DOI:
10.1039/D0RA04377J
(Paper)
RSC Adv., 2020,
10, 24697-24704
Large interlayer spacing Nb4C3Tx (MXene) promotes the ultrasensitive electrochemical detection of Pb2+ on glassy carbon electrodes†
Received
16th May 2020
, Accepted 18th June 2020
First published on 29th June 2020
Abstract
A Nb4C3Tx (MXene)-modified glassy carbon electrode was used for the electrochemical detection of Pb2+ ions in aqueous media. The sensing platform was evaluated by anodic stripping analysis after optimizing the influencing factors such as pH, deposition potential, and time. The large interlayer spacing, high c lattice parameter and higher conductivity of Nb4C3Tx compared to other MXenes enhance the electrochemical detection of Pb2+. The developed sensor can reach a detection limit of 12 nM at a potential ∼−0.6 V. Additionally, the developed sensor showed promising selectivity in the presence of Cu2+ and Cd2+, and stability for at least 5 cycles of continuous measurements with good repeatability. This work demonstrates the potential applications of Nb4C3Tx towards the development of effective electrochemical sensors.
Introduction
Lead (Pb) is a common heavy metal, used in a variety of industrial processes and anthropogenic activities.1 Pb2+ ions are known for their extremely harmful biological toxicity through enzyme inhibition and induction of oxidative stress and can cause chronic damage to several human body systems, including kidneys, gastrointestinal system, nervous system, and reproductive system.2–4 Moreover, Pb contamination poses a serious health and environmental hazard due to its high accumulation and low clearance rate at contaminated sites.5,6 The maximum level of Pb2+ in drinking water set by the United States Environmental Protection Agency (EPA) is 15 μg L−1 (72 nM),7 while the World Health Organization (WHO) limit for the blood Pb2+ level is 100 μg L−1 (483 nM).5,8
Various techniques are used for the detection of Pb2+ in water including atomic absorption/fluorescence spectrometry, optical emission spectrometry, inductively coupled plasma mass spectrometry, and chemical or optical sensors.9–11 Despite their reliability, the operations and maintenance associated with these methods are tedious, costly, and not suitable for on-site monitoring. Electrochemical techniques are inexpensive, selective, highly sensitive and effective alternative for the detection of various toxic substances and heavy metals.12–14 Moreover, electrochemical methods are characterized by their portability, easy operation, quick analysis time, and low maintenance and instrumentation costs.8,15 Stripping voltammetry (especially anodic stripping) have been used as the sensitive and powerful electrochemical technique for the detection of heavy metal ions.1,9 Different carbon nanomaterials such as carbon nanotubes, graphene, and its composite materials have been used as the sensing platform for sensitive Pb2+ detection.16–20
The 2D transition metal carbides and carbonitrides (MXenes) have attracted broad attention with unique physicochemical properties.21–24 The large lateral size with few nanometer thickness, embedded with good hydrophilicity, and activated metallic hydroxide sites render MXenes as promising materials for environmental remediation applications.25–28 MXene surfaces is negatively charged due to its surface functional groups, which facilitate the adsorption of several toxic heavy metals and emerging contaminants.27,29 In addition, MXenes nanosheets having strong trapping power to small cations, due to less inter planner distance (<2 Å).30 It was reported that Ti3C2Tx which is the most studied MXene, is an efficient adsorbent for the gold, lead and chromium cations.30 In addition, the intercalation of different cations with various sizes and charges are possible between Ti3C2Tx layers.30 After alkalization intercalation of Ti3C2Tx, alk-MXene (Ti3C2(OH/ONa)xF2−x) exhibits superior sorption behavior for Pb2+ in presence of high levels of interfering cations such as Ca2+ and Mg2+.31 Recently, alkalization-intercalated Ti3C2Tx modified electrode displayed enhanced electrochemical response towards the detection of Cd2+, Pb2+, Cu2+ and Hg2+.32 The alkalization process increases the c lattice parameter of Ti3C2Tx from 19.741 Å to 26.187 Å and the alkalization process results in unique morphology and alteration in surface chemistry. This leads to enhanced electrochemical responses for alkalization-intercalated Ti3C2Tx towards the heavy metal detection in comparison with the Ti3C2Tx.
Nb4C3Tx is another member of the MXenes family, prepared by etching of Al from the Nb4AlC3 MAX phase.33,34 Recently, Nb4C3Tx have been explored in a number of applications, including dye adsorption,35 energy storage devices,23,36–38 hematopoietic recovery,33 photothermal tumor eradication,22 supercapacitors,39 and photocatalytic hydrogen production.40 Even though, the electrochemical performance of Nb4C3Tx has not been widely explored towards sensing applications.
In this paper, we evaluate the electrochemical performance of Nb2CTx and Nb4C3Tx on the glassy carbon electrode (GCE) and their application as sensing platform for the detection of Pb2+ in the aqueous media. To the best of our knowledge, this is the first report discusses the application of Nb4C3Tx as electrochemical sensor for heavy metals.
