DOI:
10.1039/D0RA04432F
(Review Article)
RSC Adv., 2020,
10, 28608-28629
Heteroatom-doped graphene as sensing materials: a mini review
Received
18th May 2020
, Accepted 14th July 2020
First published on 4th August 2020
Abstract
Graphene is one of the astounding recent advancements in current science and one of the most encouraging materials for application in cutting-edge electronic gadgets. Graphene and its derivatives like graphene oxide and reduced graphene oxide have emerged as significant nanomaterials in the area of sensors. Furthermore, doping of graphene and its derivatives with heteroatoms (B, N, P, S, I, Br, Cl and F) alters their electronic and chemical properties which are best suited for the construction of economical sensors of practical utility. This review recapitulates the developments in graphene materials as emerging electrochemical, ultrasensitive explosive, gas, glucose and biological sensors for various molecules with greater sensitivity, selectivity and a low limit of detection. Apart from the most important turn of events, the properties and incipient utilization of the ever evolving family of heteroatom-doped graphene are also discussed. This review article encompasses a wide range of heteroatom-doped graphene materials as sensors for the detection of NH3, NO2, H2O2, heavy metal ions, dopamine, bleomycinsulphate, acetaminophen, caffeic acid, chloramphenicol and trinitrotoluene. In addition, heteroatom-doped graphene materials were also explored for sensitivity and selectivity with respect to interfering analytes present in the system. Finally, the review article concludes with future perspectives for the advancement of heteroatom-doped graphene materials.
1. Introduction
After mechanical exfoliation of graphene monolayers by Novoselov in 2004, the area of nanocomposites has sparked immense interest that has led to innovations in graphene-based materials for significant applications.1 It has appeared as an influential material on the horizon of material science, and is utilized in each and every field of research after its advent.2,3 Graphene is also considered as a perfect substitute for carbon nanotubes which makes it an outstanding material for photonic devices that require transparent and conducting thin films.4,5 The 3D graphene derived from 2D graphene has been the focal point of interest, not just on the ground that it can preserve features of 2D graphene, but also due to its own unique properties like flexible pore structures, enhanced mechanical strength and electronic conductivity. These properties played a vital role in the development and modification of multi-functional catalysts with enhanced properties.6 Furthermore, graphene and its oxidized derivatives like graphene oxide (GO) contain several oxygen functional groups such as hydroxyl, epoxy, carbonyl etc. which make the graphene sheets more hydrophilic, allowing them to inculcate different types of nanoparticles or nanoclusters, with prominent applications in sensors. In comparison to this, reduced GO has a high density of defects which further enhance the electrochemical activity of sensors.
In terms of condensed matter perspective, graphene has been fabricated with sp2 bonded carbon atoms through hybridization of s, px and py atomic orbitals, making 3 strong σ bonds with 3 adjoining atoms. The fourth pz orbital on every carbon atom interacts with nearest atoms, creating a valence band and conduction band. Consequently, graphene may be regarded as a metal with evaporating Fermi surface or a semiconductor with the absence of a substantial band gap.7,8 The applications of pristine graphene have been restricted to a great extent, due to the absence of an intrinsic bandgap, in the field of nanoelectronics, sensing, electrocatalysis and energy storage. Hence, it is attractive to encourage a band gap in graphene to promote the above-mentioned applications.9–11 A few strategies have been proposed to open the electronic band gap in graphene, like molecular adsorption, confinement, chemical functionalization, multilayer graphene and edge-effects-induced bandgap.12–14 For instance, novel electronic properties emerge when different morphologies of graphene are grown which are highly dependent on the size and edge lattice symmetry.15 Apart from morphology control, chemical doping with external particles is likewise a powerful strategy to modify electronic properties, control surface science and alter the natural structure of host materials.16 Doping, especially chemical doping, is one of the approaches to recondition the electronic, chemical, and magnetic properties of materials, and is usually of two types.17,18 The first type, known as surface transfer doping, involves the adsorption of foreign agents onto the surface of graphene which do not cause sp3 defects in the graphene cross-section. The second type, known as substitutional doping, involves the disruption of the sp2 network by foreign agents which create sp3 defect regions through covalent bonding with graphene. The chemical doping of heteroatoms like boron (B), nitrogen (N), phosphorus (P), sulfur (S), silicon (Si), fluorine (F), chlorine (Cl), bromine (Br) and iodine (I) into graphene will certainly cause structural and electronic alterations. These alterations further successfully lead to modification in the properties of graphene material, such as thermal stability, charge transport, Fermi level, bandgap, localized electronic state, spin density, optical characteristics, and magnetic properties. On the basis of nature and bonding configuration of the dopants, enhanced properties may appear and be advantageous for specific applications. Better knowledge on how the heteroatom doping can customize the properties of graphene is imperative for researchers to design/discover novel functionalities and extend the range of applications of graphene materials.
The electrochemically active sites induced by doped heteroatoms are favourable for the adsorption and activation of analytes, anchoring of functional moieties or molecules, and accelerating the charge transfer between electrode and analyte/electrolyte, all of which would favour an enhanced electrochemical sensing performance.19 Chemical doping with heteroatoms is a key strategy to alter electronic properties and chemical reactivity of graphene as the size and electronegativity of heteroatoms are particularly different from those of carbon atoms. The incorporation of heteroatoms gives new features to graphene, for employing in numerous applications.20 It has been reported that chemically modified graphene shows excellent properties like superconductivity, ferromagnetism, and enhanced chemical and electrochemical activity.21
Here, we aim to focus on the doping of different graphene materials with heteroatoms (B, N, P, S, Si, F, Cl, Br, I) and their prominent applications in sensing. Although several reports are available on specific dopants, their synthesis or particular applications, a more comprehensive review on this matter is still desired. There are no review articles on electrochemical, electrical, ultrasensitive, glucose, bio and gas sensors based on heteroatom-doped graphene materials. Therefore, this review is aimed at summarizing the recent advances in sensors employing heteroatom-doped graphene materials. Sensor systems to detect toxic gases like CO, NH3, and NO2, dopamine, ascorbic acid, uric acid, dinitrotoluene, heavy metals etc. are discussed. This review article will provide a single reference source for researchers concerned with electrochemical, electrical, ultrasensitive, glucose, bio and gas sensors based on heteroatom-doped graphene materials. Therefore, this review will be of interest to an extensive scope of audiences from various research fields and stimulate more curiosity in heteroatom-doped graphene-based sensors for upcoming sensor industries.
2. Sensors
In recent years, an enormous number of sensors and biosensors based on graphene and its related materials have been accounted for in: (a) clinical applications, for example, for detection of glucose, cholesterol, H2O2, dopamine, ascorbic acid and uric acid; (b) environmental applications, for instance, for detection of heavy metal ions and pesticides; and (c) food sciences, for instance, for detection of erythromycin, tryptamine, and Staphylococcus aureus.22 Various analytical parameters of merit like sensitivity, limit of detection, repeatability and reproducibility have been measured to evaluate the analytical performance of sensors.23 The sensitivity and limit of detection can be influenced by the morphological characteristics of sensors, and these parameters of importance should be measured to find analytical reliability and capacity of sensors. Heteroatom-doped graphene materials have attracted great attention of mainstream researchers because of their enhanced physicochemical, optical, electromagnetic and structural properties, when contrasted with undoped graphene.7 The doping of heteroatoms in the graphene structure is known to enhance the wettability of graphene materials. Graphene derivatives doped with heteroatoms are highly promising materials for applications such as energy storage, fuel cells, electrocatalysis, sensing etc. The detection of targets by graphene materials is mostly dependent on their conductance changes upon the adsorption of the species to be sensed. Heteroatom doping in graphene has many possible aspects like oxygen reduction reaction (ORR), hydrogen evolution reaction (HER) and oxygen evolution reaction which have been described by catalyst electrodes without any external support.24 The heteroatoms doped in graphene materials are mainly covalently bonded to the carbon atoms of graphene. The extraordinary features of graphene such as high specific area, 3D conductive networks and porous structure allow the heteroatoms to be reliably and easily doped.
2.1 Boron-doped graphene (BG)-based sensors
Doping of graphene with B (2s22p1) has been shown to be energetically favourable because the formation energy of BG in the case of gaseous dopant is ∼5.6 eV per atom, much lower than that of N-doping (8.0 eV per atom).25 Boron atoms in the carbon (C) lattice are sp2-hybridized for in-plane doping, permitting maintenance of the planar structure of graphene because of the similarities among B and C atoms; still, the grid boundaries are somewhat modified in the light of the fact that the B–C bond (∼1.50 Å) is longer than the C–C bond (1.42 Å). On the other hand, for out-of-plane B-doping, the dopants at vacant sites with BC4 units cause some distortion in the planar structure of graphene; however, due to instability, all the starting positions would relax to similar in-plane positions.26 As B has one valence electron less than the adjoining C, doping of B induces a charge polarization in the graphene matrix (turns out to be negatively charged) which is advantageous for p-type conduction, by downshifting the Fermi level towards the Dirac point.27–29 The B-doping offers extra holes to the valence band of graphene, which results in enhanced conductivity as well as carrier concentration in BG.30
2.1.1 Electrochemical sensors. Doped graphene materials are useful in enhancing the performance of electrochemical sensors. This is due to the fact that they promote charge transfer, adsorption and activation of analytes, and anchoring of functional molecules by introducing electrochemically active sites through heteroatom doping. These materials also eliminate the need of recognition elements or mediators, providing affordable and stable sensors. Boron (2s22p1), a neighbouring element to carbon (2s22p2) with only one less valence electron, is quite suitable for doping in graphene. The planar structure can be retained because of sp2 hybridization of B atoms in the C lattice. In-plane bonding has proved to be more stable as compared to out-of-plane bonding.31 NaBH4 (source of B dopant atoms as well as a reductant) was used for the synthesis of BG nanosheets (NS) when GO was treated in the presence of poly(diallyldimethylammonium chloride). The electrochemical applications of this synthesized nanocomposite were illustrated by various characterization techniques, and the electrocatalytic activity range was observed to be 1 × 10−6 to 7.5 × 10−5 mol L−1 for guanine. The oxidation and sensing applications of graphene nanosheets were modified by B-doping into the graphene structure. It also exhibited detection limit of 3.9 × 10−7 mol L−1 which makes B-doped nanocomposite further applicable for electrochemical sensor fabrication.32 In another study, thermally reduced GO at 700 °C on treatment with borane–tetrahydrofuran complex induced boron substitution of about 2% into the graphene lattice. This (BH3–THF) adduct also proved to be a better borylating agent than B2O3, H3BO3, NaBH4, NH3BH3, and carborane.33 Recently, Ghanbari and co-workers designed a novel electrochemical sensor for the detection of traces of flunitrazepam, based on electropolymerized β-cyclodextrin (Eβ-CD)/boron-doped reduced graphene oxide (B–rGO) composite material.34 Electrochemical studies were performed using differential pulse voltammetry (DPV) and cyclic voltammetry (CV) techniques, by employing the material as a glassy carbon electrode (GCE). Experimental data confirmed two linear ranges for the calculation of flunitrazepam between 2.0 nmol L−1 to 0.5 μmol L−1 and 0.5 μmol L−1 to 20.0 μmol L−1. For the modified GCE, the limit of detection (LOD) and sensitivity were found to be 0.6 nmol L−1 and 8.4 μA μmol L−1 cm−2, respectively.
