DOI:
10.1039/D0RA04034G
(Paper)
RSC Adv., 2020,
10, 23792-23800
Multi-omics characterization of the osmotic stress resistance and protease activities of the halophilic bacterium Pseudoalteromonas phenolica in response to salt stress†
Received
5th May 2020
, Accepted 17th June 2020
First published on 23rd June 2020
Abstract
The halophilic bacterium Pseudoalteromonas phenolica is well known as a promising candidate that enables the recycling of organic wastes at high salinity. However, for industrial applications of P. phenolica further research is required to explore the biological mechanism for maximizing the activities and productivities of this bacterium. In this study, we investigated the osmotic stress resistance and specific protease activities of P. phenolica in a normal-salt medium (0.3 M NaCl) and high-salt medium (1 M NaCl) based on intra- and extracellular multi-omics approaches. Proteins related to betaine and proline biosynthesis were increased under high salt stress. The targeted metabolite analysis found that proline was overproduced and accumulated outside the cell at high salinity, and betaine was accumulated in the cell by activation of biosynthesis as well as uptake. In addition, extracellular serine proteases were shown to be upregulated in response to salt stress by the extracellular proteomic analysis. The specific proteolytic activity assay indicated that the activities of serine proteases, useful enzymes for the recycling of organic wastes, were increased remarkably under high salt stress. Our results suggest that betaine and proline are key osmoprotectant metabolites of P. phenolica, and they can be used for the improvement of protease production and P. phenolica activities for the recycling of high-salt organic wastes in the future.
1. Introduction
Population explosion in recent years has caused large amounts of organic wastes, leading to limitation of treatment.1 As organic loading is having a tremendous effect on the environment, there is a growing interest in treating organic wastes.2 A variety of methods have been designed to recover resources in an eco-friendly manner, but bacterial treatment for recycling organic wastes is particularly highlighted due to its lower sludge generation and high capacity for substrates.3 The first phase of bacterial digestion for organic wastes is the hydrolysis of carbohydrates, lipids, and proteins.4 This process is generally a rate-limiting step in the overall bacterial waste treatment.5 Therefore, a bacterial strain with excellent hydrolytic activities is necessary for improving the efficiency of organic waste treatments.
Bacterial fermentation is ideal for treating food wastes among various types of wastes to recycle resources.6 However, food wastes from salty diets such as Korean dishes usually have high salinity, and their NaCl concentration reaches up to 1 M.7 Thus, large amounts of existing salts in the waste during bacterial fermentation reduces the activities of bacteria and their hydrolases, limiting the efficiency of waste recycling systems.8 In this respect, the discovery of a bacterial strain that can remain active in hydrolase as well as survive at high salinity is considered important for the treatment of high-salt food wastes.
P. phenolica is in the spotlight as a promising candidate that can solve these problems.9 A previous study reported that P. phenolica not only can grow under salt stress but also is exceptional for its high extracellular protease activity compared with other halophiles.9 Furthermore, P. phenolica produces proteases with halophilic, alkaliphilic and thermostable properties.10 Therefore, these features of P. phenolica are apt for the recycling of organic wastes with high salinity. However, further studies are necessary to explore the salt stress-tolerant mechanism and specific proteolytic activities of P. phenolica at high salinity prior to application on an industrial scale.
Here, we aimed to determine the differences between the protein and metabolite profiles of P. phenolica cultured in a normal-salt (0.3 M NaCl) and high-salt medium (1 M NaCl) by a multi-omics approach based on liquid chromatography–tandem mass spectrometry (LC–MS/MS). The results showed that P. phenolica overexpressed proteins related to the biosynthesis of betaine and proline, which can be used as an osmoprotectant under salt stress. Overproduction of betaine and proline was also verified by targeted metabolite quantification using multiple reaction monitoring (MRM) methods. In addition, the extracellular proteome indicates that serine proteases and metalloproteases accounted for almost all of the extracellular proteases produced by P. phenolica. Additionally, serine proteases were remarkably upregulated in response to salt stress. The specific proteolytic activity assay using protease inhibitors found that chymotrypsin activities were particularly increased in the presence of a high salt concentration. Accordingly, this study explored the biological aspects of P. phenolica such as its salt stress-tolerant mechanism and changes in protease expression under salt stress for application in recycling high-salt organic wastes.