Experimental
Materials
Phosphate buffer (PB) solution, NaOH, H2SO4, K3[Fe(CN)6], K4[Fe(CN)6]·3H2O, sodium acetate, acetic acid, lead nitrate, copper sulfate and cadmium acetate were purchased from Sigma Aldrich. The MAX phases of Nb2CTx and Nb4C3Tx (Nb2AlC and Nb4AlC3 respectively) were prepared by mixing powders of niobium (Alfa Aesar, 99.98%, −325 mesh), aluminum (Alfa Aesar, 99.9%, −325 mesh), and carbon (Alfa Aesar, 99%, 7–11 micron) at different ratios, followed by heating under an argon (Ar) flow. The detailed procedure is given in the ESI† and in the ref. 34 and 37.
Synthesis of Nb2CTx and Nb4C3Tx
The synthesis of multilayered Nb2CTx (ML-Nb2CTx) and Nb4C3Tx (ML-Nb4C3Tx) MXenes were done by hydrofluoric acid (HF) etching of Al layers from MAX phases Nb2AlC and Nb4AlC3 respectively. The Nb2AlC or Nb4AlC3 powders were stirred for 96 h at 40 °C after immersing in 50% HF aqueous solution. The resulting reaction mixture were washed 5 to 6 times using DI water and centrifuged at 3500 rpm to separate the ML-MXenes as settled powders from the supernatants. The resulting ML-MXenes were washed using ethanol, and dried at 30 °C under flow of argon. The delaminated Nb2CTx (DL-Nb2CTx) and Nb4C3Tx MXenes (DL-Nb4C3Tx) flakes were prepared by probe sonication (Cole Parmer, Ultrasonic Processor, 60% amplitude, 750 watt) of ML-Nb2CTx and ML-Nb4C3Tx MXenes (100 mg) in 5 mL of degassed DI water at 20 °C, under a flow of Ar gas for 1 h, followed by freeze-drying.
Characterization
The morphology of prepared DL-Nb2CTx and DL-Nb4C3Tx MXenes were characterized by scanning electron microscopy (SEM), using a FEI Quanta 650 FEG. The transmission electronic microscopy (TEM) was performed by using FEI Talos F200×. The ethanol dispersions of DL-Nb2CTx and DL-Nb4C3Tx were mounted on a lacey Formvar carbon-coated Cu grid for TEM analysis. Bruker D8 Advance X-ray diffractometer with Cu-Kα radiation (λ = 1.54056 Å) was used to record X-ray diffractograms.
Fabrication of DL-Nb2CTx and DL-Nb4C3Tx modified electrodes and electrochemical analysis
Prior to experiments, GCE was polished with alumina powder followed by sonication in a copious amount of ethanol and distilled water. 0.2 mg of Nb2CTx/Nb4C3Tx was dissolved in 1 mL of distilled water and homogeneous suspension was made by sonication for 1 min. Then, 6 μL of this suspension was deposited onto GCE and dried at room temperature for overnight under inert atmosphere. CHI760E electrochemical work station (CHI, Texas, USA) was used to conduct all electrochemical measurements with a three electrode system. The three electrode system consist of a modified GCE as the working electrode, Pt wire as the counter electrode, and Ag/AgCl in saturated KCl as the reference electrode. Cyclic voltammetry (CV) were performed at a scan rate of 100 mV s−1 in the 0.1 M PB solution (pH 7) and in the solution of 0.1 M KCl with 10 mM [Fe(CN)6]3−/4−. Electrochemical impedance spectroscopy (EIS) measurements were performed at a potential of 10 mV in the 100 kHz to 0.1 Hz frequency range.
Stripping voltammetry analysis
Square wave anodic stripping voltammetry (SWASV) measurements were used to detect Pb2+ in acetate buffer solution (0.1 M, pH 5.0) containing different concentration Pb2+. The pre concentration step was performed at −1.2 V for 150 s while stirring the electrolyte solution. SWASV voltammograms were recorded after an equilibration period of 15 s, in the potential range from −0.8 V to 0 V with square wave potential scan having 4 mV increment potential, 25 mV amplitude and 50 Hz frequency. After each anodic stripping measurement, a desorption step was performed at a potential of 0.8 V for 100 s under stirring to remove the residual heavy metal ions on the electrode surface. For interference measurements, the pre concentration step was carried out at the potential of −1.2 V for 150 s while string the electrolyte solution containing Cd2+ and Cu2+ (5 times concentration than Pb2+) followed by recording SWASV voltammograms in the potential range from −1.2 V to 0 V. Error bars shows the standard deviation for three repetitive measurements in each experiment.