2.1.2 Ultrasensitive explosive sensor. The mounting danger posed by the utilization of improvised explosive devices in military and non-military populaces has necessitated the development of explosive analogous synthetic fume sensors.35,36 The detection of explosive materials like dinitrotoluene (DNT) and trinitrotoluene (TNT) is crucial and particularly obligatory for national safety and environmental management.37 Sensors employing pristine graphene, GO and nanopatterned graphene have revealed exceptional potential in the detection of gases on a trace level which are hazardous to humans and the environment.38 There are certain reports in literature which claimed that the doping of heteroatoms like B or N would exceptionally improve the electronic properties of graphene and attracted ample attention.39,40 But, the use of sensors based on heteroatom-doped graphene materials in the trace detection of explosives has seen very little investigation. However, an ultra-sensitive explosive sensor was reported which could be utilized in anti-terrorism and several environmental problems. A sensitive high melting explosive (HMX) sensor was fabricated by a novel BG-modified GCE. The electrochemical conductivity of HMX on modified materials was investigated employing CV (Fig. 1). Under the streamlined conditions, CV was utilized for the quantitative detection of HMX and showed linear dependence in the concentration ranges of HMX between 2–20 and 2–100 μM, with a low detection limit of 0.83 μM (245.81 ppb).41
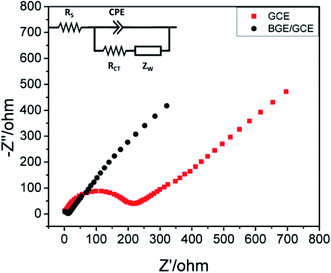 |
| Fig. 1 EIS diagram of bare GCE and B–GE/GCE in an electrolyte containing 0.5 mM [Fe(CN)6]3−/4− and 0.1 M KCl. Impedance spectra were recorded over a frequency range of 1000 kHz to 100 MHz with amplitude of 5 mV [reprinted with permission from ref. 41, Copyright© Elsevier]. | |
2.1.3 Gas sensors. Recently, air contamination has become a worldwide concern, and detection of noxious gases is of profound importance. Hence, a large number of researches are centered around the development of reasonable solid-state gas sensors. It is notable that the adsorption of gas molecules on carbonaceous materials has been widely investigated for a long time.42,43 Pristine graphene fails to detect some gas molecules because of feeble interaction, and eventually inadequate charge transfer between the adsorbates and graphene sheet.44 For the last decade, to overcome the insensitivity of pristine graphene to gas molecules, many research groups have been decorating these materials with some foreign substances to enhance the interaction between gas molecules and graphene substrates.45 Furthermore, it was observed that heteroatoms on graphene can remarkably alter the electronic properties, by utilizing first-principles methods. What is more, when gaseous particles are adsorbed on graphene sheet, these show up as changes in the resistivity, which makes it possible for graphene to be a solid-state sensor to distinguish a specific gas from others.46Good quality BG sheets were prepared by using a liquid precursor of triethylborane/hexane solution with a large area, high crystallinity and controlled growth. Scanning tunnelling microscopy revealed p-type conductivity and croissant-like features corresponding to B3-dopants embedded within the hexagonal BG lattice. BG exhibited unique sensing capabilities for toxic gases like NO2 and NH3, even at low concentrations (e.g., parts per trillion, parts per billion). Boron doping effectively gives enhanced sensitivity, i.e. 27 and 105 times for NO2 and NH3, respectively.47 Another study used density functional theory calculations for activating gas sensing applications (adsorption of gas molecules like CO, NO, NO2, H2O) of graphene doped with B-atoms. Observations revealed that there is no change in structural and electronic properties of BG surface, before and after CO and H2O adsorption, while these properties show sensitivity towards NO and NO2 adsorption because of strong molecule–graphene interactions. Thus, results established BG as a perfect candidate for gas sensing, particularly for NO and NO2.48 On the other hand, graphene quantum dots (GQD) and B-doped GQD (B–GQD) behave as p-type semiconductors which when combined with Ag–LaFeO3 (AL) gave p-type B–GQD, exhibiting a synergic effect. Low operating temperature (55 °C), excellent selectivity and high stability towards HCHO and highly sensitive nature also make B–GQD–AL a good sensor for volatile organic compounds at room temperature.49 Recently, Peng et al. fabricated a high-performance triethylamine (TEA) gas sensor, employing BG coated Au@SnO2 heterostructure.50 At moderately low temperature of 100 °C, the response to 1 ppm of TEA gas touched 69.1%, with LOD as low as ppb level. The sensor was highly selective to TEA gas, and selectivity was nearly 60 times higher than that for ethanol, methanol, and acetone. In another investigation, Srivastava and co-workers fabricated a boron-doped few-layer graphene (BFLGr)-based sensor for the sensing of ammonia gas. The as-prepared sensor shows tremendously rapid response for ammonia gas sensing under normal conditions. The results obtained indicated a new strategy to construct handy and quick gas sensing devices based on BFLGr.51
2.1.4 Biosensors. Mannan et al. synthesized GO using a modified Hummers method and successful boron doping (1.64–1.83%) was executed with boric acid as a precursor by hydrothermal reaction. The B–rGO permitted high adsorption (65–70%) which makes it suitable for biosensing application via glucose adsorption (Fig. 2).52
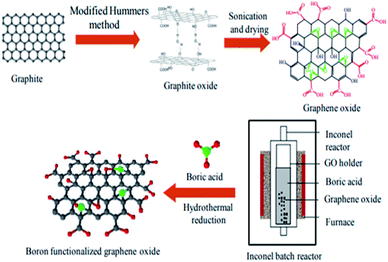 |
| Fig. 2 Schematic representation of synthesis of graphene oxide and boron doping by hydrothermal reaction [reprinted with permission from ref. 52, Copyright© Elsevier]. | |
The BG was cut into BG quantum dots (BGQDs) using a hydrothermal method. The glucose sensing is enhanced by creation of boronic acid groups on the BGQD surface which worked on the mechanism of restriction of intramolecular rotations, activated by the peculiar BGQDs–glucose interactions which further enhanced the photoluminescence of BGQDs.53
2.2 Nitrogen-doped graphene-based sensors
In carbon technology, plans for doping of nitrogen into the framework of carbon-based materials have been expanding rapidly, so as to achieve useful semiconducting properties.54–56 The main reason for the ease of incorporation of nitrogen in graphene structure is its comparable atomic size with carbon atoms, and formation of strong bonds with each other.57,58 However, the large electronegativity difference in N (3.04) and C (2.55) produces polarization in the carbon network, thus prompting the electronic, magnetic, and optical properties of graphene.59 Furthermore, the incorporation of nitrogen in graphene-based materials enhances the charge carrier density due to the involvement of p-electrons of nitrogen with the π-system of graphene. Hence, nitrogen and groups based on it play a significant part in the electrochemical study of N-doped materials.60,61 Sliwak et al. synthesised N-doped GO and employed the material as electrodes for high rate supercapacitors.62 It was revealed from cyclic voltammetry and impedance spectroscopy measurements that nitrogen doping improved ion diffusion and charge propagation of the material. The rate capacity for N-doped and non-doped GO with increasing scan rate was found to be 98% and 70%, respectively. Similarly, a novel strategy was adopted to synthesise nitrogen-doped graphene on a large scale from s-triazine molecules.63 The post-annealing of N-doped graphene, followed by gold intercalation, results in a change of the N environment from pyridinic to graphitic, and over 80% of embedded nitrogen was in graphitic form. Angle-resolved photoemission spectroscopy has detected a charge carrier concentration of ∼8 × 1012 electrons cm−2, induced by 0.4 atom% of graphitic nitrogen. The results obtained offer considerable potential for the application of the proposed system in next-generation electronic devices.
2.2.1 Electrochemical sensors. Graphene has been found to be a most promising electrode on the basis of electroanalysis methods. Numerous electrochemical sensors based on graphene and graphene composites have been fabricated.64 Nitrogen-doped graphene nanosheets (N–SEGN) were prepared via hydrothermal reaction of tetra-2-pyridinylpyrazine and sonoelectrochemically exfoliated graphene nanosheets, which appeared to be more catalytic towards H2O2 sensing. In this, nitrogen-doped chemically reduced graphene oxide (N–rGO) was formed with graphitic nitrogen for usage as a non-enzymatic H2O2 sensor. N–rGO gives higher sensitivity of 57.3 μA mM−1 cm−2 as compared to N–SEGN with 231.3 μA mM−1 cm−2 sensitivity.65 Nitrogen can also be doped to customize nitrogen-based graphene quantum dots by many methods like thermal, ultrasonic, solvothermal, hydrothermal and electronic beam approaches. Compounds such as metal oxides, nitrides, polymers and semiconductors are picked for blending with both nitrogen-doped graphene and nitrogen-doped graphene quantum dots (Fig. 3).66
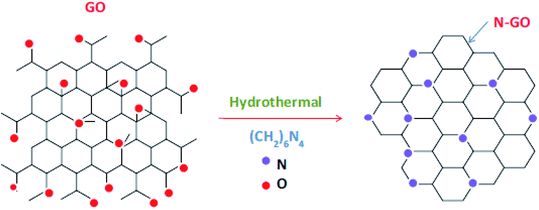 |
| Fig. 3 Preparation of N–GO by hydrothermal method [reprinted with permission from ref. 66, Copyright© Elsevier]. | |
Nitrogen-doped GO materials can be produced by four different methods, Staudenmaier (ST-GO), Hummers (HU-GO), Hofmann (HO-GO) and Brodie oxidation (BR-GO), using graphite oxide as precursor, with increasing order of ST-GO < BR-GO < HO-GO < HU-GO, for electrochemical sensing of different biomolecules. Furthermore, their performance for the detection of six biomolecules, i.e. ascorbic acid, uric acid, dopamine, NADH, adenine and cytosine, was compared, and HU-GO was found to be the best performing material.67 Electrochemical sensors based on nitrogen-doped graphene sheets (NGS) were also used as anticancer drug sensors. Nanosheet graphene (NrG) doped in 1-methyl-3-octylimidazolium chloride (MOICl) was used for this purpose. The detection limit of NrG/MOICl/CPE for anticancer agents doxorubicin and topotecan was found to be as low as 3.1 nM and 0.27 μM, respectively. It was observed that NrG/MOICl/CPE exhibited good electrical conductivity and catalytic activity.68 Some sensors modified with screen-printed carbon electrodes (SPCE) for detection of nicotine in urine and tobacco samples have also been reported (Fig. 4).69
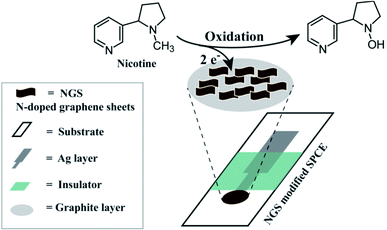 |
| Fig. 4 Representation of nicotinic oxidation process of NGS–SPCE [reprinted with permission from ref. 69, Copyright© Elsevier]. | |
This type of sensor was further investigated for electrochemical oxidation of NADH with some modification by GCE. It exhibited excellent performance with high detection sensitivity of 0.627 mA cm−2 mM−1 and low detection limit of 47 nM for nicotine. It was observed that the specific capacitance of NDG is higher than that of GCE and GO/GCE.70 Xu et al. discussed electrical conductivity and biocompatibility of N-doped graphene in tetra-sulfonated phthalocyanine, to further improve the sensitivity and stability of sensors. The detection of dopamine by adding ascorbic acid (AA) and uric acid (UA) gives low detection limit of 100 nM and better sensitivity of 88.9 μA mM−1.71 Nitrogen-doped graphene reduced with urea has lower doping content as compared to that reduced with pyridine-N. Si et al. showed that NGE–N modified electrode exhibited a low detection limit of 0.55 mM for imidacloprid (Fig. 5).72 The syntheses of NGE–N and for comparison NGE–C are shown in Fig. 5.
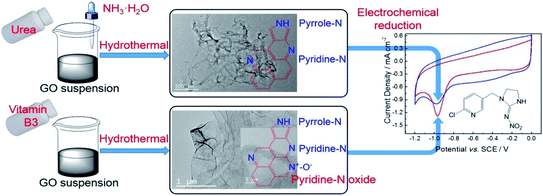 |
| Fig. 5 Synthesis scheme for NGE–N and NGE–C using hydrothermal method [reprinted with permission from ref. 72, Copyright© Elsevier]. | |
H2O2 plays an important role as mediator in various physiological and pathological processes, and is widely used in oxidative biosynthetic reactions. It can easily be reduced by heteroatom-doped graphene. Cai et al. reported facile 3D N-doped graphene aerogel electrode for H2O2 sensing by incorporating dopamine. The sensor exhibited high electrocatalytic efficiency for H2O2 estimation with detection limit of 0.05 mM which is better than that of other enzyme-based sensors (Fig. 6).73
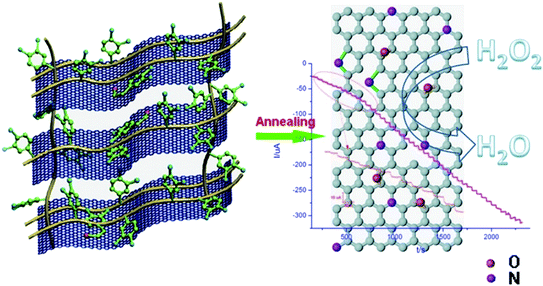 |
| Fig. 6 Schematic illustration of 3D N-doped graphene electrode incorporated with dopamine [reprinted with permission from ref. 73, Copyright© Elsevier]. | |
On the other hand, an enzyme-free H2O2 sensor was reported by Shao et al. which is also based on N-doped graphene electrode. This sensor exhibited wide linear detection range of 10−5 to 2.8 mM. This is because of the weakening of the O–O bond of H2O2 by the charge induced by nitrogen.74 Wu et al. also developed N-doped graphene for H2O2 sensing with a linear range of 0.5–1.2 mM and low detection limit of 0.05 mM, with improved performance. This is attributed to the doping of N which reduces the HOMO–LUMO gap. Furthermore, the dynamic process of release of H2O2 in living cells was also demonstrated which is another advantage of the sensitivity and specificity of this sensor.75 In a recent study, Yang et al. prepared nitrogen-doped graphene quantum dots (N–GQDs) for the fluorometric detection of Fe3+ ions. The outstanding sensitivity for Fe3+ ions was attributed to the great interaction between groups like hydroxyl, carboxyl, basic pyridinic nitrogen, and nitro present on N–GQDs and electron-deficient Fe3+ ions. The Fe3+ ions were determined in the linear concentration range of 10–1000 μM and a low detection limit of 0.19 μM.76 In another recent report, an environment friendly vitamin B12 functionalized N-doped graphene (Vit.B12–NGr) was designed for the electro-oxidative sensing of H2O2. The nanohybrid material established itself as a favourable electrocatalytic surface for the electro-oxidative sensing of H2O2, with a sensitivity of 430 μA mM−1 and 20 nM as LOD.77
2.2.2 Gas sensors. Gas sensing properties of graphene are highly unpredictable on target gases. Many methods such as atomic doping, molecular impregnation, and chemical functionalization of graphene materials have been employed for gas sensing applications. The defects in the basal plane enhance the conducting matrices of gas sensors.78 The gas molecule binds more firmly with vacant-heteroatom structure and deliberately controls the focus and morphological aspects of dopants. Nitrogen doped on carbon nanotubes gives a strong approach towards gas detection, particularly nitrogen dioxide.79 Heteroatom-based sensors operated at high temperature with higher sensitivity, have longer cyclability lifetime and maintain a strategic distance from metal surface dispersion or Ostwald ripening type of issues. Hence, N-doped CNTs exhibit the best sensitivity towards nitrogen dioxide and carbon monoxide.In an effort to develop magnetic sensors for detection of toxic gases, pyridinic-N-doped graphene was synthesized which was employed for the detection of CO as it is better chemisorbed on this material.80 It has been observed that the edge defects in reduced graphene play a vital role in its gas sensing performance. This sensor was able to detect NO2 and recovered its initial states by flowing N2 without thermal assistance.81 The heteroatom-doped rGO-based room temperature NO2 sensors displayed outstanding sensing performance. Heteroatom doping was done by a hydrothermal process using SnCl4, GO and urea as precursors, to synthesize hybrid SnO2/N–rGO which exhibited excellent sensing property for NO2.82 Graphene–ammonia sensors having two different adsorption processes, one at the top surface of graphene such as for SiO2 and another at the bottom side, close to the substrate, for NH3 molecules, were investigated. The charge transfer depends mainly upon temperature, gas concentration, and average distance between the graphene sheet and the substrate. It was also observed that the electron charge transfer is higher during interaction of graphene sheets and ammonia molecules.83
2.2.3 Biosensors. The 3-D nitrogen-doped graphene synthesized via chemical vapour deposition, using porous nickel foam as a substrate, was employed in the fabrication of a biosensor with high electrocatalytic activity for electrochemical detection of dopamine.84 This type of biosensor is also employed for pharmaceutical preparations such as tablets. The NGE modified GCE was used for the quantitative detection of nimodipine. This biosensor has a low detection limit of 9.1 nM, with a wide linear range of 0.02–3 μM of nimodipine (Fig. 7)85 whereas a combination of CdTe quantum dots with N-doped graphene on the GCE showed long-time stability and high electro-chemiluminescence (ECL) intensity factor, and was employed for the detection of ascorbic acid (AA) on the basis of ECL mechanism. The sensor exhibited a low detection limit of 0.015 μM for AA, with a linear response range of 0.04 to 200 μM (ref. 86) which is greater than that of the uncombined sensor.
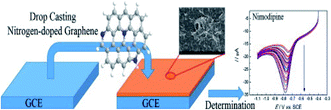 |
| Fig. 7 Preparation of nitrogen-doped graphene for nimodipine sensing [reprinted with permission from ref. 85, Copyright© Elsevier]. | |
The N-doped graphene synthesized by thermal annealing of graphite oxide with melamine also showed outstanding electrocatalytic activity, with detection limits of 2.2 × 10−6 M, 2.5 × 10−7 M and 4.5 × 10−8 M at S/N = 3 with respect to ascorbic acid, dopamine and uric acid, respectively.87 A N–GQD-based biosensor was also fabricated for the detection and estimation of bleomycinsulphate (BLM) in human serum samples. The carboxyl groups on N–GQDs lead to strong adsorption of ssDNA on its surface, resulting in effective fluorescence quenching of N–GQDs (Fig. 8).88 Thus, it can be concluded that N-doped graphene is an excellent candidate for the fabrication of selective biosensors for bioelectronics and other biocatalytic applications.