2. Experimental
2.1. Bacterial strain and culture condition
The Pseudoalteromonas phenolica KCTC 32086 used in this research was distributed from the Korean Collection for Type Cultures (KCTC, Daejeon, South Korea). P. phenolica was cultured on marine agar (BD Difco, NJ, USA) at 25 °C. A preculture was prepared by inoculating 5 mL of marine broth (BD Difco, NJ, USA) with a single colony of P. phenolica. For cultures in the normal-salt medium, P. phenolica from preculture was diluted in 50 mL of fresh marine broth to an initial optical density at 600 nm of 0.05. For the high-salt medium, 39 g L−1 of NaCl (Junsei, Tokyo, Japan) was added to the normal marine broth. And excessive-salt medium was used the marine broth added with 97.4 g L−1 of NaCl. All liquid cultures were grown on a shaking incubator (150 rpm, 25 °C). Optical densities were measured by a UV spectrophotometer (Thermo Fisher Scientific, MA, USA).
2.2. Protein extraction and preparation
After growing to the mid-log phase in the normal-salt and high-salt medium, 45 mL of P. phenolica was centrifuged at 4000 × g for 10 minutes at 4 °C. Cell pellets were used in the preparation of intracellular proteomic analysis, and 40 mL of supernatant was prepared for the extracellular proteomic analysis. For the preparation of intracellular proteome, cell pellets were suspended with 20 mL of 0.9% w/v NaCl solution and centrifuged at the same conditions as before. Supernatants were discarded, and these washing steps were replicated once more. The washed cell pellets were re-suspended in 10 mL of 0.9% w/v NaCl solution. For the protein extraction, cell solutions were sonicated by a probe sonicator (Sonics & Materials, Inc, CT, USA) on ice and precipitated with 2 mL of 100% trichloroacetic acid. After incubation for 1 hour at 4 °C, lysed cell solutions were centrifuged, and supernatants were removed. Pellets were washed with 5 mL of ice-cold acetone twice. Residual acetone was dried at 60 °C. Pellets were re-suspended in 5 mL of 8 M urea solution. The protein concentration was measured by bicinchoninic acid assay (BCA assay; Thermo Scientific, MA, USA). A calculated volume to 500 μg of intracellular proteins was transferred to a microcentrifuge tube for the following protein preparation.
For extracellular protein preparation, 40 mL of culture supernatant was added with 8 mL of 100% trichloroacetic acid and precipitated for 2 hours at 4 °C. After centrifugation, the supernatants were removed. The pellets were washed twice with 10 mL of ice-cold acetone. Residual acetone was dried at 60 °C, and pellets were dissolved in 3 mL of 8 M urea solution. BCA assay was used for measurement of the protein concentration. Then, 200 μg of protein solution was used for the subsequent processes.
Protein was reduced using dithiothreitol (DTT
:
protein = 1
:
50, w/w) and incubated for 15 minutes at 55 °C. Protein was alkylated by treating iodoacetamide (IAM
:
protein = 1
:
10, w/w), followed by incubation in the dark and at room temperature for 15 minutes. Then, 3.5 mL of 25 mM ammonium bicarbonate buffer was added to the protein solution. Protein was digested by the addition of trypsin (trypsin
:
protein = 1
:
20, w/w) and then incubated for 16 hours at 37 °C. After digestion, the samples were purified with a C18 cartridge (Waters, MA, USA) and dried by a centrifugal vacuum concentrator (Vision Scientific, Seoul, Korea). Dried peptides were dissolved in 50 μL of 0.1% v/v formic acid solution.