Results and discussion
Material characterization
DL-Nb2CTx and DL-Nb4C3Tx MXenes nanosheets were prepared by acid etching of Al layer using HF aqueous solution from their corresponding MAX phases as described in the Experimental section, followed by sonication and freeze drying. SEM images in (Fig. 1(a and c)) describe the typical accordion-like structure in both multi-layered (ML)-Nb2CTx and ML-Nb4C3Tx (Fig. 1(a and c)). After probe sonication, DL-Nb2CTx and DL-Nb4C3Tx showed similar wrinkled sheet-like structure (Fig. 1(b and d)). Energy-dispersive spectroscopy (EDS) confirmed the presence of fluorine, oxygen, carbon and niobium elements in both MXenes (Fig. S1†). The TEM images revealed electron transparent single or few sheets with an average of 200–400 nm sheet size (Fig. 1(c and f)). In addition, the high resolution TEM (HR-TEM) images of DL-Nb2CTx and DL-Nb4C3Tx shown in the inset shows the d-spacing ∼11.5 Å and ∼15 Å, respectively.36 The XRD patterns of ML-Nb2CTx, DL-Nb2CTx, ML-Nb4C3Tx and DL-Nb4C3Tx are given in Fig. S2.† After delamination the intensity of (002) peaks were increased while intensity of other peaks decreased. The strong characteristic peak (002) in both DL-Nb2CTx (at 2theta of 7.87°) and DL-Nb4C3Tx (at 2theta of 5.94°) confirmed the successful delamination and preparation of DL-MXenes.23,35 DL-Nb2CTx has a smaller c lattice parameter (c-LP) of 22.44 Å as compared to 29.70 Å for DL-Nb4C3Tx, as calculated from (002) peak position. The corresponding interlayer distance for DL-Nb2CTx was 11.22 Å and 14.85 Å for DL-Nb4C3Tx, which is in a good agreement with the TEM results. The interlayer spacing in DL-Nb4C3Tx were higher than that of DL-Nb2CTx, which could explain the interplanar distance increases with carbide blocks (n) in each MXene layer of Mn+1XnTx. A 14.85 Å spacing of DL-Nb4C3Tx is larger than most studied MXenes.41 As far as the electrochemical performance is concerned, larger interlayer space allows faster adsorption and intercalation of ions, and it enhances ion diffusion and charge transport of the electrolyte.42
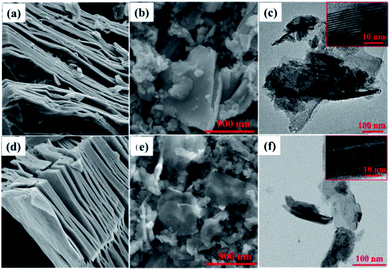 |
| Fig. 1 SEM image of (a) ML-Nb2CTx (b) DL-Nb2CTx (d) ML-Nb4C3Tx and (e) DL-Nb4C3Tx. TEM image of (c) DL-Nb2CTx and (f) DL-Nb4C3Tx. HR-TEM image of DL-Nb2CTx and DL-Nb4C3Tx are shown in the inset of (c) and (f) respectively. | |
Electrochemical characterization of Nb2CTx and Nb4C3Tx
The fabrication of Nb4C3Tx modified GCE and the development of Nb4C3Tx modified sensor for Pb2+ detection is given in Scheme 1. CV and EIS analysis were used to investigate the electrochemical behaviour of Nb2CTx and Nb4C3Tx modified electrodes in an aqueous solution containing ferrocyanide/ferricyanide redox couple solution. As observed in Fig. 2(a), well-defined redox peaks were observed for all the electrodes and these peaks can be attributed to the reversible redox behaviour of [Fe(CN)6]3−/4−. The ΔEp values for Nb2CTx/GCE and Nb4C3Tx/GCE were 197 mV and 141 mV, respectively and the lowest ΔEp value of Nb4C3Tx/GCE indicating highest electron transfer kinetics than Nb2CTx/GCE. In addition, the ΔIp values for Nb2CTx/GCE and Nb4C3Tx/GCE were 114.51 mV and 110.10 mV respectively. The higher value of ΔIp again confirmed the highest electron transfer kinetics of Nb4C3Tx/GCE than Nb2CTx/GCE. The electrochemical active surface area was calculated by using the Randles–Sevcik equation and it has a value of 0.574 × 10−3 cm2 and 0.621 × 10−3 cm2 for Nb2CTx and Nb4C3Tx respectively (see ESI†).43 The Nyquist plot for Nb2CTx/GCE and Nb4C3Tx/GCE is given in Fig. 2(b). The charge transfer resistance (Rct) parameter was obtained after fitting the Nyquist plot and was used to evaluate the electron-transfer kinetics of the redox couple at the electrode interface.44 The Rct values obtained for Nb2CTx/GCE and Nb4C3Tx/GCE were (2142 ± 24) Ω and (1732 ± 19) Ω respectively by fitting with R(Q[RW]) Randles equivalent circuit. The resistivity is lowest for Nb4C3Tx/GCE and hence Nb4C3Tx is having the highest conductivity than Nb2CTx which can be justified by the higher ‘n’ value of Nb4C3Tx (n = 3) than Nb2CTx (n = 1).45 From the CV and EIS analysis, it was found that Nb4C3Tx having highest electrochemical activity.
