙OH radical formed via AcA/H2O2 redox pair reaction could retract an H-atom from COS and resulted in the formation of COS macro radical.15 EGCG acted as an acceptor of COS macro radical, in which COS–EGCG conjugate could be formed (Fig. 1).15 It was noted that when COS at 2 wt% was used, the lower TPC was obtained, regardless of EGCG concentrations (p < 0.05). This was more likely associated with increased viscosity of COS solution, which might impede the interaction between oxidized COS and EGCG. Additionally, EGCG ratios in reaction system were also lower as COS concentration increased. Similarly, Eom et al. reported a decrease in TPC as a higher amount of COS was used for conjugation process with different PCs.20 The lower TPC was obtained for the samples containing 2 wt% COS, except C2-E0.1-conjugate, which had higher TPC as compared to the C1-E0.1-conjugate (p < 0.05). This was more likely associated with the increasing number of amino and hydroxyl groups of COS at higher concentration (2%) for conjugation with EGCG as compared to 1 wt% COS. The C1-E0.5- and C1-E1.0-conjugates had higher TPC (66.83 and 69.22 mg EGCG per g sample, respectively) as compared to other conjugates (p < 0.05). Nevertheless, no difference in TPC was detected between both samples (p > 0.05). In the present study, TPC was used to determine the amount of EGCG bound to COS. Similarly, Lei et al. also determined the amount of EGCG bound to commercial chitosan of varying MW using the FCR method.10 Therefore, concentrations of both COS and EGCG affected the formation of conjugates, as indicated by amount of EGCG incorporated into COS at various levels.
Table 1 Total phenolic content (TPC) and antioxidative activities of conjugates prepared using chitooligosaccharide (COS) and EGCG at different concentrationsa
COS–EGCG conjugates |
TPC (mg EGCG equivalent per g sample) |
DRSA (mmol TE per g sample) |
ARSA (mmol TE per g sample) |
FRAP (mmol TE per g sample) |
Values are presented as mean ± SD (n = 3). Different lowercase letters within the same COS concentration in the same column indicate a significant difference (p < 0.05). Different uppercase letters in the same column indicate a significant difference (p < 0.05). C1-E0.1: conjugates prepared using 1 wt% COS and 0.1 wt% EGCG, C1-E0.5: conjugates prepared using 1 wt% COS and 0.5 wt% EGCG and C1-E1.0: conjugates prepared using 1 wt% COS and 1 wt% EGCG. C2-E0.1: conjugates prepared using 2 wt% COS and 0.1 wt% EGCG, C2-E0.5: conjugates prepared using 2 wt% COS and 0.5 wt% EGCG, C2-E1.0: conjugates prepared using 2 wt% COS and 1 wt% EGCG. |
C1-E0.1 |
15.59 ± 0.73bF |
41.63 ± 2.89bB |
38.10 ± 1.85bD |
54.18 ± 3.6cF |
C1-E0.5 |
66.83 ± 5.52aB |
49.21 ± 3.53aA |
145.54 ± 4.99aA |
419.78 ± 4.69aB |
C1-E1.0 |
69.22 ± 4.28aA |
51.69 ± 3.19aA |
147.42 ± 5.00aA |
422.69 ± 3.14aA |
C2-E0.1 |
20.16 ± 1.57cE |
31.08 ± 1.09cD |
24.06 ± 1.29cE |
65.81 ± 7.92cE |
C2-E0.5 |
46.10 ± 2.18bD |
36.02 ± 3.03bC |
77.16 ± 4.69bC |
190.15 ± 8.94bD |
C2-E1.0 |
63.88 ± 2.95aC |
40.28 ± 2.88aB |
121.72 ± 14.24aB |
380.80 ± 22.29aC |
3.1.2. Antioxidant activities. Antioxidant activities of all conjugates synthesized under various conditions are shown in Table 1. In general, DRSA measured the ability of compounds to act as hydrogen donors or free radical scavengers.14 The conjugate prepared from 1 wt% COS had higher DRSA (41.63–51.69 mmol TE per g sample) as compared to those derived from 2 wt% COS (31.08–40.28 mmol TE per g sample), regardless of EGCG concentrations (p < 0.05). C1-E0.5- and C1-E1.0-conjugates showed the highest DRSA (49.21 and 51.69 mmol TE per g sample, respectively) (p < 0.05), but both conjugates had similar values (p > 0.05). For conjugates prepared from 2 wt% COS, DRSA was increased with increasing EGCG concentrations and the C2-E1.0-conjugate showed the highest value (40.28 mmol TE per g sample) (p < 0.05). Similarly, ARSA was augmented with increasing concentrations of EGCG used for conjugation (p < 0.05) (Table 1). C1-E0.5- and C1-E1.0-conjugates showed the highest activity (145.54 and 147.42 mmol TE per g sample, respectively) than the remaining samples (p < 0.05). C2-E1.0-conjugate had the highest ARSA (121.72 mmol TE per g sample) compared to conjugates prepared using 2 wt% COS (p < 0.05) (Table 1). The increased DRSA and ARSA were more likely associated with an increasing number of hydroxyl groups from EGCG, which could function as hydrogen donor to radicals.14 EGCG with 8 hydroxyl groups, in which hydroxyl groups at carbon number 3, 4 and 5 and with a gallate moiety at 3′ position in C ring are considered as the good electron donor. Moreover, ability of EGCG to delocalize electrons might contribute to its antioxidant activity.21 Eom et al. also reported an increase in DRSA when COS was conjugated with different kinds of PCs.20 Similarly, ARSA and DRSA were increased when chitosan–EGCG conjugate or chitosan–gallic acid conjugate were prepared via free radical/redox pair mechanism.10,22FRAP of conjugates synthesized using COS and EGCG at different concentrations is presented in Table 1. Normally, FRAP determines the reducing power of antioxidants to reduce Fe(III) to Fe(II) present in TPTZ-complex.14 FRAP of conjugates was increased with increasing EGCG concentrations used, for both COS concentrations (p < 0.05). C1-E0.5- and C1-E1.0-conjugate showed the highest FRAP (419.78 and 422.69 mmol TE per g sample, respectively) than other conjugates (p < 0.05). However, no difference in FRAP was observed between both samples (p > 0.05). EGCG has the potential to chelate metals like cadmium, chromium, iron, and copper.23 The B-ring (Fig. 4B) is more likely responsible for the metal chelating activity of EGCG.24 Hence, the results suggested that the incorporation of EGCG at appropriate concentrations into COS could enhance the antioxidant activities of the resulting conjugates.
There was no further improvement in antioxidant activities when COS and EGCG were above 1 and 0.5 wt% (p > 0.05), respectively. Therefore, C1-E0.5-conjugate was selected for further characterization.
3.2. Characteristics of C1-E0.5-conjugate in comparison COS
3.2.1. Antioxidant activities. DRSA, ARSA, FRAP, ORAC, and MCA of COS and C1-E0.5-conjugate are depicted in Table 2. DRSA and ARSA of COS were 0.50 and 4.22 mmol TE per g sample, respectively. FRAP (6.96 mmol TE per g sample), MCA (1.54 mmol EE per g sample) and ORAC (0.18 mmol TE per g sample) were lower than those of C1-E0.5-conjugate. It was noted that all the antioxidant activities of COS were increased substantially when conjugated with EGCG. C1-E0.5-conjugate showed higher ARSA (123.03 mmol TE per g sample), and MCA (77.21 mmol EE per g sample) (p < 0.05). DRSA, FRAP, and ORAC were also increased to 41.68, 390.29 and 12.17 mmol TE per g sample, respectively. Generally, ARSA assay is implemented for both hydro-philic and lipo-philic systems, whereas DRSA assay is used for the latter. ORAC assay determined the oxidative damage of fluorescence when an azo-initiator produces the peroxyl radical by heating and causing the loss of fluorescence. Free amino group (NH2) of COS has ability to take hydrogen ion and form ammonium group (NH3+), which subsequently reacts with radicals. Additionally, hydroxyl groups present at carbon number 3 and 6 could function as hydrogen-donors to radicals.14 Conjugation of EGCG at carbon number 2 or 3 or 6 of COS increased the number of hydroxyl groups which could increase the antioxidant activities of COS. The antioxidant activity of EGCG is associated with the ability to quench free radicals, terminate the chain reactions in lipid oxidation and chelate metal ions.20