2.3. Proteomic analysis by LC–MS/MS
The purified tryptic peptides were separated using an ACQUITY UPLC BEH C18 column (2.1 μm × 50 mm, 1.7 μm particle size) by Waters ACQUITY UPLC I-Class system (MA, USA). The mobile phases were 0.1% v/v formic acid in water (eluent A) and 0.1% v/v formic acid in acetonitrile (eluent B). The gradient applied was as follows: 0–3 min, 3% eluent B; 3–70 min, linear increase to 55% eluent B at 0.3 mL min−1. The injection volume was 5 μL. Mass spectrometric analysis was performed in a data-dependent mode on a Thermo Fisher Scientific LTQ Velos (CA, USA). A data-dependent analysis was performed for all LC–MS/CID runs by first acquiring an ESI mass spectrum (m/z 400–2000), the 10 most abundant ions in the ESI mass spectrum underwent CID analysis. A normalized collision energy of 35%, a q-value of 0.25, and an activation time of 30 ms was used for CID. The collected CID data were analyzed using Uniprot database and MaxQuant. They were quantified by label-free quantification (LFQ). Fold-change (>2) and p-value (<0.05) were considered significant upregulation. Fold-change (<0.5) and p-value (<0.05) were considered significant downregulation.
2.4. Osmoprotectant metabolite extraction
One milliliter of cell culture medium grown to mid-log phase in the normal and high-salt medium was centrifuged at 16
300 × g for 1 minute at 4 °C. Cell pellets were used for the extraction of intracellular metabolites, and culture supernatants were prepared for analysis of the extracellular metabolites. For extraction of intracellular metabolites, cell pellets were washed twice with 1 mL of 0.9% w/v NaCl solution. The washed pellets were re-suspended using 500 μL of ice-cold 80% v/v methanol solution, followed by incubation for 15 minutes at −70 °C. The cell lysis solution was centrifuged at 16
300 × g for 1 minute at 4 °C. Then, 350 μL of the supernatant was transferred to a new microcentrifuge tube and dried in a centrifugal vacuum concentrator. Before analysis by LC–MS/MS, dried metabolite samples were dissolved in 100 μL of 50% v/v methanol solution.
For purification of the hydrophilic extracellular metabolites, 50 μL of culture supernatant or medium control (marine broth) was mixed with 400 μL of chloroform, 200 μL of methanol and 100 μL of distilled water. The mixed solution was centrifuged at 16
300 × g for 5 minutes at 4 °C. Then, 150 μL of organic phase solution was moved into a new microcentrifuge tube and dried by a centrifugal vacuum concentrator. Dried metabolites were dissolved in 100 μL of 50% v/v methanol solution. Both intracellular and extracellular metabolites were spiked with 10 μL of 10 μg mL−1 L-phenylalanine-13C9, 15N (Sigma-Aldrich, MO, USA) for the internal standard.
2.5. Osmoprotectant metabolite analysis by LC–MRM–MS
Betaine and proline in intra/extracellular metabolites was analyzed by LC–MS/MS under multiple reaction monitoring (MRM) methods. Ten microliters of each prepared sample was analyzed by an Agilent 6420 Triple Quadrupole LC/MS (CA, USA) coupled to an Agilent 1260 Infinity Binary LC (CA, USA). Separations were performed using an Agilent Zorbax HILIC Plus column (4.6 × 100 mm, 3.5 μm) and a flow rate of 500 μL min−1. Eluent A was water containing 0.1% v/v formic acid, and B was 100% acetonitrile. The gradient applied was as follows: 0–3 min, 80% eluent B; 3–10 min, linear decrease to 20% eluent B; 10–13 min, 20% eluent B; 13–14 min, linear increase to 80% eluent B; 14–23 min, 80% eluent B. The flow rate was at 0.5 mL min−1. The capillary temperature was 300 °C, and an electrospray ionization spray voltage was used at 4 kV.