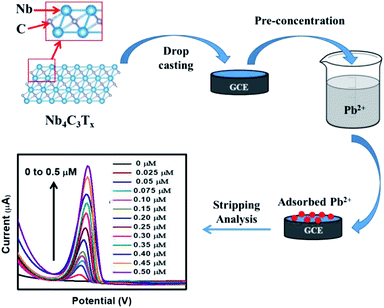 |
| Scheme 1 The electrode fabrication and development of Nb4C3Tx modified sensor for Pb2+ detection. | |
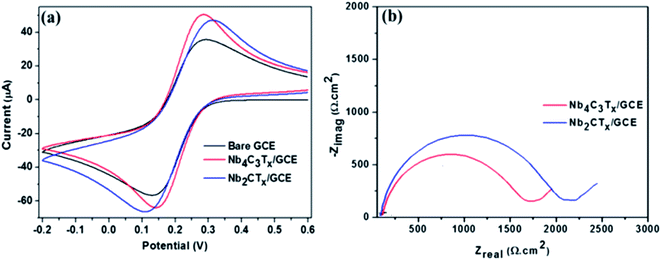 |
| Fig. 2 (a) CVs of bare GCE, Nb2CTx/GCE and Nb4C3Tx/GCE. (b) Nyquist plots of Nb2CTx/GCE and Nb4C3Tx/GCE. Frequency range: 0.1 Hz to 10 kHz. The experiments were performed in the solution of 0.1 M KCl with 10 mM [Fe(CN)6]3−/4−. | |
Stripping behaviour of Pb2+ and optimization of experimental parameters
The SWASV response of the bare GCE, Nb2CTx/GCE and Nb4C3Tx/GCE were analyzed for the detection of Pb2+ ions using acetate buffer solution containing 0.5 μM Pb2+. Compared with the bare GCE, well defined stripping peaks at around −0.58 V were observed for the Nb2CTx/GCE and Nb4C3Tx/GCE and the peak current is highest for Nb4C3Tx/GCE than Nb2CTx/GCE (Fig. 3(a)). The highest response for Nb4C3Tx can be attributed to large interlayer spacing, high c lattice parameter value than Nb2CTx which is evident from TEM and XRD measurements. In addition, it was established that the resistivity of Nb4C3Tx is lower than Nb2CTx,45 which corresponds to the higher conductivity of Nb4C3Tx as evident from electrochemical analysis (Fig. 2). Hence, Nb4C3Tx has been selected as the sensing platform for the sensitive detection of Pb2+.
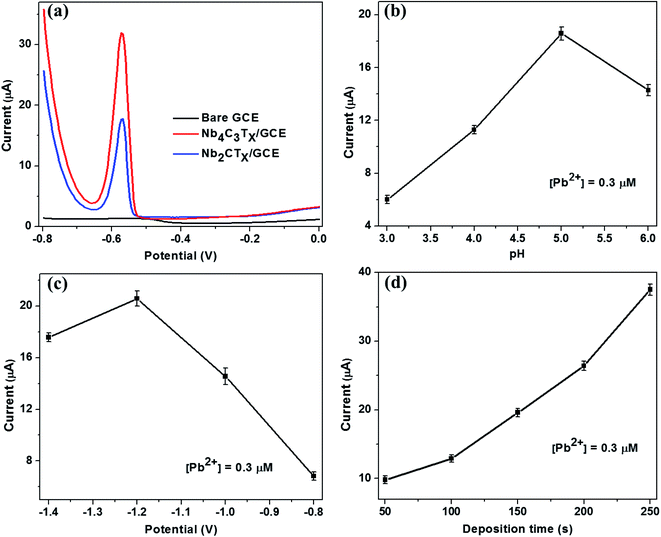 |
| Fig. 3 (a) SWASV responses for bare GCE, Nb2CTx/GCE and Nb4C3Tx/GCE in presence of 0.5 μM of Pb2+ in 0.5 M acetate buffer at pH 5, deposition potential of −1.2 V and deposition time of 150 s. Optimization of experimental parameters. (b) pH, (c) deposition potential, and (d) deposition time towards the stripping current of Nb4C3Tx/GCE. | |
The optimum conditions for highly sensitive Pb2+ detection were evaluated by changing the critical parameters such as pH, deposition time and potential. The impact of pH on the stripping current was studied from 3.0 to 6.0 (Fig. 3(b)). The peak current of Pb2+ was increased with increasing the pH from 3.0 to 5.0 and then decreased at pH = 6. The presence of [Nb–O]–H+ groups favours the ion exchange behaviour of Nb4C3Tx and this behaviour increases with the pH which results in the strengthening of stripping current.32 The decrease in peak currents at pH 6 could be attributed to the hydrolysis of cations results in the formation of more Pb(OH)2, which inhibits the further accumulation of Pb2+. Considering the maximum observed stripping peak current, pH 5 was selected as optimal for subsequent experiments.
Deposition potential and time are also critical factors for stripping analysis to detect heavy metal ions. The deposition potential was varied from −1.4 to −0.8 V and the resulting stripping currents increases with negative potential until −1.2 V (Fig. 3(c)). A reduction in the current response was observed at deposition potential lower than −1.2 V. This might be due to occurrence of more hydrogen evolution in the acetate buffer. Hence, the deposition potential of −1.2 V was selected as optimal for further experiments. The deposition time was varied from 50 to 250 s and the stripping peak currents response was evaluated (Fig. 3(d)). The stripping peak current have increased linearly with the deposition time increase. A deposition time of 150 s was selected for subsequent experiments considering the concession between short measurement time, high sensitivity and good reproducibility favoured for practical applications.