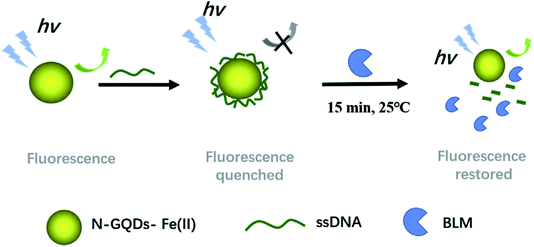 |
| Fig. 8 Schematic illustration of the N–GQDs–Fe(II) sensing system for the detection of BLM [reprinted with permission from ref. 88, Copyright© Elsevier]. | |
In another investigation, femtomolar miRNA detection was reported using laser induced self-N-doped porous graphene as an electrochemical biosensor.89 In order to detect the target miRNAs, they were purified with laser induced graphene sensor using miRNA extraction and magnetic isolation process. The results obtained revealed the detection of miRNA until the concentration reaches 10 fM. The fabricated sensor exhibited great prospects for sensing miRNA in biomedical applications, due to simplistic design and better execution.
2.2.4 Electrical sensors. Graphene-based electrical sensors are mainly used in lithium-ion batteries, photodetectors, invertors, and optoelectronics. The main purpose is to dope graphene with materials like boron or nitrogen, to discharge the capacity of batteries, store energy, and to increase the power consumption. The electrical sensing targets include different metallic ions at low temperature like cadmium, lead, and mercury. Also, calcium, potassium and hydrogen were detected by these sensors.90 The reduction of iodide ion using MoS2/nGO nanocomposite-based platinum-free low-cost dye-sensitized solar cell (DSSC) was investigated which has an excellent photovoltaic conversion efficiency.91 Low charge transfer resistance, high electrocatalytic activity and fast reaction kinetics were observed for the reduction of tri-iodide ion to iodide ion at the electrode–electrolyte interface. Ranganathan et al. reported a photoanode for DSSCs, prepared using nitrogen-doped graphene@nickel oxide (NGE/NiO) nanocomposite-doped TiO2. This nanocomposite is accepted to have better DSSC performance with respect to power conversion efficiency than GO/TiO2 and NiO/TiO2 based photoanodes. Also, NGE/NiO/TiO2 nanocomposites show large dye loading, and light scattering with lower recombination rate (Fig. 9).92
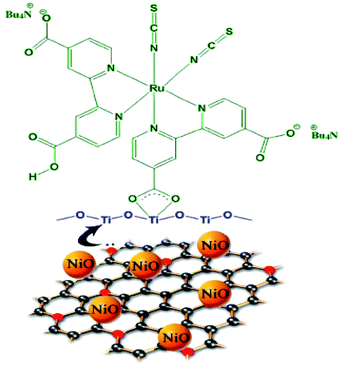 |
| Fig. 9 Graphical representation of attachment of N719 dye on NGE/NiO-modified TiO2 surface [reprinted with permission from ref. 92, Copyright© 2014 Elsevier]. | |
Ou et al.93 reported ZIF-8/GO on N-doped carbon sheets with ZIF-8 GO, realized by pyrolysis at different temperatures which combined porous carbonaceous materials and GO for DSSCs. It has been shown that the fabricated metal-free electrode in DSSCs gives electrocatalytic activity towards redox couple of I3−/I− with a power conversion efficiency of 8.2%, which is greater than that of a Pt counter electrode which is 7.6%. Abid et al.94 highlighted a wideband optical sensor used for photodetection such as sensitivity, quantum roles, bandwidth and flexibility. From an economic point of view, graphene is notably good at interacting with silicon for optoelectronic and photonics circuits.
2.2.5 Glucose sensors. Glucose is a main component in blood to provide energy, but its excess may cause death. Glucose sensors with graphene-based enzymatic as well as non-enzymatic detection are of tremendous importance. The enzymatic glucose sensors are quite sensitive and selective whereas non-enzymatic sensors have attracted a lot of attention due to their stability, reliability and sensitivity. The comparison of graphene-based sensors and graphene-less ones has revealed that graphene-based sensors are better in all aspects such as sensitivity and detection limit. Heterogeneous electron/proton transfer constant may get augmented with the reduction of graphene in-plane conductivity because of oxygenated groups present on the edges of graphene planes.95 NiO/nitrogen carbon sphere nanocomposites (NiO/NCS) were synthesized via a one-pot method in which urea was used as a source of nitrogen. This NiO/NCS modified GCE composite exhibited better sensitivity to glucose detection than nitrogen-free NiO/CS electrode, due to increased conductivity, and hence enhanced electron transfer within the N-doped composite. This electrode gave two linear regions of 1–800 μM and 4–9 mM, having sensitivity of 398.57 A mM−1 cm−2 and 17.81 μA mM−1 cm−2, and detection limit of 0.25 μM and 0.05 mM, respectively.96 Likewise, nanoneedle-like copper oxide on N-doped reduced GO also exhibited high electrocatalytic activity for glucose detection. This non-enzymatic sensor was used for monitoring glucose level in blood serum samples by amperometric test that involved the detection of current at certain applied potential. This sensor gave better results in terms of selectivity, with a good linear concentration range of 0.5–639 M and 0.01 M detection limit97 (Fig. 10). Sivasankar et al. also analyzed human serum samples for glucose by CuNPs/NGO which was prepared by direct carbonization of [Cu2(BDC)2(DABCO)] MOF.98 It exhibited a linear concentration range of 1–1803 μM with a detection limit of 0.44 μM. It also showed good sensitivity of 2500 μA mM−1 cm−2. The real benefit of CuNPs/NGO/SPCE nanocomposite for glucose detection which can be used in future is its commendable catalytic activity for batteries, biosensors and supercapacitors.
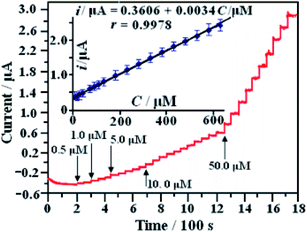 |
| Fig. 10 i–t response of a modified electrode recorded at 0.60 V with the successive addition of amounts of glucose; the inset shows the linear relationship between i and C of glucose [reprinted with permission from ref. 97, Copyright© Elsevier]. | |
In comparison to this, nitrogen-doped graphene with gold nanoparticles demonstrated a long linear range of 2 μM to 19.6 mM, with detection limit of 500 nM and high sensitivity of 0.9824 μA mM−1 cm−2 for glucose. The preparation of N–GR–CNTs/Au nanoparticles is presented schematically in Fig. 11. The catalytic activity of AuNPs seems to be well dispersed on the N–GR–CNTs network and has an interconnected network due to high charge transfer and high surface area which consequently improved the electrocatalytic activity.99
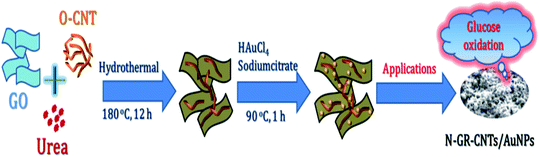 |
| Fig. 11 Schematic representation of the preparation of N–GR–CNTs/AuNPs nanohybrid [reprinted with permission from ref. 99, Copyright© Elsevier]. | |
2.2.6 N- and B-doped graphene-based sensors. Over the last decade, it has been reported that diverse electronic properties are demonstrated by graphene when doped with p- and n-type dopants.100,101 To date, various p- and n-type materials have been doped in graphene with different methods and employed for numerous applications.7,102–106 Still, few investigations have been reported regarding electrochemical properties and electrochemical probes. So, it is interesting to dope graphene materials with both p- and n-type dopants, as they would show different electrochemical nature as compared to that displayed by a single dopant.107 In this regard, Hui et al. successfully doped p (B)- and n (N)-type dopants in graphene in the presence of BF3 and NH3 as B and N precursors, respectively (Fig. 12). Differential pulse voltammograms were recorded for gallic acid oxidation on undoped (TRG), boron-doped (B–TRG) and nitrogen-doped (N–TRG) thermally reduced graphene materials. It has been demonstrated that boron-doped graphene delivers better electroanalytical results for the detection of gallic acid, generally employed as a standard probe for the quantification of antioxidant activity of foods and beverages.108
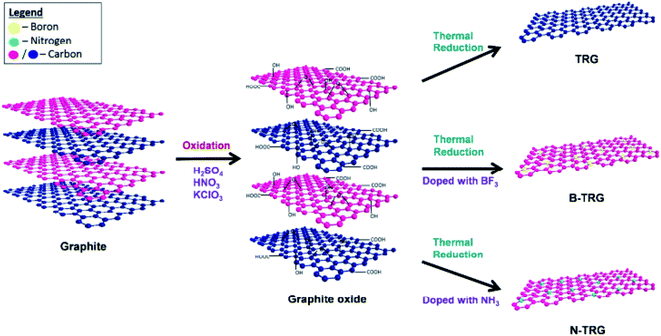 |
| Fig. 12 Schematic representation of the synthesis of undoped (TRG), boron-doped (B–TRG) and nitrogen-doped (N–TRG) thermally reduced graphene materials [reprinted with permission from ref. 108, Copyright© Royal Society of Chemistry]. | |
2.3 Silicon-doped graphene-based sensors
Other than the heteroatom doping discussed above, hypothetical study indicates that silicon doping can strikingly regulate electronic structure and enhance physical/chemical properties.109,110 Doping of silicon generates confined structural changes in both carbon nanotubes and graphene. It has been reported by Azadeh et al. that with the addition of silicon in graphene, a zero band gap was opened up.111 Chen and Niu illustrated that graphene doped with silicon is conceivably a notable candidate as a metal-free sensor for NO2.112,113 In another investigation, silicon-doped reduced GO was synthesized via thermal annealing method.114 Results obtained reveal that when Si–rGO is employed as electrocatalyst in counter electrodes in DSSCs, substantial improvement in electrocatalytic and electrochemical properties was noticed in comparison to pristine rGO (Fig. 13).
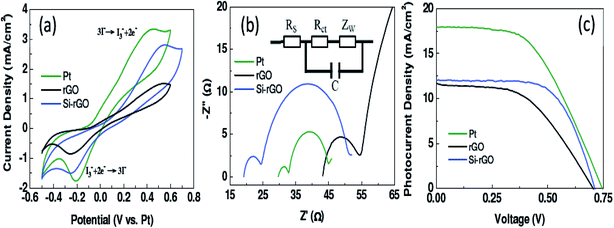 |
| Fig. 13 Electrochemical properties of Si–rGO, rGO and Pt based counter electrodes: CV (a), EIS (b) and photovoltage vs. photocurrent curves (c) [reprinted with permission from ref. 114, Copyright© Royal Society of Chemistry]. | |
Moreover, when Si–rGO was applied as active electrode in a supercapacitor, the conversion efficiency and specific capacity were enhanced by 29.6% and 48.5%, respectively. These studies displayed the outstanding properties of silicon-doped graphene, which will widen the range of applications of graphene-based materials.
2.3.1 Electrochemical sensors. A surface plasmon resonance biosensor employing graphene or MoS2 with silicon based on Kretschmann configuration has been reported. This sensor was observed to be highly selective and sensitive towards gold and silicon layers. The surface plasmon resonance biosensor is considered as an electrochemical sensor and the replacement of graphene by MoS2 leads to better reliability, good detection accuracy as well as best quality factor with minimum reflectance. This sensor was useful for detection of DNA with precision and accuracy.115 Verma et al.116 explored the adsorption theory of biomolecules using gold and graphene whose sensitivity is increased with the addition of silicon. Initially, the surface plasmon resonance was detected by using graphene and silicon layers coated on a prism having gold nanoparticles (Fig. 14). The best performance of sensor can be checked by its highly sensitive nature, the thickness of gold and silicon, and full width at half maximum.