Table 2 Antioxidant and antimicrobial activities of the selected COS–EGCG conjugate in comparison with COSa
Activities |
Samples |
COS |
C1-E0.5-conjugate |
Values are presented as mean ± SD (n = 3). Different lowercase letters within the same row indicate a significant difference (p < 0.05). COS: chitooligosaccharide, C1-E0.5-conjugate: conjugate prepared using 1 wt% COS and 0.5 wt% EGCG. |
Antioxidant activities |
DRSA (mmol TE per g sample) |
0.50 ± 0.21b |
41.69 ± 5.60a |
ARSA (mmol TE per g sample) |
4.22 ± 2.64b |
123.03 ± 15.47a |
FRAP (mmol TE per g sample) |
6.96 ± 1.96b |
390.29 ± 17.98a |
ORAC (mmol TE per g sample) |
0.18 ± 0.01b |
12.17 ± 1.40a |
MCA (mmol EE per g sample) |
1.54 ± 0.16b |
77.27 ± 1.43a |
Antimicrobial activities |
Escherichia coil |
MIC (mg mL−1) |
0.05 ± 0.00a |
0.01 ± 0.00b |
MBC (mg mL−1) |
0.1 ± 0.00a |
0.05 ± 0.01b |
Listeria monocytogenes |
MIC (mg mL−1) |
2.00 ± 0.09a |
1.00 ± 0.12b |
MBC (mg mL−1) |
2.00 ± 0.10a |
1.00 ± 0.10b |
3.2.2. Antibacterial activity. MIC and MBC of COS and C1-E0.5-conjugate against targeted bacteria are presented in Table 2. For COS, MIC were 0.05 and 2 mg mL−1 for E. coli and L. monocytogenes, respectively, whereas MIC for C1-E0.5-conjugate was observed at 0.01 and 1 mg mL−1, respectively. Both COS and C1-E0.5-conjugate had higher MIC values for L. monocytogenes as compared to E. coil. L. monocytogenes, which is a Gram-positive bacterium consisting of the thicker cell wall, could be more tolerant against antimicrobial agents than Gram-negative bacteria. However, E. coil, a Gram-negative bacterium, was more susceptible to those agents. Singh et al. also observed similar results for COS from squid pen against Gram-positive bacteria.14 Generally, the antibacterial activity of COS was associated with disruption of bacterial cell wall via electrostatic interaction due to amino and hydroxyl groups.14 Moreover, modification of microbial DNA, mRNA and protein synthesis via diffused COS could lead to cell death.25 It was noted that C1-E0.5-conjugate had lower MIC than COS indicated higher antibacterial activity. EGCG has been known to possess antimicrobial activity against various Gram-positive and Gram-negative bacteria, which is caused by membrane disruption, inhibition of bacterial DNA gyrase, prevention of DNA supercoiling, thus leading to bacterial cell death.26 Therefore, conjugation of EGCG to COS synergistically enhanced antimicrobial activity of COS.COS had MBC of 0.1 and 2 mg mL−1 for E. coli and L. monocytogenes, while C1-E0.5-conjugate showed MBC of 0.05 and 1 mg mL−1, respectively. MBC/MIC ratio can be used to determine antibacterial potential of substances.14 Generally, an antibacterial agent having MBC/MIC ratio equal to or lower than 2 is considered as ‘bacteriostatic’. On the other hand, a ratio higher than 2 is considered as ‘bactericidal’. MBC/MIC ratios for COS against L. monocytogenes and E. coli were 1 and 2, respectively. For C1-E0.5-conjugate, the MBC/MIC ratios towards L. monocytogenes and E. coli were 1 and 5, respectively. Hence, COS could act as a bactericidal agent for both bacteria, whereas C1-E0.5-conjugate acted as bactericidal and bacteriostatic agent for E. coil and L. monocytogenes, respectively.