Quantitative detection of Pb2+
Under the optimal conditions, the quantitative detection of Pb2+ was performed by SWASV on Nb4C3Tx/GCE. Fig. 4(a) shows the SWASV responses at different concentrations from 0 to 0.5 μM of Pb2+. The stripping peak currents increases with increasing the Pb2+ concentration and a good linear relationship was observed in the concentration range from 0.025 μM to 0.5 μM. There was no response for the developed sensor when the Pb2+ concentration was less than 0.025 μM and this concentration can be regarded as limit of quantification of the sensor. The corresponding calibration plot is given in Fig. 4(b), by plotting the peak current vs. Pb2+ concentration. The calibration plot equation was represented as i (μA) = 58.49[Pb2+] + 1.13, with 0.99688 as correlation coefficient (R2). The limit of detection (LOD) was calculated as 12 nM (S/N = 3), which is much lower (or comparable) than the similar kind of sensors for Pb2+ detection (refer Table 1). In a similar work with alkaline intercalated Ti3C2Tx MXene as platform for electrochemical detection of heavy metals, the stripping analysis showed a detection limit of 32 nM with linear range of 0.10–0.55 μM and this response is significantly higher than bare Ti3C2Tx. The analytical performance of Nb4C3Tx modified GCE is higher compared to bare Ti3C2Tx as well as alkaline intercalated Ti3C2Tx. The analytical performance of Nb4C3Tx modified GCE was compared with other similar reported electrodes (summarized in Table 1) and it showed that the Nb4C3Tx modified GCE exhibited a wider range, promising detection limit and enhanced sensitivity. The large interlayer spacing and high c lattice parameter of Nb4C3Tx (in comparison with Nb2CTx) have allowed for the adsorption of larger amount of Pb2+ between the sheets as well as the higher conductivity of Nb4C3Tx have improved the electrochemical response on the electrode surface. This strategy can be easily implemented into screen printed electrodes for practical and portable applications considering the versatile sensor fabrication by drop-casting Nb4C3Tx on the electrode followed by drying.46
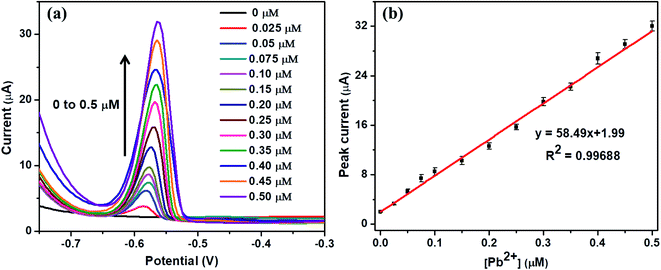 |
| Fig. 4 (a) SWASV response of the Nb4C3Tx/GCE for in presence of Pb2+ from 0 to 0.5 μM. (b) The calibration plot of peak current vs. Pb2+ concentrations. | |
Table 1 The analytical performance of different 2D materials used for Pb2+ detection
Electrode |
Detection technique |
Detection limit |
Linear range |
Ref. |
L-Cys/AuNPs/NG/GCE |
SWV |
56 nM |
1–80 μM |
1 |
Bismuth modified exfoliated graphite |
SWASV |
53 nM |
1.0–250 μM |
9 |
MWCNT/P1,5-DAN/Pt |
SWASV |
2.1 μM |
4 to 150 μM |
16 |
MWCNT/5-Br-PADAP/GCE |
SWASV |
100 nM |
0.9 to 114.6 μM |
17 |
G/PANI/PS nanoporous fiber/SPCE |
SWASV |
3.30 μM |
10-500 μM |
18 |
L-Cys-rGO/GCE |
DPASV |
215 nM |
0.4 to 1.2 μM |
19 |
Nafion/G/PANI nanocomposite/SPE |
SWASV |
100 nM |
1–300 μM |
20 |
Alk-Ti3C2/GCE |
SWASV |
32 nM |
0.10–0.55 μM |
32 |
Nb4C3Tx/GCE |
SWASV |
12 nM |
0.025–0.5 μM |
This work |
Selectivity, stability and repeatability
The selectivity of the Nb4C3Tx/GCE was analyzed in the presence of Cu2+ and Cd2+ as inferring agents in 5 fold excess along with Pb2+ ion. The SWASV was performed under the optimum conditions. It is found that no or negligible change in the peak current of Pb2+ in presence of interfering ions. The peak for Cu2+ at around −0.1 V,32 is present in the SWASV curve as shown in Fig. 5(a). The peak for Cd2+ at around −0.8 V,32 is not clearly visible; however, the current response is higher in the particular range where the peak for Cd2+ normally visible. Fig. 5(b) shows the current response for the individual metal ions (Pb2+, Cd2+, Cu2+) and their mixture at −0.58 V. The peak current was almost the same for Pb2+ alone and in the mixture with other interfering ions. These is no current response at particular potential of −0.58 V for the electrolyte solution containing Cd2+ or Cu2+. These results confirmed the insignificant impact of interfering metal ions and hence the selectivity of the sensor at around −0.58 V.