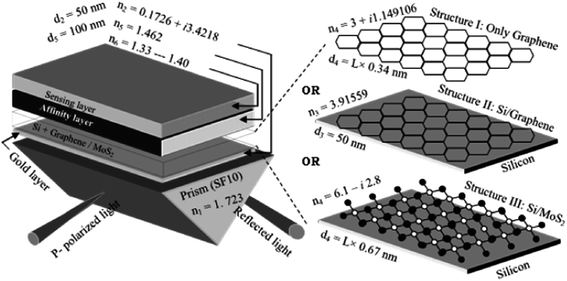 |
| Fig. 14 Schematic representation of three structures of surface plasmon resonance biosensor: (I) only graphene; (II) silicon/graphene; and (III) silicon/MoS2 [reprinted with permission from ref. 116, Copyright© Elsevier]. | |
2.3.2 Gas sensors. Gas sensors are small, low-cost and low-power-consumption devices for gas sensing. Gas sensors are classified in several ways such as catalytic gas sensors, electrochemical gas sensors, thermal conductivity gas sensors, optical gas sensors, and acoustic gas sensors which have physical and chemical advantages as well as disadvantages. Researchers have mainly focused on active layer fabrication by various techniques like synthesized sputtering, air-brushing, spin-coating, and spraying.117 Eom et al.118 proposed graphene-doped porous silicon substrates as hydrogen gas sensors. The interfacial sensing between the graphene and n-type silicon highlighted the sensing mechanism, an electrochemical etching process, and a capillary force-assisted dropping method. Graphene was doped with porous silicon and the resulting material was used as a sensor for optical and medical applications. Graphene nanosheets with palladium nanoparticles and porous silicon were investigated as hydrogen gas sensors.119 The hydrogen concentration shows linearity over response time where atomization plays an important role. This further gives fast and superior response compared to other hydrogen gas sensors. On the other hand, Zhu et al. explored different types of planar substrate and a textured substrate-based GO sensors. The textured substrate shows an excellent response and gives more molecular adsorption sites, favourable for sensing of NH3. Also, the textured substrate gave a uniform GO film, useful for gas sensing applications (Fig. 15).120 Furthermore, this textured GO film is suitable for polar oxygen configurations which intensified the ammonia response. For stress-based humidity sensors, nano-carbonaceous materials offer certain advantages over other polymer-like materials. For this, Yao et al. reported a GO silicon bi-layer flexible structure in which GO thin films were deposited onto silicon microbridge by spin-coating method. This structure exhibited high humidity sensitivity, good repeatability, small humidity hysteresis, and clear and fast response recovery over a wide relative humidity range of 10–98%.121
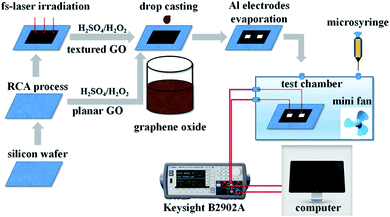 |
| Fig. 15 Schematic diagram of sensor preparation and gas sensing test system [reprinted with permission from ref. 120, Copyright© Elsevier]. | |
2.4 Phosphorus-doped graphene-based sensors
Phosphorus (P) atom has a pair of electrons just like nitrogen but its donor capacity is much higher than that of nitrogen. Hence, it is anticipated that phosphorus doping in graphene can deliver a better n-type nature in comparison to N doping. P can be doped into CNT/graphene, but it is basically different from N due to the presence of valence electrons in the third shell.122,123 Phosphorus, being larger in size than nitrogen, leads to more structural distortion on doping. P can form pyramidal-like bonding with carbon by converting sp2 hybridised C to sp3 state. Consequently, the P–C bond length is also increased by 24.6% as compared to the C–C bond length in pristine graphene. Also, the lower electronegativity of phosphorus than carbon makes the polarity of C–P bond exactly opposite to that of the C–N bond. However, in spite of certain constraints, Kim and co-workers were the first to fabricate P-doped graphene with n-type behaviour. The degree of doping and bonding configurations of doped graphene nanosheets were assessed via Raman spectroscopy, X-ray photoelectron spectroscopy and energy dispersive X-ray spectroscopy.124
2.4.1 Electrochemical sensors. Phosphorus-doped graphene (P–G) was used both as metal-free catalyst and metal catalyst support for electrochemical detection of dopamine (DA). As a metal-free catalyst, P–G shows important DA sensing property as the electrocatalytic activity of graphene towards DA oxidation is enhanced due to doping with P. It can be employed as a better support material for Au nanoparticle loading, with an excellent electrocatalytic activity and sensing applications. Wide linear range (0.1–180 μM) and low detection limit (0.002 μM) were revealed for Au/P–G hybrid which ensures its potential as a high-performance electrode material for electrochemical sensor applications (Fig. 16).125
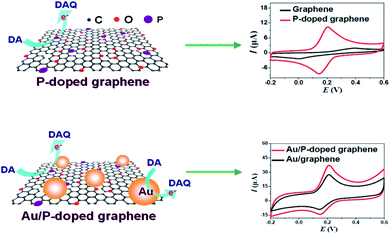 |
| Fig. 16 Structures of P-doped and Au/P-doped graphene and their CV responses [reprinted with permission from ref. 125, Copyright© Elsevier]. | |
The 3D phosphorus-doped graphene was explored for electrochemical sensing of H2O2. The results revealed excellent sensing property for hydrogen peroxide with wide linear range (0.0002–41.2 mM) and low detection limit (0.17 μM), and it can be employed for the detection of H2O2 even in living cells.126 Phosphorus-doped graphene-based sensors can also be used for the detection of acetaminophen (AP) in pharmaceuticals. An extraordinary electrocatalytic activity due to modified electrochemical conductivity and electron transfer was shown by a P–rGO-coated GCE. Low detection limit (0.36 μM), wide linear range (1.5–120 μM), enhanced selectivity, reproducibility and stability for AP were exhibited by this sensor.127
2.4.2 Gas sensors. The doping of more electron rich P into graphene leads to modification of the chemical properties of graphene to a large extent. Various research groups across the world have synthesized P-doped graphene and employed the material in different applications.123,128,129 Still, P-doped graphene/GO material has not been explored so much in the field of gas sensing. However, Niu and co-workers prepared P-doped graphene nanosheets for sensing of NH3 gas at room temperature (Fig. 17). The results obtained indicate that as-synthesized P-doped graphene nanosheets demonstrate better NH3 sensing capability, with improved response as compared to that of thermally synthesized rGO.130
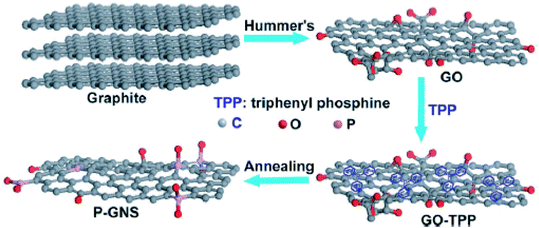 |
| Fig. 17 Schematic illustration of the synthesis of phosphorus-doped graphene nanosheets (P–GNS) through high-temperature annealing of graphene oxide and triphenylphosphine (GO–TPP) mixture [reprinted with permission from ref. 130, Copyright© Royal Society of Chemistry]. | |
2.5 Sulphur-doped graphene-based sensors
Modifying the electronic framework of graphene-based materials by doping could be a handy approach for appreciably refining materials for various applications.131 It will be intriguing to study the doping of a carbon material like graphene with an element having comparable electronegativity to that of carbon.132 Some research groups have successfully doped S in graphene-based materials and employed the same as a metal-free cathode catalyst for oxygen reduction.131,133 Similarly, Denis and co-workers134 reported that a curved carbon nanostructure (e.g. carbon nanotube) recommends S-doping more than flat graphene. S-doping on graphene involved two steps: formation of defect sites and SQS bond rupture. The resultant graphene sheet was more metallic than pristine graphene, making it a small-bandgap semiconductor.
2.5.1 Electrochemical sensors. An environment-friendly biomass precursor, lanthionine, was used for the preparation of S-doped reduced GO (S–rGO) which was further employed as an electrochemical sensor for identification of 8-hydroxy-2-deoxyguanosine (8-OH-2-dG) molecule (biomarker for cancer, oxidative stress). High electron-donating capability of sulphur enhanced the electrochemical sensing property of this sensor and shaped it as a good candidate for the detection of 8-OH-2-dG. The S-doping of about 2.28 atom% resulted in a sensitivity of 1 nM, and good stability, reproducibility and selectivity.135 Tian et al.136 used a microwave method for the consistent incorporation of Ag nanoparticles in sulphur-doped graphene (SG), and high S-doping (2.8 atom%) was observed in the fabricated Ag/SG hybrid. Enhanced electrocatalytic functioning for H2O2 reduction was observed because of doping which provided active sites and better charge transfer. This sensor exhibited better sensitivity, low detection limit (0.14 μM), superior selectivity and stable identification of H2O2 in electrochemical sensing. Fu et al.137 reported a SG-based electrochemical sensor and also a GCE, using 3,3,5,5-tetramethylbenzidine (TMB) composite (S–G–TMB) for detection of silver ions (Ag+). The S–G–TMB composite was fabricated and then tuned on the GCE surface which provided high selectivity and low detection limit (2.15 μM) for Ag+, due to the synergistic effect, good electrical conductivity of SG and coupling interactions between Ag and S. A porous sulphur-doped GO sensor was employed for the electrochemical detection of Hg(II) in which the porous structure and doping of S atoms have a crucial role. The porous S–rGO showed very low detection limit as compared to that exhibited by undoped porous rGO. The results obtained with this sensing platform were verified using atomic absorption spectroscopy. It was employed for decontamination of Hg(II) from drinking water, owing to surface and chemical features of the material.138
2.5.2 Gas sensors. Liang et al. reported an easy method for doping of S atoms in graphene by the introduction of a sulphur source at 1000 °C, to distinguish NO2 from other gases. The characterization results revealed successful doping of S atoms which formed honeycomb C–S compound crystal domains. It showed high selectivity sensing for NO2 as compared to NH3, CH4, SO2 and CO.139 But by combining S-doped graphene with micro-hot platform, very sensitive, quick response and fast recovery time sensors could be realized for NO2 as reported by Guo et al. After transportation of S onto a micro-hot platform, S-doping in graphene was achieved via chemical vapor deposition at 500 °C. This sensor showed sensitivity of 5 ppb for NO2 with fast recovery time (5 min).140
2.5.3 Biosensors. Most prominently, carbon-based sensors exhibit great potential in the field of electrochemical analysis.97,141,142 It has been revealed from the literature that graphene-based nanocomposites are very well established as biosensors. In this regard, Ren et al.143 reported the analysis of carcino-embryonic antigen (CEA) and nuclear matrix protein 22 (NMP22), employing a biosensing method in which matrix and label are GO/polyaniline nanostructures and mesoporous NKF-5-3, respectively. In this, click chemistry was used to fabricate sulphur-doped graphene sheets. The excellent detection of urine aliquots was attained with low detection limit of 25 fg mL−1 and 30 fg mL−1, respectively, for NMP22 and CEA.
2.6 N- and S-co-doped graphene-based sensors
Due to the presence of extensive π-electrons in the structure of graphene and graphene-doped materials, they are of interest for their electrocatalytic activity.144 The electrocatalytic activity of GO, N–GO, and NS–GO has been explored by Kumar and co-workers, employing potassium ferrocyanide as an electro-active probe molecule.145 The synthesized material was used as an electrochemical sensor for the detection of Eu(III) ions. The applicability in sensing was compared with that of single-doped graphene, and the results revealed better electrocatalytic activity of dual-doped graphene (LOD value of 5.92 μg L−1) than single-doped one.
2.6.1 Biosensors. A nitrogen–sulphur co-doped graphene (NS–G) with doping level of about 2.1 at% and 6.8 at% of S and N, respectively, was synthesized by a microwave-assisted solvothermal process. The N- and S-doped graphene exhibited enhanced electrocatalytic activity for H2O2 reduction as compared to that of undoped and mono-doped counterparts because of synergistic effect of N, S co-doping. NS–G was employed as an electrochemical sensor with low detection limit of 0.2 μM and 0.5 μM for H2O2 and glucose, respectively.146 S–rGO/CuS nanocomposite was fabricated by the simple but highly efficient sonochemical method and was used as a glucose sensor electrode (Fig. 18). This electrode was employed to determine glucose over a wide linear concentration range (0.0001–3.88 mM and 3.88–20.17 mM), along with quite low detection limit of 32 nM, with good stability and selectivity. The electrode was used practically for sensing of glucose in real life biological samples such as human saliva, urine and blood serum.147 N- and S-co-doped graphene materials have been widely used for the fabrication of sensors, to target various materials as summarized in Table 1, in terms of their linear detection range and low LOD.123,133
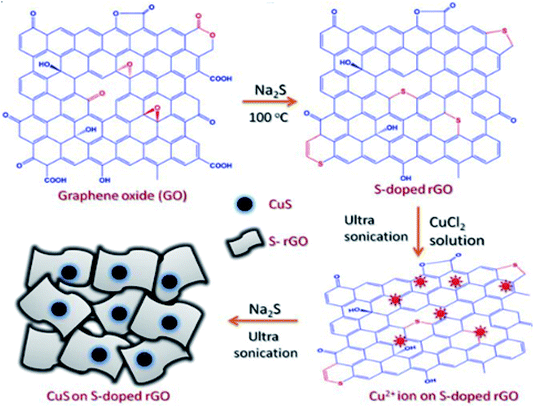 |
| Fig. 18 Schematic representation of sonochemical fabrication of S–rGO/CuS [reprinted with permission from ref. 147, Copyright© Scientific Reports]. | |
Table 1 Sensors based on N- and S-co-doped graphene nanocomposites for different target materials
Graphene-based nanocomposite |
Target material |
Linear range |
Limit of detection (LOD) |
Ref. |
N-doped GQDs |
Al3+ |
2.5–75 μM |
1.3 μM |
148 |
S-doped GQDs |
Ag+ |
0.1–130 μM |
30 nM |
149 |
N-doped GQDs |
Hg2+ |
2–200 nM |
0.32 nM |
150 |
N-doped GQDs |
Omethoate |
0.1–1.7 nM |
0.041 pM |
151 |
N-doped GQDs MIP |
Thiacloprid |
0.1–10 mg L−1 |
0.03 mg L−1 |
152 |
N,S–rGO |
DNA |
5–100 nM |
2.4 nM |
153 |
S-doped GQDs |
Fe3+ |
0–0.7 mM |
4.2 nM |
154 |
N-(Aminobutyl)-N-(ethylisoluminol) (ABEI)-functionalized GQDs |
Flubendiamide |
|
10 ng mL−1 |
155 |
N/S–GQD |
Ethion |
19.2–961.2 μg L−1 |
8 μg L−1 |
156 |
N–GQD@V2O5 |
Cysteine |
0–125 μM |
50 nM |
157 |
AuNPs@S–GQD |
4-Nitrophenol |
0.005–50 μM |
3.5 nM |
158 |
2.7 Halogen-doped sensors
Halogen atoms display both electron-withdrawing and resonance donating effects due to high electronegativity and the presence of a lone pair of electrons, respectively. On the basis of the type of halogen atom, the electronic structure of graphene changes remarkably. Fluorinated graphene has a wide band gap (3.1 eV) whereas chlorinated graphene has very small band gap of 0.9 eV, and brominated graphene has nearly zero band gap. Experimental and theoretical study reveals that with growing size of halogen atom or diminishing electronegativity, the electric resistivity of halogen-doped graphene declines.159 Halogen-doped graphene illustrates speedy heterogenous electron transfer for Cl-doped graphene and steady for I-doped graphene in terms of electrochemical properties.160
2.7.1 Iodine-doped sensors. Halogen doping in graphene has attracted increasing consideration in recent years.161 The literature shows that the doping of halogens can considerably enhance the catalytic properties of graphene materials because halogen dopants are able to alter the electronic structure as well as hybridisation of graphene, simultaneously.162,163 Among the halogens, fluorine (F) has revealed exceptional dynamics for graphene doping. The doping of F can give extreme charge polarization of adjoining C atoms, for improving the electrocatalytic action.164 Ongoing research has demonstrated that other than F, iodine (I) doping can likewise notably advance the application of graphene, in the light of the fact that geometry and electronic structures of graphene can change remarkably.165,166 The experimental scheme for preparation of I-doped graphene composite is shown in Fig. 19.
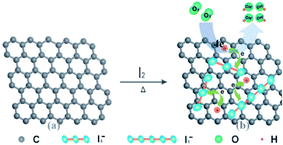 |
| Fig. 19 Schematic illustration of I-doped graphene preparation [reprinted with permission from ref. 166, Copyright© Royal Society of Chemistry]. | |
Jeon and co-workers established ORR activity with the doping of three types of halogens, i.e. Cl, Br and I, in graphene. It was found that I-doped graphene as a metal-free electrocatalyst displayed high ORR activity as compared to Cl- or Br-doped graphene and industrial Pt/C catalyst.166 Similarly, in another investigation, it was proved that I-doped graphene exhibited better electrochemical properties than pristine graphene.167 In case of sensing, Chu and co-workers reported a one-step and low-temperature approach for the synthesis of iodine-doped graphene, and employed the material in HER and electrochemical sensing (Fig. 19). The I-doped graphene material showed a low overpotential of 245 mV at 10 mA cm−2 and a small Tafel slope of 96 mV dec−1, which is superior to that of pristine graphene, when applied in metal-free HER. Further, the as-prepared material was also used for the fabrication of an electrochemical sensor for sensing of DA. This sensor showed exceptional selectivity with a linear range of 0.1–130.5 mM, and a low detection limit of 0.05 μM.168
2.7.2 Chlorine-doped sensors. A green and effective preparation method for chlorine-doped reduced GO (Cl–rGO) was established by Wang and co-workers.169 The Cl–rGO material was further utilized as an electrochemical sensor for the detection of chloramphenicol (CAP), a veterinary drug in milk, calf plasma, water and pharmaceutical samples. The sensor showed a linear relation between current intensity and CAP concentration (2–35 μM), with a detection limit of 1 μM. The sensor based on Cl–rGO material also showed exceptional reproducibility and storage stability.