2.6. Protease activity assay
Fifteen milliliters of culture medium, grown to mid-log phase in the normal-salt and high-salt media, were centrifuged at 4000 × g for 10 minutes at 4 °C. Then, 10 mL of culture supernatants was transferred into a 10 kDa molecular weight cut-off filter and centrifuged at 4000 × g for 1 hour at 4 °C. The protease activities of the concentrated supernatants were measured based on a protocol of universal protease activity assay.11 In brief, 500 μL of 0.65% w/v casein solution was added to 50 μL of concentrated supernatant. Then, 10 μL of each protease inhibitor (3 mg mL−1 antipain, 2.4 mg mL−1 bestain, 1.8 mg mL−1 chymostain, 0.3 mg mL−1 E-64, 42 μg mL−1 pepstatin, 30 mg mL−1 Pefabloc SC and 24 mg mL−1 EDTA) was mixed with the prepared samples. To make the control sample, 10 μL of distilled water was mixed instead of the protease inhibitor. Each protease inhibitor was purchased from Sigma-Aldrich (MO, USA). The mixed solution was incubated for 10 minutes at 37 °C. The proteolytic reaction was stopped by adding 500 μL of 110 mM TCA solution. Following incubation for 10 minutes at 37 °C, the samples were filtered using a 0.45 μm polyethersulfone syringe filter (Hyundai Micro, Seoul, Korea). Then, 200 μL of filtrate was moved into a new microcentrifuge tube. The samples were added to 500 μL of a 0.5 M sodium carbonate buffer and 100 μL of 0.5 M Folin & Ciolcaltea's phenol followed by incubation for 30 minutes at 37 °C. The samples were filtrated by a 0.45 μm polyethersulfone syringe filter. Finally, each filtrate of the samples was transferred to 96-well plates, and the optical density (660 nm) was measured using a UV spectrophotometer.
2.7. Statistical analysis
Student's t-test, an analysis of variance (ANOVA) and Fisher's least significant difference (LSD) tests were conducted using the R statistical programming environment.
3. Results & discussion
3.1. Growth of P. phenolica under salt stress
The ability to grow under salt stress was tested. P. phenolica was grown in a normal-salt (0.3 M NaCl), high-salt (1 M NaCl) and excessive-salt (2 M NaCl) medium; 0.3 M NaCl is the original NaCl concentration of the marine broth that was used for culturing P. phenolica, and 1 M NaCl is the maximum NaCl concentration of food waste from high-salt diets such as Korean dishes. Although P. phenolica was not grown in the excessive-salt medium at all, typical bacterial growth was observed in the high-salt medium and normal-salt medium (Fig. 1). The specific growth rate of P. phenolica in the normal-salt medium was measured at 0.043 h−1, 1.5-fold higher compared with that of the high-salt medium (0.029 h−1). P. phenolica did not grow in the high-salt medium as fast as in the normal-salt medium, but it showed typical bacterial growth curves. This result means that P. phenolica can maintain its biological activities under high salinity, and it can be applied to the recycling of high-salt organic wastes in the future.12,13 Therefore, based on the growth patterns according to each salt condition, this study aimed to compare the biological phenotypes according to salt stress using multi-omics approaches.
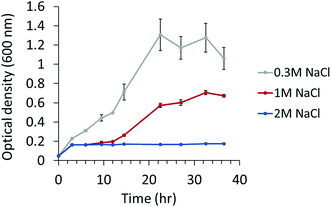 |
| Fig. 1 Growth of P. phenolica in marine broth with normal (0.3 M NaCl), high-salt (1 M NaCl) and excessive-salt concentration (2 M NaCl). Each experiment performed in triplicates. | |
3.2. Proteomic changes of P. phenolica grown in response to salt stress
We investigated the proteomic changes in P. phenolica under salt stress based on a proteomic approach using LC–MS/MS. Protein samples were collected at the mid-log phase during the growth of P. phenolica in each normal and high-salt medium and subjected to LFQ. Overall, 640 proteins were identified in the intracellular protein analysis, and 102 proteins among all identified intracellular proteins were significantly upregulated (fold-change > 2, p < 0.05) in the samples from the high-salt medium compared with the normal-salt medium (Fig. 2A and Table S1†). Conversely, 71 proteins were significantly downregulated (fold-change < 0.5, p < 0.05). In the extracellular protein analysis, 633 proteins were identified, of which 46 proteins were significantly upregulated (fold-change > 2, p < 0.05) in the samples from the high-salt medium and 61 proteins were significantly downregulated (fold-change < 0.5, p < 0.05) (Fig. 2B and Table S2†). Both the intra- and extracellular protein analysis showed that proteins related to the proline biosynthetic pathway were overexpressed in the samples from the high-salt medium. Furthermore, a protein involved in proline catabolism was decreased in the samples from the high-salt medium. These results mean that proline produced by P. phenolica was accumulated and less degraded under salt stress. Proline not only is an amino acid used for constituting proteins but also has an osmoprotective function.14 In accordance with these results, this study focused on the osmoprotectant biosynthetic pathway of P. phenolica.