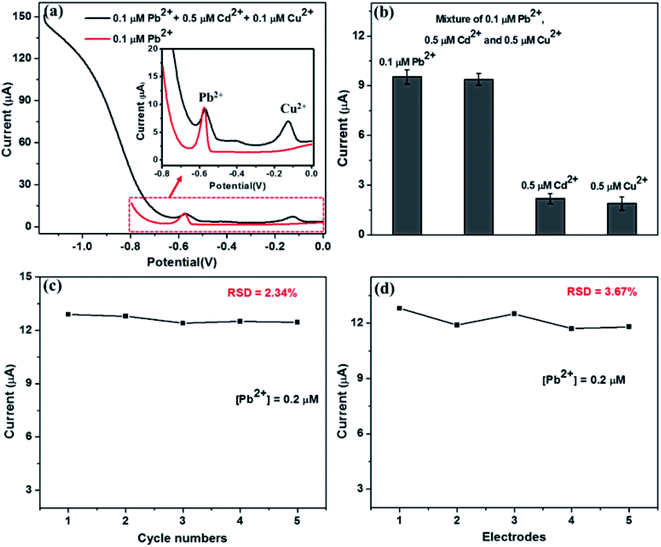 |
| Fig. 5 (a) SWASV response of the Nb4C3Tx/GCE for Pb2+ (0.1 μM) in presence of Cd2+ (0.5 μM) and Cu2+ (0.5 μM). (b) Selectivity plot-SWASV response of the Nb4C3Tx/GCE at −0.6 V for different heavy metals. (c) The stability of Nb4C3Tx/GCE – SWASV responses for 5 repetitive cyclic measurements with 0.2 μM Pb2+. (d) The repeatability of Nb4C3Tx/GCE – SWASV responses for 5 different electrodes with 0.2 μM Pb2+. | |
The stability of the Nb4C3Tx/GCE was investigated after 5 repetitive measurements using the same electrode and electrolyte containing 0.2 μM Pb2+. After every measurement, a desorption step at a potential of 0.8 V was performed before the next electrodeposition steps. It is found that the SWASV response was highly reproducible with RSD value of 2.34 (Fig. 5(c)). In addition, the stripping current of Nb4C3Tx/GCE for Pb2+ was measured after keeping the electrode at 4 °C for one week and the stripping current obtained was 94.3% of the initial current with RSD of 3.15. These results confirmed the stability of the Nb4C3Tx/GCE electrode towards the detection of Pb2+. The reproducibility analysis of the sensor was carried out by using five identical Nb4C3Tx/GCE electrodes for the detection of Pb2+ using similar procedures. The SWASV responses for five different electrodes with 0.2 μM Pb2+ showing good repeatability between different electrodes with RSD value of 3.67 (Fig. 5(d)).
Practical applications
To evaluate the practical applications of the developed Nb4C3Tx/GCE sensor, the response of the sensor has been measured after spiking the Pb2+ in bottled and tap water samples. The SWASV response of the sensor has been measured and the results are given in Table 2. The sensor exhibited a promising recovery between 95% and 102% with a relative standard deviation of 1.8–3.0%. From these results, it was confirmed that the developed sensor can be used for the detection of Pb2+ from samples.
Table 2 Recovery of Pb2+ in drinking water and tap water using the Nb4C3Tx/GCE sensor
Sample |
Pb2+ added (μM) |
Pb2+ detected (%) |
RSD (%) |
Drinking water (bottled) |
0.05 |
95.8 |
2.35 |
0.1 |
99.5 |
1.81 |
0.2 |
96.5 |
2.57 |
Tap water |
0.05 |
102.3 |
2.95 |
0.1 |
97.5 |
3.02 |
0.2 |
95.5 |
2.13 |
Conclusions
The electrochemical behaviour of Nb2CTx and Nb4C3Tx was investigated to explore its potential in electrochemical applications. The Nb4C3Tx has demonstrated promising electrochemical performance and its electrochemical response is higher than Nb2CTx. The electrochemical detection capability of Nb4C3Tx towards Pb2+ ions has been investigated by stripping analysis at optimized conditions. Evident by the high sensitivity and good reproducibility, the large interlayer spacing of Nb4C3Tx can accommodate Pb2+ ions without destroying the layered structure of the electrode. The results showed that Nb4C3Tx can be used as an immobilization platform for sensitive detection of Pb2+ with wide linear range and detection limit of 12 nM. This work validates the potential application of Nb4C3Tx for the first time towards electrochemical sensing applications.
Conflicts of interest
There are no conflicts to declare.
Acknowledgements
PAR and RPP acknowledge the financial support from the National priority research program (NPRP) grants # NPRP8-286-2-118 and # NPRP9-254-2-120 from Qatar National Research Fund (A member of Qatar Foundation), respectively. Kaitlyn Prenger at Tulane University is acknowledged for the synthesis of MXenes used in this study. The authors acknowledge M. Pasha, A. R Shetty, and J. Ponraj of Core Labs, QEERI for SEM, XRD and TEM analysis respectively.