2.7.3 Bromine-doped sensors. A novel low-cost electrochemical sensor was synthesized using bromine (Br)-doped graphene (GBR) coated GCE. This electrode exhibited excellent CV response towards hydrogen peroxide (H2O2).170 Calibration curves for H2O2 detection employing CV and DPV techniques were designed with 0.1–10 mM linearity range and with LOD of 0.048 and 0.063 mM, respectively. Being highly selective and specific, its activity is also retained for a long time due to which it can be used as a substitute for natural enzymes.
2.7.4 Fluorine-doped sensors. Amongst the various doped graphene-based nanomaterials, F-doped materials are of considerable importance as a result of their remarkable properties, for example, high-temperature resistance and improved electrocatalytic activity.171 The structural changes of single-layer graphene during the fluorination process were investigated by transmission electron micrographs (Fig. 20).
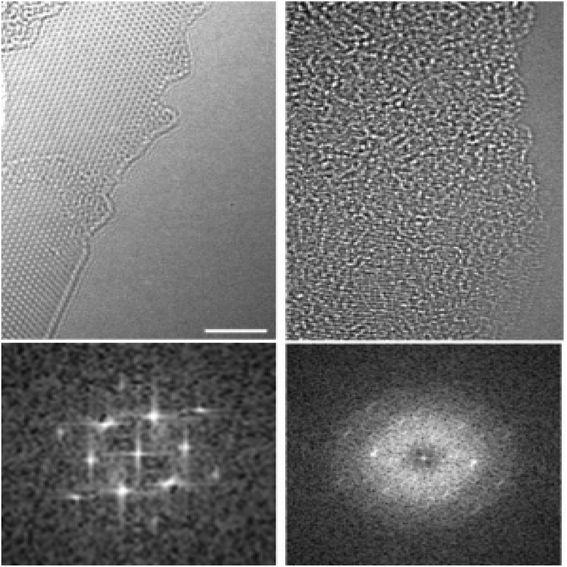 |
| Fig. 20 Atomic-resolution TEM micrographs of single-layer graphene and fluorographene at edges and corresponding digital diffractograms [reprinted with permission from ref. 171, Copyright© American Chemical Society]. | |
Being a highly electronegative element, fluorine may lead to diverse bonding characteristics, like ionic, semi-ionic and covalent, with the relatively less electronegative carbon of graphene.172 Moreover, F doping may cause appreciable change in the electronic structure of graphene, due to the strong electron-withdrawing nature of F and electron-donating nature of lone pair of electrons.173 Consequently, various research groups have widely employed fluorinated graphene in a variety of applications.174–178 In addition to these applications, F-doped GO has also been used in sensing. For example, Park and co-workers fabricated F-doped GO (F–GO)/SiO2/Si composite material for sensing of ammonia gas. The as-prepared sensor exhibits nearly 7% change in resistive response, while undoped GO does not show any sensing behaviour towards NH3 gas.179 In another study, one-pot synthesis of fluorinated GO (FGO) for electrochemical sensing of heavy metal ions has been reported.180 The FGO material was successfully applied as a sensor for the simultaneous detection of Hg2+, Cu2+, Pb2+ and Cd2+ions, using square wave anodic stripping voltammetry (SWASV). Sensitivity for the simultaneous detection of Hg2+, Cu2+, Pb2+ and Cd2+ions was found to be 4.24, 3.64, 6.05 and 3.64 μA μM−1, respectively (Fig. 21).
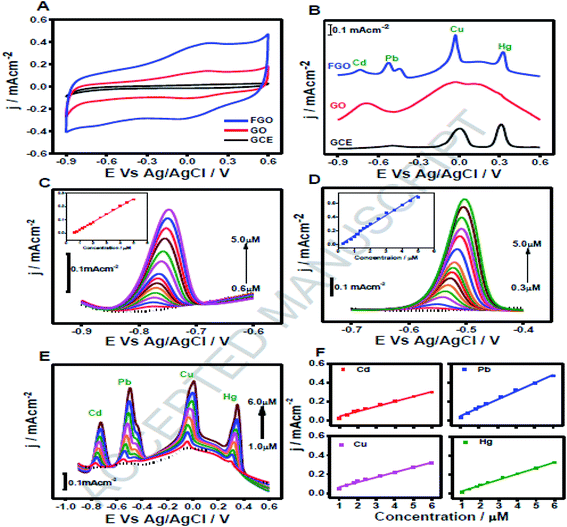 |
| Fig. 21 (A) CVs of GO and FGO modified GCE in acetate buffer. (B) SWASVs for 2 μM analytes each of Cd(II), Pb(II), Cu(II), Hg(II) on FGO (blue line), GO (red line) and GCE (black line). (C) SWASVs of FGO for Cd(II) sensing; inset shows the calibration curve. (D) SWASVs of FGO for Pb(II) sensing; inset shows the corresponding calibration curve. (E) SWASVs of FGO for the simultaneous sensing of Cd(II), Pb(II), Cu(II), Hg(II). (F) Calibration plots for the electrochemical detection of Cd(II), Pb(II), Cu(II) and Hg(II) ions [reprinted with permission from ref. 180, Copyright© Elsevier]. | |
Recently, Manikandan et al. fabricated F-doped GO-based sensor for the detection of caffeic acid (CA) in wine.181 For critical quantification of CA, the DPV technique was used and the sensor generated a stable oxidation signal in 0.5 to 100.0 μM concentration range of CA with LOD of 0.018 μM.
3. Conclusion and future perspectives
In this article, noteworthy advances made in the development of new sensor devices using graphene-based materials that include pristine graphene, GO, reduced GO and GQDs have been reviewed. The electronic structure of graphene offers tremendous optical, electrochemical and mechanical properties. The best outcome was found in terms of affectability, linear concentration range, and detection limit of sensors. These kinds of sensors are well suited for detecting metal ions in various natural situations or human serum tests. There are a variety of heteroatoms like N, P, S, Si, Cl, Br, and I which can be doped in graphene or GO. Doping of these graphene-based materials with different heteroatoms can significantly influence their electromagnetic, optical and structural behaviour. These changes enhance the ability of graphene, GO and rGO to detect a wide range of analytes, thus imparting remarkable performance to the sensors. Heteroatom doping is achieved by a variety of approaches. The type of dopant, bonds formed, reaction time, temperature etc. also play a crucial role in fabricating better and promising materials. These new doped materials have attracted much attention for their utilization in numerous sensing applications. This article is particularly focused on reviewing the progress and accomplishment of doped graphene materials for sensing which is at present picking up pace. In spite of these impressive advancements, there is still a challenge to carefully control the doping of heteroatoms, due to huge and uncontrolled heterogeneity of the materials and the nature of bonding arrangements of dopants for the in-depth understanding of structure–property relationships of different graphene materials. This is because the dopant is not fully integrated into the lattice and is more commonly present on it in the form of electron withdrawing or donating group which needs to be accessed. This article has surveyed different dopants for emerging sensing applications, and there is a further need for co-doping or multi-doping of heteroatoms in their appropriate relative ratios and compositions that will selectively enhance the distinct properties of graphene. So, the new hopes provided by heteroatom doping will enable graphene to flourish even more in the near future and inspire more applications of this evolving group of nanomaterials.
Regardless of a lot of work done on sensors, there is still a huge scope for further improvement. There is an urgent necessity to carry out more research on graphene as there are a lot more findings that need to be explored.
Conflicts of interest
There are no conflicts of interest to declare.
References
- A. Madni, S. Noreen, I. Maqbool, F. Rehman, A. Batool, P. M. Kashif, M. Rehman, N. Tahir and M. I. Khan, Graphene-based nanocomposite, synthesis and their theranostic applications, J. Drug Targeting, 2018, 10, 858–883 CrossRef PubMed
. - M. F. Craciun, I. Khrapach, M. D. Barnes and S. Russo, Properties and applications of chemically functionalized graphene, J. Phys.: Condens. Matter, 2013, 25, 1–22 CrossRef PubMed
. - Y. Zhu, S. Murali, W. Cai, X. Li, J. W. Suk, J. R. Potts and R. S. Ruoff, Graphene and Graphene Oxide: Synthesis, Properties, and Applications, Adv. Mater., 2010, 22, 3906–3924 CrossRef CAS PubMed
. - T. C. Dinadayalane, D. Leszczynska and J. Leszczynski, Graphene: Properties, Biomedical Applications and Toxicity, Chapter 1, J. Nanosci. Nanotechnol., 2012, 1–26 Search PubMed
. - Nanomaterial-Based Flexible and Multifunction Sensors, ed. E. Singh and H. S. Nalwa, American Scientific Publishers, Los Angeles, CA, 2019 Search PubMed
. - B. Qiu, M. Xing and J. Zhang, Recent advances in three-dimensional graphene-based materials for catalysis applications, Chem. Soc. Rev., 2018, 47, 2165–2216 RSC
. - M. Pumera, Heteroatom modified graphenes, electronic and electrochemical applications, J. Mater. Chem. C, 2014, 2, 6454–6461 RSC
. - J. Duan, S. Chen, M. Jaroniec and S. Z. Qiao, Heteroatom-doped graphene-based materials for energy-relevant electrocatalytic processes, ACS Catal., 2015, 5, 5207–5234 CrossRef CAS
. - J. Wu, W. Pisula and K. Müllen, Graphenes as potential material for electronics, Chem. Rev., 2007, 107, 718–747 CrossRef CAS PubMed
. - M. D. Stoller, S. Park, Y. Zhu, J. An and R. S. Ruoff, Graphene-based ultracapacitors, Nano Lett., 2008, 8, 3498–3502 CrossRef CAS PubMed
. - N. G. Sahoo, Y. Pan, L. Li and S. H. Chan, Graphene-based materials for energy conversion, Adv. Mater., 2012, 24, 4203–4210 CrossRef CAS PubMed
. - P. R. Nair and M. A. Alam, Screening-limited response of nano biosensors, Nano Lett., 2008, 8, 4373–4379 CrossRef PubMed
. - J. C. Byers, A. G. Güell and P. R. Unwin, Nanoscale electrocatalysis: visualizing oxygen reduction at pristine, kinked, and oxidized sites on individual carbon nanotubes, J. Am. Chem. Soc., 2014, 136, 11252–11255 CrossRef CAS PubMed
. - K. A. Ritter and J. W. Lyding, The influence of edge structure on the electronic properties of graphene quantum dots and nanoribbons, Nat. Mater., 2009, 8, 235–242 CrossRef CAS PubMed
. - X. Yan, X. Cui and L. Li, Synthesis of large, stable colloidal graphene quantum dots with tunable size, J. Am. Chem. Soc., 2010, 132(17), 5944–5945 CrossRef CAS PubMed
. - H. Wang, T. Maiyalagan and X. Wang, Review on recent progress in nitrogen-doped graphene: synthesis, characterization, and its potential applications, ACS Catal., 2012, 2, 781–794 CrossRef CAS
. - V. Georgakilas, M. Otyepka, A. B. Bourlinos, V. Chandra, N. Kim, K. C. Kemp, P. Hobza, R. Zboril and K. S. Kim, Functionalization of graphene: covalent and non-covalent approaches, derivatives and applications, Chem. Rev., 2012, 112, 6156–6214 CrossRef CAS PubMed
. - C. I. L. Justino, A. R. Gomes, A. C. Freitas, A. C. Duarte and T. Santos, Graphene based sensors and biosensors, Trends Anal. Chem., 2017, 91, 53–66 CrossRef CAS
. - G. Chen, Y. Liu, Y. Liu, Y. Tian and X. Zhang, Nitrogen and sulfur dual-doped graphene for glucose biosensor application, J. Electroanal. Chem., 2015, 738, 100–107 CrossRef CAS
. - R. Ma, Y. Ma, Y. Dong and J. M. Lee, Recent advances in heteroatom-doped graphene materials as efficient electrocatalysts towards the oxygen reduction reaction, Nano Adv., 2016, 1, 50–61 CrossRef
. - S. Agnoli and M. Favaro, Doping graphene with boron: a review of synthesis methods, physicochemical characterization and emerging applications, J. Mater. Chem. A, 2016, 4, 5002–5025 RSC
. - C. I. L. Justino, T. Santos and A. C. Duarte, Review of analytical figures of merit of sensors and biosensors in clinical applications, Trends Anal. Chem., 2010, 29, 1172–1183 CrossRef CAS
. - C. I. L. Justino, T. Santos and A. C. Duarte, Review of analytical figures of merit of sensors and biosensors in clinical applications, Trends Anal. Chem., 2010, 29, 1172–1183 CrossRef CAS
. - S. Basu and S. K. Hazra, Graphene–Noble Metal Nano-Composites and Applications for Hydrogen Sensors, C, 2017, 3, 1–15 Search PubMed
. - Y. B. Tang, L. C. Yin, Y. Yang, X. H. Bo, Y. L. Cao, H. E. Wang, W. J. Zhang, I. Bello, S. T. Lee, H. M. Cheng and C. S. Lee, Tunable Band Gaps and p-Type Transport Properties of Boron-Doped Graphenes by Controllable Ion Doping Using Reactive Microwave Plasma, ACS Nano, 2012, 6, 1970–1978 CrossRef CAS PubMed
. - R. Faccio, L. F. Werner, H. Pardo, C. Goyenola, O. N. Ventura and Á. W. Mombrú, Electronic and Structural Distortions in Graphene Induced by Carbon Vacancies and Boron Doping, J. Phys. Chem. C, 2014, 114, 18961–18971 CrossRef
. - X. Li, L. Fan, Z. Li, K. Wang, M. Zhong, J. Wei, D. Wu and H. Zhu, Boron Doping of Graphene for Graphene–Silicon p–n Junction Solar Cells, Adv. Energy Mater., 2012, 2, 425–429 CrossRef CAS
. - S. Yu, W. Zheng, C. Wang and Q. Jiang, Nitrogen/Boron Doping Position Dependence of the Electronic Properties of a Triangular Graphene, ACS Nano, 2010, 4(12), 7619–7629 CrossRef CAS PubMed