3.3. FTIR spectra
The stretching vibrations, bending vibrations of COS, EGCG and C1-E0.5-conjugate were determined using FTIR spectra (Fig. 2). For COS, the bands around 3434 and 2852–3009 cm−1 represent the stretching vibrations of O–H and C–H symmetric stretching vibrations in the polymer chains, respectively.27,28 The prominent peaks at 1641, 1560, and 1413 cm−1 were corresponding to the amide bands I (stretching vibrations of C–O), II (bending vibrations of N–H) and amide III (stretching vibrations of C–N), respectively.29–31 Peaks found at 800–1100 cm−1 were related to C–O, and C–C stretching vibrations, whereas region between 1400–1200 cm−1 was due to C–C–H, O–C–H, and C–O–H bending vibrations mode of mono or polysaccharides.29,32 For EGCG spectrum, band at 3500–3200 cm−1 was dominant, which was more likely associated with vibration of eight hydroxyl groups.9,10 Spectrum had two bands at 1613 and 1145 cm−1 related to C–C (aromatic ring) and C
O bonds (pyranose heterocyclic chain).9
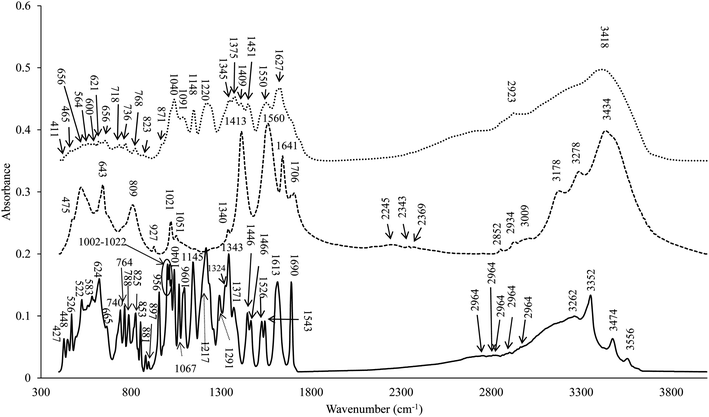 |
| Fig. 2 FTIR spectra of epigallocatechin gallate (EGCG) ( ) chitooligosaccharide (COS) ( ) and the selected conjugate (C1-E0.5) conjugate (⋯). C1-E0.5-conjugate was prepared using 1 wt% COS and 0.5 wt% EGCG. | |
For FTIR spectrum of C1-E0.5-conjugate, peaks within the range of 2900–3550 cm−1 appeared broader. The wavenumber 3434 cm−1 was shifted to the lower wavenumber (3418 cm−1) after conjugation with EGCG, indicating the interaction of EGCG with OH group of COS. Similarly, strong interaction of COS with EGCG was ascertained by the shift of wavenumber of 1641, 1560 and 1413 cm−1 to the lower wavenumbers, namely 1627, 1550 and 1409 cm−1, respectively. Sousa et al. also reported a decreasing intensity and a band-shift in the NH-bending region as a result of amide linkage (1627, 1550, and 1409 cm−1) of the catechin at C-2 of the glucosamine unit.33 The formation of new peaks at 1148 and 1220 cm−1 was more likely associated with hydroxyl groups or ether-cyclic nature of the EGCG.33 The prominent peak at wavenumber 1040 cm−1 in conjugate was more likely related to EGCG (as shown in EGCG spectrum), which represents the stretching of the aromatic and aliphatic C–O bond of catechin.34 Similarly, wavenumber 1627 cm−1 was more likely associated with peaks (1690 and 1613 cm−1) present in EGCG, which represents C
C stretching of cyclic alkenes.35 The formation of bands at wavenumbers of 1148 cm−1 in C1-E0.5-conjugate was related with wavenumber 1145 cm−1 in EGCG, which corresponds to the C–O bonds. Moreno-Vásquez et al. also reported that bands at 1606 and 1149 cm−1 were related to C
C and C–O bonds of EGCG.9 Furthermore, a decreased intensity of primary amine band at 1550 cm−1 and the increased intensity of amide II and amide I bands was noticed when COS was grafted with EGCG. This was more likely associated with stretching vibrations of C
C bond present in grafted EGCG.36 Therefore, changes in FTIR spectrum of the C1-E0.5-conjugate indicated the incorporation of EGCG at different binding sites of COS.
3.4. 1H NMR and 13C NMR analyses
1H NMR spectra of COS and C1-E0.5-conjugate are depicted in Fig. 3A and B, respectively. In COS spectrum, the signals at 5.08, 3.12 and 3.3–4.0 ppm were attributed to H1, H2 and H3–H6 proton in pyranose units of COS, respectively (Table 3). Moreover, protons from amino and hydroxyl groups also appeared at 1.94 ppm.37 Kim et al. also reported that the location of protons of glucopyranosyl ring was located between 3.3–4.6 ppm.38 When COS was conjugated, a new peak at 7.0 ppm was noticed, which was known as the zone of aromatic protons of EGCG (between 6.0 and 7.5 ppm). The formation of new peaks and weak downfield signals in the region of pyranose units (2.77–5.08) were also observed.