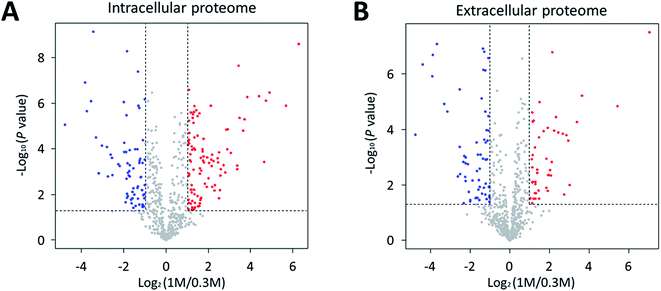 |
| Fig. 2 Volcano plot of differentially expressed proteins in response to salt stress. Volcano plots under (A) intracellular proteome and (B) extracellular proteome indicating the up-regulating proteins of high-salt condition (1 M NaCl) in red circles and down-regulating proteins in blue circles. | |
3.3. Osmoprotectant expression of P. phenolica under salt stress
In the proteomic analysis, the proteins in the proline biosynthetic pathway were remarkably upregulated in the high-salt medium (Table 1). The gamma-glutamyl phosphate reductase and glutamate 5-kinase involved in the L-proline biosynthesis pathway were increased in both the intra- and extracellular proteome from the cultures at high salinity. Moreover, the expression of bifunctional protein PutA functioning in proline catabolism was downregulated in the samples from the high-salt medium compared with those of the normal-salt medium. This study conducted an MRM method-based metabolite analysis by LC–MS/MS to verify the proline overexpression at high-salinity. The analysis of intracellular metabolites indicated that the intracellular proline of P. phenolica cultured in the high-salt medium increased 13.3-fold compared with in the normal-salt medium (Fig. 3A). Extracellular proline was 19.2-fold upregulated in the high-salt medium (Fig. 3B). A multi-omics approach found that P. phenolica enhanced the proline biosynthetic pathway under salt stress and secreted proline out of the cell. Proline synthesis is increased by a variety of Gram-positive bacteria under osmotic stress, and proline accumulates to high intracellular concentrations.15 Meanwhile, Gram-negative bacteria, especially the Enterobacteriaceae family, tend to accumulate proline by enhanced transport to take up exogenous proline.16 P. phenolica overproduced proline and its biosynthetic enzymes in addition to accumulating it out of the cell in response to osmotic stress despite being a Gram-negative bacterium. A causal relationship between intracellular proline accumulation and salt tolerance has long been demonstrated in bacteria, and exogenous proline has been reported to improve the growth or activities of bacteria.17 Thus, proline synthesis under salt stress is thought to be a pivotal mechanism of osmoadaptation in P. phenolica, unlike other Gram-negative bacteria.
Table 1 Identified proteins of P. phenolica involved in the osmoprotectant metabolism (N/S, not significant)
Unitprot accession |
Protein name |
Function |
Cellular location |
Fold change (high-salt/normal-salt) |
p value |
A0A0S2K0C8 |
Glycine betaine aldehyde dehydrogenase |
Betaine biosynthesis |
Intracellular |
1.84 |
NS |
Extracellular |
4.33 |
0.001 |
A0A0S2K6P1 |
Glutamate 5-kinase |
Proline biosynthesis |
Intracellular |
2.82 |
0.01 |
Extracellular |
4.94 |
<0.0001 |
A0A0S2K703 |
Gamma-glutamyl phosphate reductase |
Proline biosynthesis |
Intracellular |
76.93 |
<0.0001 |
Extracellular |
129.67 |
<0.0001 |
A0A0S2JZ14 |
Bifunctional protein PutA |
Proline catabolism |
Intracellular |
0.04 |
<0.0001 |
Extracellular |
0.04 |
0.0002 |
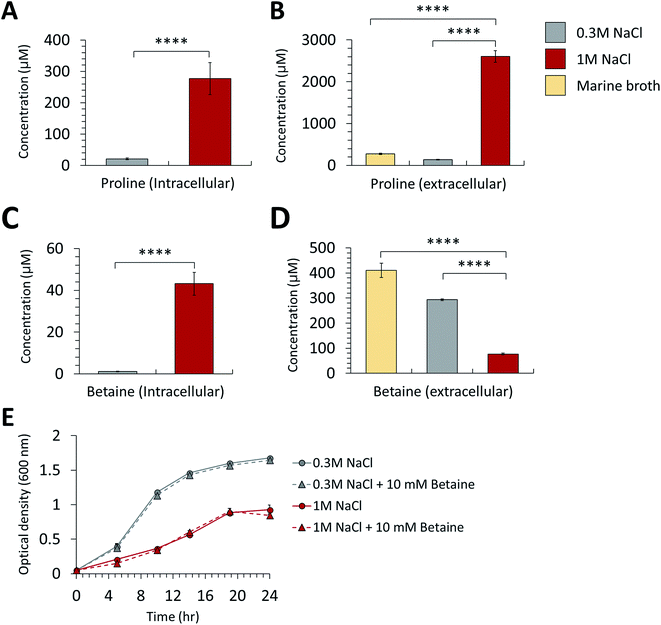 |