References
- Y.-m. Cheng, H.-b. Fa, W. Yin, C.-j. Hou, D.-q. Huo, F.-m. Liu, Y. Zhang and C. Chen, J. Solid State Electrochem., 2015, 20, 327–335 CrossRef.
- F. Gao, C. Gao, S. He, Q. Wang and A. Wu, Biosens. Bioelectron., 2016, 81, 15–22 CrossRef CAS PubMed.
- T. Ndlovu, O. A. Arotiba, S. Sampath, R. W. Krause and B. B. Mamba, J. Appl. Electrochem., 2011, 41, 1389–1396 CrossRef CAS.
- M. B. Gumpu, S. Sethuraman, U. M. Krishnan and J. B. B. Rayappan, Sens. Actuators, B, 2015, 213, 515–533 CrossRef CAS.
- P. Chooto, P. Wararatananurak and C. Innuphat, ScienceAsia, 2010, 36, 150 CrossRef CAS.
- H. R. Vanaei, A. Eslami and A. Egbewande, Int. J. Pressure Vessels Piping, 2017, 149, 43–54 CrossRef CAS.
- A. Bala, M. Pietrzak, Ł. Górski and E. Malinowska, Electrochim. Acta, 2015, 180, 763–769 CrossRef CAS.
- L. Wang, Y. Wen, L. Li, X. Yang, N. Jia, W. Li, J. Meng, M. Duan, X. Sun and G. Liu, Biosens. Bioelectron., 2018, 115, 91–96 CrossRef CAS PubMed.
- P. J. Mafa, A. O. Idris, N. Mabuba and O. A. Arotiba, Talanta, 2016, 153, 99–106 CrossRef CAS PubMed.
- B. Feng, R. Zhu, S. Xu, Y. Chen and J. Di, RSC Adv., 2018, 8, 4049–4056 RSC.
- B. Wen, J. Xue, X. Zhou, Q. Wu, J. Nie, J. Xu and B. Du, ACS Appl. Mater. Interfaces, 2018, 10, 25706–25716 CrossRef CAS PubMed.
- B. Bansod, T. Kumar, R. Thakur, S. Rana and I. Singh, Biosens. Bioelectron., 2017, 94, 443–455 CrossRef CAS PubMed.
- M. R. Saidur, A. R. A. Aziz and W. J. Basirun, Biosens. Bioelectron., 2017, 90, 125–139 CrossRef CAS PubMed.
- M. Li, H. Gou, I. Al-Ogaidi and N. Wu, ACS Sustainable Chem. Eng., 2013, 1, 713–723 CrossRef CAS.
- Y. Fang, B. Cui, J. Huang and L. Wang, Sens. Actuators, B, 2019, 284, 414–420 CrossRef CAS.
- H. D. Vu, L.-H. Nguyen, T. D. Nguyen, H. B. Nguyen, T. L. Nguyen and D. L. Tran, Ionics, 2015, 21, 571–578 CrossRef CAS.
- A. Salmanipour and M. A. Taher, J. Solid State Electrochem., 2011, 15, 2695–2702 CrossRef CAS.
- N. Promphet, P. Rattanarat, R. Rangkupan, O. Chailapakul and N. Rodthongkum, Sens. Actuators, B, 2015, 207, 526–534 CrossRef CAS.
- S. Muralikrishna, K. Sureshkumar, T. S. Varley, D. H. Nagaraju and T. Ramakrishnappa, Anal. Methods, 2014, 6, 8698–8705 RSC.
- N. Ruecha, N. Rodthongkum, D. M. Cate, J. Volckens, O. Chailapakul and C. S. Henry, Anal. Chim. Acta, 2015, 874, 40–48 CrossRef CAS PubMed.
- M. Naguib, V. N. Mochalin, M. W. Barsoum and Y. Gogotsi, Adv. Mater., 2014, 26, 992–1005 CrossRef CAS PubMed.
- H. Lin, S. Gao, C. Dai, Y. Chen and J. Shi, J. Am. Chem. Soc., 2017, 139, 16235–16247 CrossRef CAS PubMed.
- O. Mashtalir, M. R. Lukatskaya, M.-Q. Zhao, M. W. Barsoum and Y. Gogotsi, Adv. Mater., 2015, 27, 3501–3506 CrossRef CAS PubMed.
- K. Rasool, R. P. Pandey, P. A. Rasheed, S. Buczek, Y. Gogotsi and K. A. Mahmoud, Mater. Today, 2019, 30, 80–102 CrossRef CAS.
- C. Tan, X. Cao, X.-J. Wu, Q. He, J. Yang, X. Zhang, J. Chen, W. Zhao, S. Han, G.-H. Nam, M. Sindoro and H. Zhang, Chem. Rev., 2017, 117, 6225–6331 CrossRef CAS PubMed.