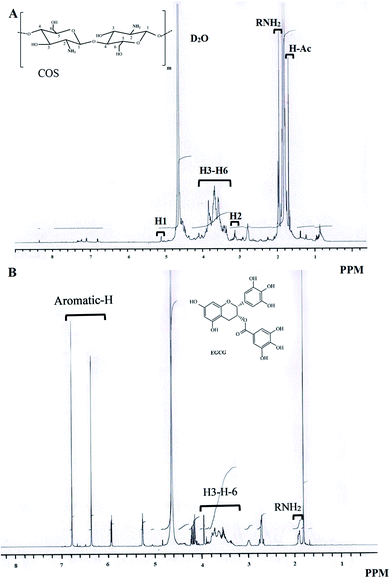 |
| Fig. 3 1H NMR spectra of chitooligosaccharide (COS) (A) and the selected conjugate (C1-E0.5) (B). C1-E0.5-conjugate was prepared using 1 wt% COS and 0.5 wt% EGCG. | |
Table 3 1H NMR and 13C NMR signals of the selected COS–EGCG conjugate in comparison with COSa
1H NMR |
13C NMR |
Source of H-signal |
δ (ppm) |
Source of C-signal |
δ (ppm) |
COS |
C1-E0.5-conjugate |
COS |
C1-E0.5-conjugate |
COS: chitooligosaccharide, C1-E0.5-conjugate: conjugate prepared using 1 wt% COS and 0.5 wt% EGCG. ND: not detected. *Samples showed similar signals (no chemical shifts after conjugation). A, B, C and D rings are belonging to EGCG structure (Fig. 4B). |
H1 |
5.08 |
* |
C2 |
58.98 |
59.02 |
|
|
|
C6 |
62.87 |
63.75 |
H2 |
3.12 |
* |
C5 |
74.7 |
* |
H3–H6 |
3.3–4.0 |
* |
C3 |
80.05 |
* |
|
|
|
C4 |
75.28 |
* |
NH- and OH-groups |
1.97 |
* |
C1 |
102.6 |
* |
|
|
|
A and C rings |
ND |
58–80 |
Aromatic protons |
ND |
7.0 |
B and D rings |
ND |
100–170 |
The 13C NMR spectra of COS and C1-E0.5-conjugate are revealed in Fig. 4A and B, respectively. In COS, the signals from carbon number 2, 6, 5, 3, 4 and 1 appeared at 58.98, 62.87, 74.7, 80.05, 75.28, and 102.6 ppm, respectively (Table 3).39 The similar peaks were obtained in 13C NMR spectrum of commercial COS as studied by Huang et al.19 The low signals were more likely due to the presence of paramagnetic centers which might alter the intensity of 13C NMR of different chitin or chitosan or COS materials.40 A typical 13C NMR spectrum of chitin/chitosan is influenced by the presence of anomeric (C-1) signals from both GlcN and GlcNAc at 102–105 ppm. Nevertheless, other carbon present at positions 3, 2, 4, 5 and 6 showed signals at 73–75, 55–57, 81–85 and 60 ppm, respectively.41
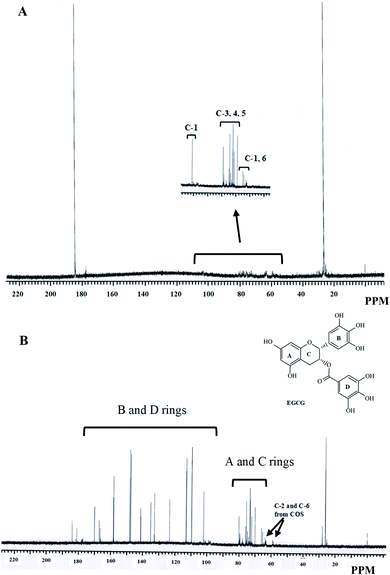 |
| Fig. 4 13C NMR spectra of chitooligosaccharide (COS) (A) and the selected conjugate (C1-E0.5) (B). C1-E0.5-conjugate was prepared using 1 wt% COS and 0.5 wt% EGCG. | |
For C1-E0.5-conjugate, a large number of peaks were observed in 13C NMR spectrum. Generally, EGCG showed the resonance at 100–170 ppm owing to the presence of aromatic rings (B and D rings, Fig. 4B and Table 3). The resonance for A and C aromatic rings of EGCG appeared between 58 and 80 ppm.42 Therefore, aromatic rings A and C might overlap the signals from carbons of COS. A chemical shift at C-2 of COS was noticed (from 58.98 to 59.02 ppm, Table 3) when EGCG was grafted. Moreover, C-6 of COS was also shifted to a higher resonance (from 62.87 to 63.75 ppm) when conjugated with EGCG. Both chemical shifts suggested the involvement of C-2 and C-6 of COS in binding with EGCG. The results were in agreement with the increase in TPC and antioxidative activities of C1-E0.5-conjugate. Hence, 1H and 13C NMR reconfirmed the successful grafting of EGCG at the various position of COS.