. - M. Singh, S. Kaushal, P. Singh and J. Sharma, Boron doped graphene oxide with enhanced photocatalytic activity for organic pollutants, J. Photochem. Photobiol., A, 2018, 364, 130–139 CrossRef CAS
. - C. Dou, S. Saito, K. Matsuo, I. Hisaki and S. Yamaguchi, A Boron-Containing PAH as a Substructure of Boron-Doped Graphene, Angew. Chem., Int. Ed., 2012, 51, 12206–12210 CrossRef CAS PubMed
. - X. Wang, G. Sun, P. Routh, D. H. Kim, W. Huang and P. Chen, Heteratom-doped graphene materials: syntheses, properties and applications, Chem. Soc. Rev., 2014, 43, 7067–7098 RSC
. - J. Borowiec and J. Zhang, Hydrothermal Synthesis of Boron-Doped Graphene for Electrochemical Sensing of Guanine, J. Electrochem. Soc., 2015, 162, 332–336 CrossRef
. - B. R. Sathe, X. Zou and T. Asefa, Metal-free B-doped graphene with efficient electrocatalytic activity for hydrogen evolution reaction, Catal. Sci. Technol., 2014, 4, 2023–2030 RSC
. - M. H. Ghanbari, Z. Norouzi and M. M Ghanbari, Using a nanocomposite consist of boron-doped reduced graphene oxide and electropolymerized β-cyclodextrin for flunitrazepam electrochemical sensor, Microchem, 104994, 156, 2020 Search PubMed
. - K. S. Hwang, M. H. Lee, J. Lee, W. S. Yeo, J. H. Lee, K. M. Kim, J. Y. Kang and T. S. Kim, Peptide receptor-based selective dinitrotoluene detection using a microcantilever sensor, Biosens. Bioelectron., 2011, 30, 249–254 CrossRef CAS PubMed
. - C. R. Carmona, O. Malinkiewicz, A. Soriano, G. M. Espallargas, A. Garcia, P. Reinecke, T. Kroyer, M. I. Dar, M. K. Nazeeruddin and H. J. Bolink, Flexible high efficiency perovskite solar cells, Energy Environ. Sci., 2014, 7, 994–997 RSC
. - K. Lee, Y. K. Yoo, M. S. Chae, K. S. Hwang, J. Lee, H. Kim, D. Hur and J. H. Lee, Highly selective reduced graphene oxide (rGO) sensor based on a peptide aptamer receptor for detecting explosives, Sci. Rep., 2019, 9, 1–9 CrossRef PubMed
. - J. Zhang and E. P. Fahrenthold, Graphene-Based Sensing of Gas-Phase Explosives, ACS Appl. Nano Mater., 2019, 2, 1445–1456 CrossRef CAS
. - Q. Hao, X. Xia, W. Lei, W. Wang and J. Qiu, Facile synthesis of sandwich-like polyaniline/boron-doped graphene nano hybrid for supercapacitors, Carbon, 2015, 81, 552–563 CrossRef CAS
. - J. Znu, G. He, Z. Tian, L. Liang and P. K. Shen, Facile synthesis of boron and nitrogen-dual-doped graphene sheets anchored platinum nanoparticles for oxygen reduction reaction, Electrochim. Acta, 2016, 194, 276–282 CrossRef
. - Y. Xu, W. Lei, Z. Han, T. Wang, M. Xia and Q. Hao, Boron-doped graphene for fast electrochemical detection of HMX explosive, Electrochim. Acta, 2016, 216, 219–227 CrossRef CAS
. - B. Huang, Z. Li, Z. Liu, G. Zhou, S. Hao, J. Wu, B. L. Gu and W. Duan, Adsorption of Gas Molecules on Graphene Nanoribbons and Its Implication for Nanoscale Molecule Sensor, J. Phys. Chem. C, 2008, 112, 13442–13446 CrossRef CAS
. - W. Li, L. S. Zhang, Q. Wang, Y. Yu, Z. Chen, C. Y. Cao and W. G. Song, Low-cost synthesis of graphitic carbon nanofibers as excellent room temperature sensors for explosive gases, J. Mater. Chem., 2012, 22, 15342–15347 RSC
. - Y. Zou, F. Li, Z. H. Zhu, M. W. Zhao, X. G. Xu and X. Y. Su, An ab initio study on gas sensing properties of graphene and Si-doped graphene, Eur. Phys. J. B, 2011, 81, 475–479 CrossRef CAS
. - Y. Tang, Z. Liu, X. Dai, Z. Yang and Z. Lu, Theoretical study on the Si-doped graphene as an efficient metal-free catalyst for CO oxidation, Appl. Surf. Sci., 2014, 308, 402–407 CrossRef CAS
. - O. Leenaerts, B. Partoens and F. M. Peeters, Adsorption of H2O, NH3, CO, NO2 and NO graphene: a first principle study, Phys. Rev. B: Condens. Matter Mater. Phys., 2008, 77(12), 125416–125424 CrossRef
. - R. Lv, G. Chen, Q. Li, A. McCreary, A. B. Mendez, S. V. Morozov, L. Liang, X. Declerck, N. P. Lopez, D. A. Cullen, S. Feng, A. L. Elias, R. C. Silva, K. Fujisawa, M. Endo, F. Kang, J. C. Charlier, V. Meunier, M. Pan, A. R. Harutyunyan, K. S. Novoselov and M. Terrones, Ultrasensitive gas detection of large-area boron-doped graphene, Proc. Natl. Acad. Sci. U. S. A., 2015, 112, 14527–14532 CrossRef CAS PubMed
. - S. S. Varghese, S. Swaminathan and K. K. Singh, Ab initio study on gas sensing properties of group III (B, Al and Ga) doped graphene, Comput. Condens. Matter, 2016, 9, 40–55 CrossRef
. - Y. Zhang, J. Zhao, H. Sun, Z. Zhu, J. Zhang and Q. Liu, B, N, S, Cl doped graphene quantum dots and their effects on gas-sensing properties of Ag–LaFeO3, Sens. Actuators, B, 2018, 266, 364–374 CrossRef CAS
. - R. Peng, Y. Li, T. Liu, P. Si, J. Feng, J. Suhr and L. Ci, Boron-doped graphene coated Au@SnO2 for high-performance triethylamine gas detection, Mater. Chem. Phys., 2020, 239, 121961 CrossRef CAS
. - S. Srivastava, S. K. Jain, G. Gupta, T. D. Senguttuvan and B. K. Gupta, Boron-doped few-layer graphene nanosheet gas sensor for enhanced ammonia sensing at room temperature, RSC Adv., 2020, 10, 1007–1014 RSC
. - M. A. Mannan, Y. Hirano, A. T. Quitain, M. Koinuma and T. Kida, Boron doped graphene oxide: synthesis and application to glucose responsive reactivity, J. Mater. Sci. Eng., 2018, 7, 1–6 Search PubMed
. - L. Zhang, Z. Y. Zhang, R. P. Liang, Y. H. Li and J. D. Qiu, Boron-Doped Graphene Quantum Dots for Selective Glucose Sensing Based on the “Abnormal” Aggregation-Induced Photoluminescence Enhancement, Anal. Chem., 2014, 86, 4423–4430 CrossRef CAS PubMed
. - X. Wang, X. Li, L. Zhang, Y. Yoon, P. K. Weber, H. Wang, J. Guo and H. Dai, N-doping of graphene through electrothermal reactions with ammonia, Science, 2009, 324, 768–771 CrossRef CAS PubMed
. - Z. Sun, Z. Yan, J. Yao, E. Beitler, Y. Zhu and J. M. Tour, Growth of graphene from solid carbon sources, Nature, 2010, 468, 549–552 CrossRef CAS PubMed
. - Z. Jin, J. Yao, C. Kittrell and J. M. Tour, Large Scale Growth and Characterizations of Nitrogen-Doped Monolayer Graphene Sheets, ACS Nano, 2011, 5, 4112–4117 CrossRef CAS PubMed
. - M. P. Kumar, T. Kesavan, G. Kalita, P. Ragupathy, T. N. Narayanan and D. K. Pattanayak, On the large capacitance of nitrogen doped graphene derived by a facile route, RSC Adv., 2014, 4, 38689–38697 RSC
. - H. Zhang, T. Kuila, N. H. Kim, D. S. Yu and J. H. Lee, Simultaneous reduction, exfoliation, and nitrogen doping of graphene oxide via a hydrothermal reaction for energy storage electrode materials, Carbon, 2014, 69, 66–78 CrossRef CAS
. - Y. Li, Z. Zhou, P. Shen and Z. Chen, Spin Gapless Semiconductor–Metal–Half-Metal Properties in Nitrogen-Doped Zigzag Graphene Nanoribbons, ACS Nano, 2009, 3, 1952–1958 CrossRef CAS PubMed
. - L. Sun, L. Wang, C. Tian, T. Tan, Y. Xie, K. Shi, M. Li and H. Fu, Nitrogen-doped graphene with nitrogen level via a one-step hydrothermal reaction of graphene oxide with urea for superior capacitive energy storage, RSC Adv., 2012, 2, 4498–4506 RSC
. - Y. H. Lee, K. H. Chang and C. C. Hu, Differentiate the pseudocapacitance and double-layer capacitance contributions for nitrogen-doped reduced graphene oxide in acidic and alkaline electrolytes, J. Power Sources, 2013, 227, 300–308 CrossRef CAS
. - A. Śliwak, B. Grzyb, N. Díez and G. Gryglewicz, Nitrogen-doped reduced graphene oxide as electrode material for high rate supercapacitors, Appl. Surf. Sci., 2017, 399, 265–271 CrossRef
. - D. Usachov, O. Vilkov, A. Grüneis, D. Haberer, A. Fedorov, V. K. Adamchuk, A. B. Preobrajenski, P. Dudin, A. Barinov, M. Oehzelt, C. Laubschat and D. V. Vyalikh, Nitrogen-Doped Graphene: Efficient Growth, Structure, and Electronic Properties, Nano Lett., 2011, 11, 5401–5407 CrossRef CAS PubMed
. - Y. Shao, J. Wang, H. X. Wu, J. J. Liu, I. A. Aksay and Y. Lin, Graphene Based Electrochemical Sensors and Biosensors: A Review, Electroanalysis, 2010, 22, 1027–1036 CrossRef CAS
. - Y. S. Wu, Z. T. Liu, T. P. Wang, S. Y. Hsu and C. L. Lee, A comparison of nitrogen-doped sonoelectrochemical and chemical graphene nanosheets as hydrogen peroxide sensors, Ultrason. Sonochem., 2018, 42, 659–664 CrossRef CAS PubMed
. - M. Kaur, M. Kaur and V. K. Sharma, Nitrogen-doped graphene and graphene quantum dots: a review on synthesis and applications in energy, sensors and environment, Adv. Colloid Interface Sci., 2018, 259, 44–64 CrossRef CAS PubMed
. - M. Megawati, C. K. Chua, Z. Sofer, K. Klímová and M. Pumera, Nitrogen-doped graphene: effect of graphite oxide precursors and nitrogen content on the electrochemical sensing properties, Phys. Chem. Chem. Phys., 2017, 19, 15914–15923 RSC
. - A. Mohammadian, M. Ebrahimi and H. K. Maleh, Synergic effect of 2D nitrogen doped reduced graphene nano-sheet and ionic liquid as a new approach for fabrication of anticancer drug sensor in analysis of doxorubicin and topotecan, J. Mol. Liq., 2018, 265, 727–732 CrossRef CAS
. - X. Li, H. Zhao, L. Shi, X. Zhu, M. Lan, Q. Zhang and Z. H. Fan, Electrochemical sensing of nicotine using screen-printed carbon electrodes modified with nitrogen-doped graphene sheets, J. Electroanal. Chem., 2017, 784, 77–84 CrossRef CAS
. - S. Mutyala and J. Mathiyarasu, A highly sensitive NADH biosensor using nitrogen doped graphene modified electrodes, J. Electroanal. Chem., 2016, 775, 329–336 CrossRef CAS
. - H. Xu, J. Xiao, L. Yan, L. Zhu and B. Liu, An electrochemical sensor for selective detection of dopamine based on nickel tetrasulfonated phthalocyanine functionalized nitrogen-doped graphene nanocomposites, J. Electroanal. Chem., 2016, 779, 92–98 CrossRef CAS
. - W. Si, W. Lei, Q. Hao, X. Xia, H. Zhang, J. Li, Q. Li and R. Cong, Facile Synthesis of Nitrogen-Doped Graphene Derived from Graphene Oxide and Vitamin B3 as High-Performance Sensor for Imidacloprid Determination, Electrochim. Acta, 2016, 212, 784–790 CrossRef CAS
. - Z. X. Cai, X. H. Song, Y. Y. Chen, Y. R. Wang and X. Chen, 3D nitrogen-doped graphene aerogel: a low-cost, facile prepared direct electrode for H2O2 sensing, Sens. Actuators, B, 2016, 222, 567–573 CrossRef CAS
. - Y. Y. Shao, S. Zhang, M. H. Engelhard, G. S. Li, G. C. Shao, Y. Wang, J. Liu, I. A. Aksay and Y. H. Lin, Nitrogen-doped graphene and its electrochemical applications, J. Mater. Chem., 2010, 20, 7491–7496 RSC
. - P. Wu, Y. D. Qian, P. Du, H. Zhang and C. X. Cai, Facile synthesis of nitrogen-doped graphene for measuring the releasing process of hydrogen peroxide from living cells, J. Mater. Chem., 2012, 22, 6402–6412 RSC
. - F. Yang, W. Bao, T. Liu, B. Zhang, S. Huang, W. Yang, Y. Li, N. Li, C. Wang, C. Pan and Y. Li, Nitrogen-doped graphene quantum dots prepared by electrolysis of nitrogen-doped nanomesh graphene for the fluorometric determination of ferric ions, Microchim. Acta, 2020, 187, 322 CrossRef CAS PubMed
. - S. A. Bhat, N. Rashid, M. A. Rather, S. A. Pandit, P. P. Ingole and M. A. Bhat, Vitamin B12 functionalized N-doped graphene: a promising electro-catalyst For hydrogen evolution and electro-oxidative sensing of H2O2, Electrochim. Acta, 2020, 135730 CrossRef CAS
. - S. S. Varghese, S. Lonkar, K. K. Singh, S. Swaminathan and A. Abdala, Recent advances in graphene based gas sensors, Sens. Actuators, B, 2015, 218, 160–183 CrossRef CAS
. - J. J. Adjizian, R. Leghrib, A. A. Koos, I. S. Martinez, A. Crossley, P. Wagner, N. Grobert, E. Llobet and C. P. Ewels, Boron- and nitrogen-doped multi-wall carbon nanotubes for gas detection, Carbon, 2014, 66, 662–673 CrossRef CAS