- J. Zhu, E. Ha, G. Zhao, Y. Zhou, D. Huang, G. Yue, L. Hu, N. Sun, Y. Wang, L. Y. S. Lee, C. Xu, K.-Y. Wong, D. Astruc and P. Zhao, Coord. Chem. Rev., 2017, 352, 306–327 CrossRef CAS.
- A. Shahzad, K. Rasool, W. Miran, M. Nawaz, J. Jang, K. A. Mahmoud and D. S. Lee, ACS Sustainable Chem. Eng., 2017, 5, 11481–11488 CrossRef CAS.
- Y. Ying, Y. Liu, X. Wang, Y. Mao, W. Cao, P. Hu and X. Peng, ACS Appl. Mater. Interfaces, 2015, 7, 1795–1803 CrossRef CAS PubMed.
- A. Shahzad, M. Nawaz, M. Moztahida, J. Jang, K. Tahir, J. Kim, Y. Lim, V. S. Vassiliadis, S. H. Woo and D. S. Lee, Chem. Eng. J., 2019, 368, 400–408 CrossRef CAS.
- C. E. Ren, K. B. Hatzell, M. Alhabeb, Z. Ling, K. A. Mahmoud and Y. Gogotsi, J. Phys. Chem. Lett., 2015, 6, 4026–4031 CrossRef CAS PubMed.
- Q. Peng, J. Guo, Q. Zhang, J. Xiang, B. Liu, A. Zhou, R. Liu and Y. Tian, J. Am. Chem. Soc., 2014, 136, 4113–4116 CrossRef CAS PubMed.
- X. Zhu, B. Liu, H. Hou, Z. Huang, K. M. Zeinu, L. Huang, X. Yuan, D. Guo, J. Hu and J. Yang, Electrochim. Acta, 2017, 248, 46–57 CrossRef CAS.
- X. Ren, M. Huo, M. Wang, H. Lin, X. Zhang, J. Yin, Y. Chen and H. Chen, ACS Nano, 2019, 13, 6438–6454 CrossRef CAS PubMed.
- J. Yang, M. Naguib, M. Ghidiu, L.-M. Pan, J. Gu, J. Nanda, J. Halim, Y. Gogotsi and M. W. Barsoum, J. Am. Ceram. Soc., 2016, 99, 660–666 CrossRef CAS.
- C. Peng, P. Wei, X. Chen, Y. Zhang, F. Zhu, Y. Cao, H. Wang, H. Yu and F. Peng, Ceram. Int., 2018, 44, 18886–18893 CrossRef CAS.
- S. Zhao, X. Meng, K. Zhu, F. Du, G. Chen, Y. Wei, Y. Gogotsi and Y. Gao, Energy Storage Mater., 2017, 8, 42–48 CrossRef.
- M. Naguib, J. Halim, J. Lu, K. M. Cook, L. Hultman, Y. Gogotsi and M. W. Barsoum, J. Am. Chem. Soc., 2013, 135, 15966–15969 CrossRef CAS PubMed.
- C. Zhang, M. Beidaghi, M. Naguib, M. R. Lukatskaya, M.-Q. Zhao, B. Dyatkin, K. M. Cook, S. J. Kim, B. Eng, X. Xiao, D. Long, W. Qiao, B. Dunn and Y. Gogotsi, Chem. Mater., 2016, 28, 3937–3943 CrossRef CAS.
- Y. Xin and Y.-X. Yu, Mater. Des., 2017, 130, 512–520 CrossRef CAS.
- T. Su, R. Peng, Z. D. Hood, M. Naguib, I. N. Ivanov, J. K. Keum, Z. Qin, Z. Guo and Z. Wu, ChemSusChem, 2018, 11, 688–699 CrossRef CAS PubMed.
- J. Pang, R. G. Mendes, A. Bachmatiuk, L. Zhao, H. Q. Ta, T. Gemming, H. Liu, Z. Liu and M. H. Rummeli, Chem. Soc. Rev., 2019, 48, 72–133 RSC.
- S. Zhao, C. Chen, X. Zhao, X. Chu, F. Du, G. Chen, Y. Gogotsi, Y. Gao and Y. Dall’Agnese, Adv. Funct. Mater., 2020, 2000815 CrossRef.
- P. V. Sarma, C. S. Tiwary, S. Radhakrishnan, P. M. Ajayan and M. M. Shaijumon, Nanoscale, 2018, 10, 9516–9524 RSC.
- H. Bagheri, N. Pajooheshpour, A. Afkhami and H. Khoshsafar, RSC Adv., 2016, 6, 51135–51145 RSC.
- M. Ghidiu, M. Naguib, C. Shi, O. Mashtalir, L. M. Pan, B. Zhang, J. Yang, Y. Gogotsi, S. J. L. Billinge and M. W. Barsoum, Chem. Commun., 2014, 50, 9517–9520 RSC.
- P. A. Rasheed and N. Sandhyarani, Sens. Actuators, B, 2014, 204, 777–782 CrossRef CAS.
Footnote |
† Electronic supplementary information (ESI) available. See DOI: 10.1039/d0ra04377j |
|
This journal is © The Royal Society of Chemistry 2020 |