4. Conclusion
Chitooligosaccharide (COS) and epigallocatechin gallate (EGCG) conjugates were produced using COS and EGCG at various concentrations using a free radical grafting method. Conjugate prepared using 1 wt% COS and 0.5 wt% EGCG (C1-E0.5-conjugate) had higher total phenolic content, DRSA, ARSA and FRAP than others. When compared with COS, C1-E0.5-conjugate showed higher DRSA, ARSA, FRAP, ORAC, MCA and antimicrobial activity. FTIR, 1H-NMR and 13C-NMR analyses confirmed the successful grafting of EGCG into COS. Since, antioxidant activities of COS were enhanced tremendously, when conjugated with EGCG, the resulting conjugate could be used as a potential antioxidant incorporated in fatty foods to retard lipid oxidation.
Conflicts of interest
There are no conflicts to declare.
Acknowledgements
This study was funded by Postdoctoral Fellowship from Prince of Songkla University (PSU), Thailand to Dr Avtar Singh. Prince of Songkla University (Grant No. AGR6302013N) was also acknowledged.
References
- A. Singh, S. Benjakul and H. Kishimura, J. Aquat. Food Prod. Technol., 2017, 26, 1083–1092 CrossRef CAS.
- A. Singh, S. Benjakul and T. Prodpran, J. Food Sci., 2019, 84, 224–234 CrossRef CAS.
- G. Lodhi, Y.-S. Kim, J.-W. Hwang, S.-K. Kim, Y.-J. Jeon, J.-Y. Je, C.-B. Ahn, S.-H. Moon, B.-T. Jeon and P.-J. Park, BioMed Res. Int., 2014, 2014, 654913 Search PubMed.
- A. Singh, S. Benjakul and T. Prodpran, Int. J. Food Sci. Technol., 2019, 54, 2831–2838 CrossRef CAS.
- F. Liaqat and R. Eltem, Carbohydr. Polym., 2018, 184, 243–259 CrossRef CAS.
- D.-H. Ngo, Z.-J. Qian, D.-N. Ngo, T.-S. Vo, I. Wijesekara and S.-K. Kim, Food Chem., 2011, 128, 974–981 CrossRef CAS.
- Y. Zhong and F. Shahidi, Food Chem., 2012, 131, 22–30 CrossRef CAS.
- T. H. Quan and S. Benjakul, Colloids Surf., A, 2019, 579, 123711 CrossRef CAS.
- M. J. Moreno-Vásquez, E. L. Valenzuela-Buitimea, M. Plascencia-Jatomea, J. C. Encinas-Encinas, F. Rodríguez-Félix, S. Sánchez-Valdes, E. C. Rosas-Burgos, V. M. Ocaño-Higuera and A. Z. Graciano-Verdugo, Carbohydr. Polym., 2017, 155, 117–127 CrossRef.
- F. Lei, X. Wang, C. Liang, F. Yuan and Y. Gao, J. Appl. Polym. Sci., 2014, 131 Search PubMed.
- T.-K. Eom, B. Ryu, J.-K. Lee, H.-G. Byun, S.-J. Park and S.-K. Kim, J. Enzyme Inhib. Med. Chem., 2013, 28, 214–217 CrossRef CAS.
- J. M. Lorenzo and P. E. S. Munekata, Asian Pac. J. Trop. Biomed., 2016, 6, 709–719 CrossRef.
- J. Liu, H. Pu, S. Liu, J. Kan and C. Jin, Carbohydr. Polym., 2017, 174, 999–1017 CrossRef CAS.
- A. Singh, S. Benjakul and T. Prodpran, Food Production, Processing and Nutrition, 2019, 1, 5 CrossRef.
- M. Curcio, F. Puoci, F. Iemma, O. I. Parisi, G. Cirillo, U. G. Spizzirri and N. Picci, J. Agric. Food Chem., 2009, 57, 5933–5938 CrossRef CAS.
- L. Chotphruethipong, S. Benjakul and K. Kijroongrojana, J. Food Biochem., 2017, 41, e12379 CrossRef.