| Fig. 3 Comparison of intracellular (A) betaine and (B) proline concentration in the normal-salt (0.3 M NaCl) and high-salt (1 M NaCl) conditions. Comparison of extracellular (C) betaine and (D) proline concentration in the normal, high-salt conditions and marine broth control. (E) Growth changes of P. phenolica in the normal and high-salt medium according to adding 10 mM of betaine to each medium (****p value < 0.0001). Each experiment tested in triplicates. | |
In addition to proline, we observed the differential expression of betaine and its synthetic enzyme at the metabolite and protein levels under salt stress. Glycine betaine aldehyde dehydrogenase, a betaine biosynthetic enzyme, was increased in both the intra- and extracellular proteome of the high-salt medium compared with the normal-salt medium (Table 1). Additionally, the metabolite analysis based on MRM methods indicated that intracellular betaine was overproduced under salt stress, but extracellular betaine was decreased over the medium control (marine broth) and normal-salt medium (Fig. 3C and D). Our results suggest that P. phenolica accumulates betaine in the cell through uptake as well as overproduction to resist salt stress (Fig. 4). In contrast, with proline, however, P. phenolica was shown to absorb more exogenous betaine than was secreted out of the cell. This tendency is similar to the osmoprotective mechanism of other bacteria.18 Osmoprotectant metabolites serve to increase osmotic pressure in the cytoplasm, and they can also stabilize proteins and membranes under salt stress.19,20 Thus, enhancing the osmoprotectant biosynthetic pathway is enormously helpful in maintaining the bioactivities of organisms at high salinity. Actually, in the case of industrial applications under the needed salt stress-tolerant bacteria, productivities have been increased by the improvement of osmoprotectant biosynthesis using genetic manipulation.21 Based on this knowledge, if P. phenolica were to be designed to maximize growth and bioactivities at high salinity by genetic engineering in proline and betaine biosynthesis, its availability is expected to increase as an industrial strain for the recycling of high-salt organic wastes.
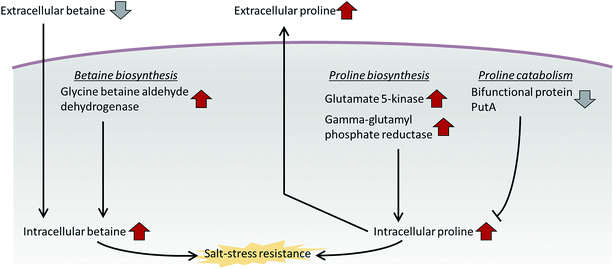 |
| Fig. 4 Salt resistance mechanism of P. phenolica using the betaine and proline biosynthetic pathways. | |
We measured the growth difference of P. phenolica between culturing in normal and high-salt media with supplementation of 10 mM betaine to verify how excessive exogenous betaine is helpful in improving growth (Fig. 3E). However, the excessive treatment of betaine did not affect the growth of P. phenolica. These characteristics are consistent with the previous report showing that E. coli did not improve its growth above a certain level at high salinity despite the excessive betaine added to the medium.22 Along with the results of the multi-omics analysis, P. phenolica was shown to uptake extracellular betaine under salt stress but was limit to enhance the growth according to the betaine concentration. Hence, this study could be a basis for the industrial application of P. phenolica at high salinity to supplement suitable amounts of betaine.