. - C. C. Ma, X. H. Shao and D. P. Cao, Nitrogen-doped graphene as an excellent candidate for selective gas sensing, Sci. China: Chem., 2014, 57, 911–917 CrossRef CAS
. - H. Peng, F. Li, Z. Hua, K. Yang, F. Yin and W. Yuan, Highly sensitive and selective room-temperature nitrogen dioxide sensors based on porous graphene, Sens. Actuators, B, 2018, 275, 78–85 CrossRef CAS
. - Z. Wang, C. Zhao, T. Han, Y. Zhang, S. Liu, T. Fei, G. Lu and T. Zhang, High-performance reduced graphene oxide-based room-temperature NO2 sensors: a combined surface modification of SnO2 nanoparticles and nitrogen doping approach, Sens. Actuators, B, 2017, 242, 269–279 CrossRef CAS
. - A. R. Cadore, E. Mania, A. B. Alencar, N. P. Rezende, S. de Oliveira, K. Watanabe, T. Taniguchi, H. Chacham, L. C. Campos and R. G. Lacerda, Enhancing the response of NH3 graphene-sensors by using devices with different graphene-substrate distances, Sens. Actuators, B, 2018, 266, 438–446 CrossRef CAS
. - X. Feng, Y. Zhang, J. Zhou, Y. Li, S. Chen, L. Zhang, Y. Ma, L. Wang and X. Yan, Three-dimensional nitrogen-doped graphene as an ultrasensitive electrochemical sensor for the detection of dopamine, Nanoscale, 2015, 7, 2427–2432 RSC
. - W. Lei, W. Si, Q. Hao, Z. Han, Y. Zhang and M. Xia, Nitrogen-doped graphene modified electrode for nimodipine sensing, Sens. Actuators, B, 2015, 212, 207–213 CrossRef CAS
. - C. Zhang, L. Wang, A. Wang, S. Zhang, C. Mao, J. Song, H. Niu, B. Jin and Y. Tian, A novel electro chemiluminescence sensor based on nitrogen-doped graphene/CdTe quantum dots composite, Appl. Surf. Sci., 2014, 315, 22–27 CrossRef CAS
. - Z. H. Sheng, X. Q. Zheng, J. Y. Xu, W. J. Bao, F. B. Wang and X. H. Xia, Electrochemical sensor based on nitrogen doped graphene: simultaneous determination of ascorbic acid, dopamine, and uric acid, Biosens. Bioelectron., 2012, 34, 125–131 CrossRef CAS PubMed
. - X. Liu, W. Na, Q. Liu and X. Su, A novel label-free fluorescent sensor for highly sensitive detection of bleomycin based on nitrogen-doped graphene quantum dots, Anal. Chim. Acta, 2018, 1028, 45–49 CrossRef CAS PubMed
. - Z. Wan, M. Umer, M. Lobino, D. Thiel, N. T. Nguyen, A. Trinchi, M. J. A. Shiddiky, Y. Gao and Q. Li, Laser induced self-N-doped porous graphene as an electrochemical biosensor for femtomolar miRNA detection, Carbon, 2020, 163, 385–394 CrossRef CAS
. - A. Nag, A. Mitra and S. C. Mukhopadhyay, Graphene and its sensor-based applications: a review, Sens. Actuators, A, 2018, 270, 177–194 CrossRef CAS
. - C. K. Cheng, C. H. Lin, H. C. Wu, C. C. M. Ma, T. K. Yeh, H. Y. Chou, C. H. Tsai and C. K. Hsieh, The two-dimensional nanocomposite of molybdenum disulfide and nitrogen-doped graphene oxide for efficient counter electrode of dye-sensitized solar cells, Nanoscale Res. Lett., 2016, 11, 1–9 CrossRef CAS PubMed
. - P. Ranganathan, R. Sasikumar, S. M. Chen, S. P. Rwei and P. Sireesha, Enhanced photovoltaic performance of dye-sensitized solar cells based on nickel oxide supported on nitrogen-doped graphene nanocomposite as a photoanode, J. Colloid Interface Sci., 2014, 504, 570–578 CrossRef PubMed
. - J. Ou, C. Gong, M. Wang, J. Xiang and J. Liu, Highly efficient ZIF-8/graphene oxide derived N-doped carbon sheets as counter electrode for dye-sensitized solar cells, Electrochim. Acta, 2018, 286, 212–218 CrossRef CAS
. - Abid, P. Sehrawat, S. S. Islam, P. Mishra and S. Ahmad, Reduced graphene oxide (rGO) based wideband optical sensor and the role of temperature, defect States and quantum efficiency, Sci. Rep., 2018, 8, 1–13 CrossRef PubMed
. - M. Carbone, L. Gorton and R. Antiochia, An overview of the latest graphene-based sensors for glucose detection: the effects of graphene defects, Electroanalysis, 2015, 27, 16–31 CrossRef CAS
. - J. Zhu, H. Yin, J. Gong, M. S. H. Al-Furjan and Q. Nie, Easy one pot synthesis of NiO/nitrogen doped carbon spheres for highly sensitive enzyme free amperometric glucose sensors, Appl. Surf. Sci., 2018, 444, 56–64 CrossRef CAS
. - S. Yang, G. Li, D. Wang, Z. Qiao and L. Qu, Synthesis of nanoneedle-like copper oxide on N-doped reduced graphene oxide: a three-dimensional hybrid for nonenzymatic glucose sensors, Sens. Actuators, B, 2017, 238, 588–595 CrossRef CAS
. - K. Sivasankar, K. K. Rani, S. F. Wang, R. Devasenathipathy and C. H. Lin, Copper Nanoparticle and Nitrogen Doped Graphite Oxide Based Biosensor for the Sensitive Determination of Glucose, Nanomaterials, 2018, 8, 1–14 CrossRef PubMed
. - H. Jeong, D. M. Nguyen, M. S. Lee, H. G. Kim, S. C. Ko and L. K. Kwac, N-doped graphene–carbon nanotube hybrid networks attaching with gold nanoparticles for glucose non-enzymatic sensor, Mater. Sci. Eng. C, 2018, 90, 38–45 CrossRef CAS PubMed
. - Y. Liu, X. Dong and P. Chen, Biological and chemical sensors based on graphene materials, Chem. Soc. Rev., 2012, 41, 2283–2307 RSC
. - S. K. Krishnan, E. Singh, P. Singh, M. Meyyappan and H. S. Nalwa, A review on graphene-based nanocomposites for electrochemical and fluorescent biosensors, RSC Adv., 2019, 9, 8778–8881 RSC
. - Y. Lu, Y. Huang, M. Zhang and Y. Chen, Nitrogen-doped graphene material for supercapacitor applications, J. Nanosci. Nanotechnol., 2014, 14, 1134–1144 CrossRef CAS PubMed
. - Z. Yang, H. Nie, X. Chen, X. Chen and S. Huang, Recent progress in doped carbon nanomaterials as effective cathode catalysts for fuel cell oxygen reduction reaction, J. Power Sources, 2013, 236, 238–249 CrossRef CAS
. - C. H. Choi, M. W. Chung, H. C. Kwon, S. H. Park and S. I. Woo, B, N- and P, N-doped graphene as highly active catalyst for oxygen reduction reactions in acidic media, J. Mater. Chem. A, 2013, 1, 3694–3699 RSC
. - C. H. Choi, S. H. Park and S. I. Woo, Binary and ternary doping of nitrogen, boron, and phosphorus into carbon for enhancing electrochemical oxygen reduction activity, ACS Nano, 2012, 6, 7084–7091 CrossRef CAS PubMed
. - Y. Zhao, L. Yang, S. Chen, X. Wang, Y. Ma, Q. Wu, Y. Jiang, W. Qian and Z. Hu, Can boron and nitrogen co-doping improve oxygen reduction reaction activity of carbon nanotubes?, J. Am. Chem. Soc., 2013, 135, 1201–1204 CrossRef CAS PubMed
. - P. Lazar, R. Zboril, M. Pumera and M. Otyepka, Chemical nature of boron and nitrogen dopant atoms in graphene strongly influences its electronic properties, Phys. Chem. Chem. Phys., 2014, 16, 14231–14235 RSC
. - K. H. Hui, A. Ambrosi, Z. Sofer, M. Pumera and A. Bonanni, The dopant type and amount governs the electrochemical performance of graphene platforms for the antioxidant activity quantification, Nanoscale, 2015, 7, 9040–9045 RSC
. - D. Kplan, V. Swaminathan, G. Recine, R. Balu and S. Kama, Bandgap tuning of mono- and bilayer graphene doped with group IV elements, J. Appl. Phys., 2013, 113, 183701 CrossRef
. - S. K. Mudedla, K. Balamurugan and V. Subramanian, Computational study on the interaction of modified nucleobases with graphene and doped graphenes, J. Phys. Chem. C, 2014, 118, 16165–16174 CrossRef CAS
. - M. S. S. Azadeh, A. Kokabi, M. Hosseini and M. Fardmanesh, Tunable bandgap opening in the proposed structure of silicon-doped graphene, Micro Nano Lett., 2011, 6, 582–585 CrossRef CAS
. - F. Niu, J. M. Liu, L. M. Tao, W. Wang and W. G. Song, Nitrogen and silica co-doped graphene nanosheets for NO2 gas sensing, J. Mater. Chem. A, 2013, 1, 6130–6133 RSC
. - Y. Chen, Y. J. Liu, H. X. Wang, J. X. Zhao, Q. H. Cai, X. Z. Wang and Y. H. Ding, Silicon-doped graphene: an effective and metal-free catalyst for NO reduction to N2O?, ACS Appl. Mater. Interfaces, 2013, 5, 5994–6000 CrossRef CAS PubMed
. - Z. Wang, Y. Chen, P. Li, J. He, W. Zhang, Z. Guo, Y. Li and M. Dong, Synthesis of silicon-doped reduced graphene oxide and its applications in dye-sensitive solar cells and supercapacitors, RSC Adv., 2016, 6, 15080–15086 RSC
. - J. B. Maurya, Y. K. Prajapati, V. Singh, J. P. Saini and R. Tripathi, Improved performance of the surface plasmon resonance biosensor based on graphene or MoS2 using silicon, Opt. Commun., 2016, 359, 426–434 CrossRef CAS
. - R. Verma, B. D. Gupta and R. Jha, Sensitivity enhancement of a surface plasmon resonance-based biomolecules sensor using graphene and silicon layers, Sens. Actuators, B, 2011, 160, 623–631 CrossRef CAS
. - Z. Yunusa, M. N. Hamidon, A. Kaiser and Z. Awang, Gas Sensors: A Review, Sens. Transducers, 2014, 168, 61–75 Search PubMed
. - N. S. A. Eom, H. B. Cho, Y. Song, W. Lee, T. Sekino and Y. H. Cho, Room-Temperature H2 Gas Sensing Characterization of Graphene-Doped Porous Silicon via a Facile Solution Dropping Method, Sensors, 2017, 17, 2750–2757 CrossRef PubMed
. - H. G. Shiraz, Efficient room temperature hydrogen gas sensing based on graphene oxide and decorated porous silicon, Int. J. Hydrogen Energy, 2017, 42, 15966–15972 CrossRef CAS
. - S. Zhu, H. Sun, X. Liu, J. Zhuang and L. Zhao, Room-temperature NH3 sensing of graphene oxide film and its enhanced response on the laser textured silicon, Sci. Rep., 2017, 7, 14773–14781 CrossRef PubMed
. - Y. Yao, X. Chen, H. Guo, Z. Wu and X. Li, Humidity sensing behaviors of graphene oxide–silicon bi-layer flexible structure, Sens. Actuators, B, 2012, 161, 1053–1058 CrossRef CAS
. - H. M. Wang, H. X. Wang, Y. Chen, Y. J. Liu, J. X. Zhao, Q. H. Cai and X. Z. Wang, Phosphorus-doped graphene and (8,0) carbon nanotube: structural, electronic, magnetic properties, and chemical reactivity, Appl. Surf. Sci., 2013, 273, 302–309 CrossRef CAS
. - C. Zhang, N. Mahmood, H. Yin, F. Liu and Y. Hou, Synthesis of phosphorus-doped graphene and its multifunctional applications for oxygen reduction reaction and lithium ion batteries, Adv. Mater., 2013, 25, 4932–4937 CrossRef CAS PubMed
. - S. Some, J. Kim, K. Lee, A. Kulkarni, Y. Yoon, S. Lee, T. Kim and H. Lee, Highly air-stable phosphorus-doped n-type graphene field-effect transistors, Adv. Mater., 2012, 24, 5481–5486 CrossRef CAS PubMed
. - K. Chu, F. Wang, X. Wang, X. Zhao and Y. Tian, Electrochemical dopamine sensor based on P-doped graphene: highly active metal-free catalyst and metal catalyst support, Mater. Sci. Eng., C, 2017, 81, 452–458 CrossRef CAS PubMed
. - Y. Tian, Z. Wei, K. Zhang, S. Peng, X. Zhang, W. Liu and K. Chu, Three-dimensional phosphorus-doped graphene as an efficient metal-free electrocatalyst for electrochemical sensing, Sens. Actuators, B, 2017, 241, 584–591 CrossRef CAS
. - X. Zhang, K. P. Wang, L. N. Zhang, Y. C. Zhang and L. She, Phosphorus-doped graphene-based electrochemical sensor for sensitive detection of acetaminophen, Anal. Chim. Acta, 2018, 1036, 26–32 CrossRef CAS PubMed
. - R. Li, Z. Wei, X. Gou and W. Xu, Phosphorus-doped graphene nano sheets as efficient metal-free oxygen reduction electrocatalysts, RSC Adv., 2013, 3, 9978–9984 RSC
. - M. L. Sanchez, A. Primo and H. Garcia, P-doped graphene obtained by pyrolysis of modified alginate as a photocatalyst for hydrogen generation from water–methanol mixtures, Angew. Chem., Int. Ed., 2013, 52, 11813–11816 CrossRef PubMed
. - F. Niu, L. M. Tao, Y. C. Deng, Q. H. Wang and W. G. Song, Phosphorus doped graphene nanosheets for room temperature NH3 sensing, New J. Chem., 2014, 38, 2269–2272 RSC
. - Z. Yang, Z. Yao, G. Li, G. Fang, H. Nie, Z. Liu, X. Zhou, X. Chen and S. Huang, Sulfur-doped graphene as an efficient metal-free cathode catalyst for oxygen reduction, ACS Nano, 2012, 6, 205–211 CrossRef CAS PubMed
. - D. R. Lide, CRC Handbook of Chemistry and Physics, CRC Press, Boca Raton, FL, 84th edn, 2003 Search PubMed