- T. Sae-Leaw, Y. C. O'Callaghan, S. Benjakul and N. M. O'Brien, J. Food Sci. Technol., 2016, 53, 197–208 CrossRef CAS.
- A. Singh and S. Benjakul, Innovative Food Sci. Emerging Technol., 2020, 102339, DOI:10.1016/j.ifset.2020.102339.
- R. Huang, E. Mendis and S.-K. Kim, Bioorg. Med. Chem., 2005, 13, 3649–3655 CrossRef CAS.
- T.-K. Eom, M. Senevirathne and S.-K. Kim, Environ. Toxicol. Pharmacol., 2012, 34, 519–527 CrossRef CAS.
- J. He, L. Xu, L. Yang and X. Wang, Med. Sci. Monit., 2018, 24, 8198 CrossRef CAS.
- Q. Hu, T. Wang, M. Zhou, J. Xue and Y. Luo, J. Agric. Food Chem., 2016, 64, 5893–5900 CrossRef CAS.
- S. Legeay, M. Rodier, L. Fillon, S. Faure and N. Clere, Nutrients, 2015, 7, 5443–5468 CrossRef CAS.
- C. Rice-evans, D. Leake, K. R. Bruckdorfer and A. T. Diplock, Free Radical Res., 1996, 25, 285–311 CrossRef CAS.
- A. Singh, A. Mittal and S. Benjakul, Food Rev. Int., 2020, 1–25, DOI:10.1080/87559129.2020.1734611.
- N. C. Gordon and D. W. Wareham, Int. J. Antimicrob. Agents, 2010, 36, 129–131 CrossRef CAS.
- J. Coates, Encyclopedia of analytical chemistry: applications, theory and instrumentation, 2006 Search PubMed.
- H. Wang, Y. Zhou, Y. Wang, Z. Wang and J. Wang, RSC Adv., 2018, 8, 41938–41949 RSC.
- A. Singh and S. Benjakul, Waste Biomass Valorization, 2019, 10, 3351–3361 CrossRef CAS.
- D. Katiyar, B. Singh, A. M. Lall and C. Haldar, Eur. J. Pharm. Sci., 2011, 44, 534–543 CrossRef CAS.
- D. Usoltsev, V. Sitnikova, A. Kajava and M. Uspenskaya, Biomolecules, 2019, 9, 359 CrossRef CAS.
- V. K. Mourya, N. N. Inamdar and Y. M. Choudhari, Polym. Sci., Ser. A, 2011, 53, 583–612 CrossRef CAS.
- F. Sousa, G. M. Guebitz and V. Kokol, Process Biochem., 2009, 44, 749–756 CrossRef CAS.
- R. Soto, J. Freer and J. Baeza, Bioresour. Technol., 2005, 96, 95–101 CrossRef CAS.
- V. Roucoules, C. A. Fail, W. C. Schofield, D. O. Teare and J. P. S. Badyal, Langmuir, 2005, 21, 1412–1415 CrossRef CAS.
- R. A. Muzzarelli, G. Littarru, C. Muzzarelli and G. Tosi, Carbohydr. Polym., 2003, 53, 109–115 CrossRef CAS.
- D.-N. Ngo, M.-M. Kim and S.-K. Kim, Int. J. Biol. Macromol., 2012, 50, 624–631 CrossRef CAS.
- J. Y. Kim, J. K. Lee, T. S. Lee and W. H. Park, Int. J. Biol. Macromol., 2003, 32, 23–27 CrossRef CAS.
- L. Rui, M. Xie, B. Hu, L. Zhou, M. Saeeduddin and X. Zeng, Carbohydr. Polym., 2017, 170, 206–216 CrossRef CAS.
- M. L. Duarte, M. C. Ferreira, M. R. Marvão and J. Rocha, Int. J. Biol. Macromol., 2001, 28, 359–363 CrossRef CAS.
- J. Kumirska, M. Czerwicka, Z. Kaczyński, A. Bychowska, K. Brzozowski, J. Thöming and P. Stepnowski, Mar. Drugs, 2010, 8, 1567–1636 CrossRef CAS.
- R. Seto, H. Nakamura, F. Nanjo and Y. Hara, Biosci., Biotechnol., Biochem., 1997, 61, 1434–1439 CrossRef CAS.
|
This journal is © The Royal Society of Chemistry 2020 |
Click here to see how this site uses Cookies. View our privacy policy here.