. - J. P. Paraknowitsch, A. Thomas and J. Schmidt, Microporous sulfur-doped from thienyl-based polymer network precursors, Chem. Commun., 2011, 47, 8283–8285 RSC
. - P. A. Denis, R. Faccio and A. W. Mombru, Is It Possible to Dope Single-Walled Carbon Nanotubes and Graphene with Sulfur?, ChemPhysChem, 2009, 10, 715–722 CrossRef CAS PubMed
. - F. Shahzad, S. A. Zaidi and C. M. Koo, Highly sensitive electrochemical sensor based on environmentally friendly biomass-derived sulphur-doped graphene for cancer biomarker detection, Sens. Actuators, B, 2017, 241, 716–724 CrossRef CAS
. - Y. Tian, Y. Liu, W. Wang, X. Zhang and W. Peng, Sulfur-doped graphene-supported Ag nanoparticles for nonenzymatic hydrogen peroxide detection, J. Nanopart. Res., 2015, 17, 1–9 CrossRef CAS
. - Y. Fu, Y. Yang, T. Tuersun, Y. Yu and J. Zhi, Simple preparation and highly selective detection of silver ions using an electrochemical sensor based on sulfur-doped graphene and a 3,3′,5,5′-tetramethylbenzidine composite modified electrode, Analyst, 2018, 143, 2076–2082 RSC
. - B. Manna and C. R. Raj, Nanostructured sulfur-doped porous reduced graphene oxide for the ultrasensitive electrochemical detection and efficient removal of Hg(II), ACS Sustainable Chem. Eng., 2018, 6, 6175–6182 CrossRef CAS
. - C. Liang, Y. L. Wang and T. Li, Sulfur-doping in graphene and its high selectivity gas sensing in NO2, 2015 Transducers – 2015 18th International Conference on Solid-State Sensors, Actuators and Microsystems (TRANSDUCERS), 2015, pp. 1420–1423 Search PubMed
. - L. Guo, Y. Wang and T. Li, High performance nitrogen dioxide sensors with sulfur doped graphene and micro-hot platform, IEEE Sens. J., 2017, 1–3 Search PubMed
. - X. Xu, S. Jiang, Z. Hu and S. Liu, Nitrogen-doped carbon nanotubes: high electrocatalytic activity toward the oxidation of hydrogen peroxide and its application for biosensing, ACS Nano, 2010, 4, 4292–4298 CrossRef CAS PubMed
. - C. C. Hwang, G. Ruan, L. Wang, H. Zheng, E. L. G. Samuel, C. Xiang, W. Lu, W. Kasper, K. Huang, Z. Peng, Z. Schaefer, A. T. Kan, A. A. Marti, M. S. Wong, M. B. Tomson and J. M. Tour, Carbon-based nanoreporters designed for subsurface hydrogen sulphide detection, ACS Appl. Mater. Interfaces, 2014, 6, 7652–7658 CrossRef CAS PubMed
. - X. Ren, H. Ma, T. Zhang, Y. Zhang, T. Yan, B. Du and Q. Wei, A Sulfur-Doped Graphene-Based Immunological Biosensing Platform for Multianalysis of Cancer, ACS Appl. Mater. Interfaces, 2017, 9, 37637–37644 CrossRef CAS PubMed
. - J. Liang, Y. Jiao, M. Jaroniec and S. Z. Qiao, Sulfur and nitrogen dual-doped mesoporous graphene electrocatalyst for oxygen reduction with synergistically enhanced performance, Angew. Chem., Int. Ed., 2012, 51, 11496–11500 CrossRef CAS PubMed
. - S. Kumar, S. Patra, R. Madhuri and P. K. Sharma, Dual doped graphene oxide for electrochemical sensing of europium ion, AIP Conf. Proc., 2017, 1832, 050068 CrossRef
. - Y. Tian, Y. Ma, H. Liu, X. Zhang and W. Peng, One-step and rapid synthesis of nitrogen and sulfur co-doped graphene for hydrogen peroxide and glucose sensing, J. Electroanal. Chem., 2015, 742, 8–14 CrossRef CAS
. - N. Karikalan, R. Karthik, S. M. Chen, C. Karuppiah and A. Elangovan, Sonochemical Synthesis of Sulfur Doped Reduced Graphene Oxide Supported CuS Nanoparticles for the Non-Enzymatic Glucose Sensor Applications, Sci. Rep., 2017, 7, 1–10 CrossRef CAS PubMed
. - B. Y. Fang, C. Li, Y. Y. Song, F. Tan, Y. C. Cao and Y. D. Zhao, Nitrogen-Doped Graphene Quantum Dot for Direct Fluorescence Detection of Al3+ in Aqueous Media and Living Cells, Biosens. Bioelectron., 2018, 100, 41–48 CrossRef CAS PubMed
. - S. Bian, C. Shen, Y. Qian, J. Liu, F. Xi and X. Dong, Facile Synthesis of Sulfur-Doped Graphene Quantum Dots as Fluorescent Sensing Probes for Ag+ Ions Detection, Sens. Actuators, B, 2017, 242, 231–237 CrossRef CAS
. - D. Peng, L. Zhang, R.-P. Liang and J.-D. Qiu, Rapid Detection of Mercury Ions Based on Nitrogen-Doped Graphene Quantum Dots Accelerating Formation of Manganese Porphyrin, ACS Sens., 2018, 3, 1040–1047 CrossRef CAS PubMed
. - C. Zhang, B. Lin, Y. Cao, M. Guo and Y. Yu, Fluorescence Determination of Omethoate Based on a Dual Strategy for Improving Sensitivity, J. Agric. Food Chem., 2017, 65, 3065–3073 CrossRef CAS PubMed
. - Y. Liu, N. Cao, W. Gui and Q. Ma, Nitrogen-Doped Graphene Quantum Dots-Based Fluorescence Molecularly Imprinted Sensor for Thiacloprid Detection, Talanta, 2018, 183, 339–344 CrossRef CAS PubMed
. - L. Chen, L. Song, Y. Zhang, P. Wang, Z. Xiao, Y. Guo and F. Cao, Nitrogen and Sulfur Codoped Reduced Graphene Oxide as a General Platform for Rapid and Sensitive Fluorescent Detection of Biological Species, ACS Appl. Mater. Interfaces, 2016, 8, 11255–11261 CrossRef CAS PubMed
. - S. Li, Y. Li, J. Cao, J. Zhu, L. Fan and X. Li, Sulfur-Doped Graphene Quantum Dots as a Novel Fluorescent Probe for Highly Selective and Sensitive Detection of Fe3+, Anal. Chem., 2014, 86, 10201–10207 CrossRef CAS PubMed
. - L. Gao, L. Ju and H. Cui, Chemiluminescent and Fluorescent Dual-Signal Graphene Quantum Dots and Their Application in Pesticide Sensing Arrays, J. Mater. Chem. C, 2017, 5, 7753–7758 RSC
. - F. Nemati, M. Hosseini, R. Zare-Dorabei and M. R. Ganjali, Sensitive Recognition of Ethion in Food Samples Using Turn-on Fluorescence N and S Co-Doped Graphene Quantum Dots, Anal. Methods, 2018, 10, 1760–1766 RSC
. - A. B. Ganganboina, A. D. Chowdhury and R.-A. Doong, N Doped Graphene Quantum Dots-Decorated V2O5 Nanosheet for Fluorescence Turn Off–On Detection of Cysteine, ACS Appl. Mater. Interfaces, 2017, 10, 614–624 CrossRef PubMed
. - N. T. N. Anh and R.-a. Doong, One-Step Synthesis of Size-Tunable Gold@Sulfur-Doped Graphene Quantum Dot Nanocomposites for Highly Selective and Sensitive Detection of Nanomolar 4-Nitrophenol in Aqueous Solutions with Complex Matrix, ACS Appl. Nano Mater., 2018, 1, 2153–2163 CrossRef
. - R. Zbořil, F. Karlický, A. B. Bourlinos, T. A. Steriotis, A. K. Stubos, V. Georgakilas, K. Šafářová, D. Jančík, C. Trapalis and M. Otyepka, Graphene Fluoride: A Stable Stoichiometric Graphene Derivative and its Chemical Conversion to Graphene, Small, 2010, 6, 2885–2891 CrossRef PubMed
. - H. L. Poh, P. Šimek, Z. Sofer and M. Pumera, Halogenation of Graphene with Chlorine, Bromine, or Iodine by Exfoliation in a Halogen Atmosphere, Chem.–Eur. J., 2013, 19, 2655–2662 CrossRef CAS PubMed
. - R. B. Garrido, H. G. Baldovi, M. L. Sachez, M. Alvaro and H. Garcia, Photocatalytic hydrogen generation from water–methanol mixtures using halogenated reconstituted graphenes, J. Mater. Chem. A, 2013, 1, 11728–11734 RSC
. - X. B. Zhang, L. Zhang, J. S. Hu and X. H. Huang, Facile hydro thermal synthesis and improved photocatalytic activities of Zn2+ doped Bi2MoO6 nanosheets, RSC Adv., 2016, 6, 32349–32357 RSC
. - O. Jankovsky, P. Simek, K. Klimova, D. Sedmidubsky, S. Matejkova, M. Pumera and Z. Sofer, Towards graphene bromide: bromination of graphite oxide, Nanoscale, 2014, 6, 6065–6074 RSC
. - X. Sun, Y. Zhang, P. Song, J. Pan, L. Zhuang, W. Xu and W. Xing, Fluorine-doped carbon blacks: highly efficient metal-free electrocatalysts for oxygen reduction reaction, ACS Catal., 2013, 3, 1726–1729 CrossRef CAS
. - Z. Yao, H. Nie, Z. Yang, X. Zhou, Z. Li and S. Huang, Catalyst-free synthesis of iodine-doped graphene via a facile thermal annealing process and its use for electrocatalytic oxygen reduction in an alkaline medium, Chem. Commun., 2012, 48, 1027–1029 RSC
. - I. Y. Jeon, H. J. Choi, M. Choi, J. M. Seo, S. M. Jung, M. J. Kim, S. Zhang, L. Zhang, Z. Xia, L. Dai, N. Park and J. B. Baek, Facile, scalable synthesis of edge halogenated graphene nanoplatelets as efficient metal-free electrocatalysts for oxygen reduction reaction, Sci. Rep., 2013, 3, 1–7 Search PubMed
. - Y. Zhan, B. Zhang, L. Cao, X. Wu, Z. Lin, X. Yu, X. Zhang, D. Zeng, F. Xie, W. Zhang, J. Chen and H. Meng, Iodine doped graphene as anode material for lithium ion battery, Carbon, 2015, 94, 1–8 CrossRef CAS
. - K. Chu, F. Wang, X. L. Zhao, X. Wei, X. W. Wang and Y. Tian, One-step and low-temperature synthesis of iodine-doped graphene and its multifunctional applications for hydrogen evolution reaction and electrochemical sensing, Electrochim. Acta, 2017, 246, 1155–1162 CrossRef CAS
. - K. P. Wang, Y. C. Zhang, X. Zhang and L. Shen, Green preparation of chlorine-doped graphene and its application in electrochemical sensor for chloramphenicol detection, SN Appl. Sci., 2019, 157 CrossRef
. - S. Singh, M. Singh, K. Mitra, R. Singh, S. K. S. Gupta, I. Tiwari and B. Ray, Electrochemical sensing of hydrogen peroxide using brominated graphene as mimetic catalase, Electrochim. Acta, 2017, 258, 1435–1444 CrossRef CAS
. - K. J. Jeon, Z. Lee, E. Pollak, L. Moreschin, A. Bostwick, C. M. Park, R. Mendelsberg, V. Radmilovic, R. Kostecki, T. J. Richardson and E. Rotenberg, Fluorographene: a wide bandgap semiconductor with ultraviolet luminescence, ACS Nano, 2011, 5, 1042–1046 CrossRef CAS PubMed
. - W. Feng, P. Long, Y. Feng and Y. Li, Two-dimensional fluorinated graphene: synthesis, Structures, Properties and applications, Adv. Sci., 2016, 3, 1–22 Search PubMed
. - L. Cheng, S. Jandhyala, G. Mordi, A. T. Lucero, J. Huang, A. Azcati, R. Addou, R. M. Wallace, L. Colombo and J. Kim, Partially fluorinated graphene: structural and electrical characterization, ACS Appl. Mater. Interfaces, 2016, 8, 5002–5008 CrossRef CAS PubMed
. - D. Damien, P. M. Sudeep, T. N. Narayanan, M. R. Anantharaman, P. M. Ajayan and M. M. Shaijumon, Fluorinated graphene-based electrodes for high performance primary lithium batteries, RSC Adv., 2013, 3, 25702–25706 RSC
. - R. R. Aburto, T. N. Narayanan, Y. Nagaoka, T. Hasumura, T. M. Mitcham, T. Fukuda, P. J. Cox, R. R. Bouchard, T. Maekawa, D. S. Kumar, S. V. Torti, S. A. Mani and P. M. Ajayan, Fluorinated graphene oxide; a new multimodal material for biological applications, Adv. Mater., 2013, 25, 5632–5637 CrossRef PubMed
. - F. G. Zhao, G. Zhao, X. H. Liu, C. W. Ge, J. T. Wang, B. L. Li, Q. G. Wang, W. S. Li and Q. Y. Chen, Fluorinated graphene: facile solution preparation and tailorable properties by fluorine-content tuning, J. Mater. Chem. A, 2014, 2, 8782–8789 RSC
. - S. Boopathi, T. N. Narayanan and S. S. Kumar, Improved heterogeneous electron transfer kinetics of fluorinated graphene derivatives, Nanoscale, 2014, 6, 10140–10146 RSC
. - X. Wang, Y. Dai, J. Gao, J. Huang, B. Li, C. Fan, J. Yang and X. Liu, High yield production of highly fluorinated graphene by direct heating fluorination of graphene-oxide, ACS Appl. Mater. Interfaces, 2013, 5, 8294–8299 CrossRef CAS PubMed
. - M. S. Park, K. H. Kim, M. J. Kim and Y. S. Lee, NH3 gas sensing properties of a gas sensor based on fluorinated graphene oxide, Colloids Surf., A, 2016, 490, 104–109 CrossRef CAS
. - A. R. Thiruppathi, B. Sidhureddy, W. Keeler and A. Chen, Facile one-pot synthesis of fluorinated graphene oxide for electrochemical sensing of heavy metal ions, Electrochem. Commun., 2017, 76, 42–46 CrossRef CAS
. - V. S. Manikandan, B. Sidhureddy, A. R. Thiruppathi and A. Chen, Sensitive electrochemical detection of caffeic acid in wine based on fluorine-doped graphene oxide, Sensors, 2019, 19, 1604 CrossRef CAS PubMed
.
|
This journal is © The Royal Society of Chemistry 2020 |
Click here to see how this site uses Cookies. View our privacy policy here.