3.4. Changes in extracellular protease composition and proteolytic activities in response to salt stress
To determine the expression changes of extracellular proteases in P. phenolica under salt stress, we compared the protein profiles of extracellular proteases in the normal and high-salt culture media, for which 38 and 36 proteases were detected in the samples, respectively (Table 2). Among all detected proteases, 20 and 18 proteases were identified as metalloprotease groups and 13 and 14 proteases were identified as serine protease groups in the normal and high-salt culture media, respectively. Metalloproteases and serine proteases accounted for almost all of the proteases of P. phenolica. In the case of metalloproteases, peptidase M14 and M48 family peptidase were only detected in the normal-salt culture medium, but most of the proteases were not significantly changed according to salt stress (fold-change > 2, p < 0.05). With respect to serine proteases, 5 proteases (signal peptidase I, tricorn protease homolog, periplasmic serine endoprotease DegP-like peptidase S46, carboxypeptidase and peptidase S9) were significantly upregulated in the high-salt culture medium, and signal peptidase I was only identified in the high-salt culture medium. Thus, the LC–MS/MS-based extracellular proteomic analysis indicated that the expression of serine proteases in P. phenolica was significantly increased over other protease groups in response to salt stress. Extracellular proteases originating from P. phenolica have high catalytic activities compared with those of other bacteria, and they are reported to be worth applying on industrial strains for recycling high-salt organic wastes because of their halophilic, alkaliphilic and thermostable properties.9,10 Proteases are classified under several catalytic types, and then their functions and industrial applications are different according to their catalytic types.23 Therefore, determining the compositions and expression profiles of extracellular proteases is essential for the appropriate application of P. phenolica as a protease-producing industrial strain. We found that P. phenolica overexpressed serine proteases under salt stress using the extracellular proteomic analysis. Serine proteases are valuable in the recycling of food wastes because several useful proteases such as trypsin and chymotrypsin belong to the serine protease group.24 Hence, these results suggest that the characteristics of P. phenolica related to overexpression of serine proteases under salt stress are suited to the industrial application of recycling high-salt food wastes.
Table 2 Expression changes of identified extracellular proteases in P. phenolica under salt stress (ND, not detected; NS, not significant)
Group |
Uniprot accession |
protein name |
Fold change (high-salt/normal-salt) |
p value |
Metalloprotease |
A0A0S2JXA1 |
Xaa-Pro dipeptidase |
1.78 |
0.0004 |
A0A0S2K3H8 |
Peptidase M17, leucyl aminopeptidase domain-containing protein |
1.74 |
0.003 |
A0A0S2K1Y7 |
Probable cytosol aminopeptidase |
1.68 |
<0.0001 |
A0A0S2JZ46 |
Aminopeptidase B |
1.56 |
<0.0001 |
A0A0S2K1B1 |
ATP-dependent zinc metalloprotease FtsH |
1.46 |
NS |
A0A0S2K1X2 |
Peptidase M13 |
1.43 |
0.006 |
A0A0S2K958 |
Leucyl aminopeptidase |
1.42 |
0.01 |
A0A0S2JZ16 |
Peptidase M16 |
1.41 |
NS |
A0A0S2K135 |
Peptidase M28 |
1.39 |
NS |
A0A0S2K236 |
Peptidase M14, carboxypeptidase A |
1.37 |
NS |
A0A0S2K2G0 |
Proline dipeptidase |
1.35 |
NS |
A0A0S2K3K3 |
Peptidase M16 |
1.23 |
NS |
A0A0S2K5F2 |
Probable cytosol aminopeptidase |
0.98 |
NS |
A0A0S2K4W4 |
Oligopeptidase A |
0.95 |
NS |
A0A0S2K6B9 |
Aminopeptidase |
0.92 |
NS |
A0A0S2K4H5 |
ATP-dependent Clp protease ATP-binding subunit ClpX |
0.90 |
NS |
A0A0S2K0T8 |
Peptidase M3 |
0.64 |
NS |
A0A0S2K1S1 |
Aminopeptidase YpdF (MP-, MA-, MS-, AP-, NP-specific) |
0.48 |
NS |
A0A0S2K4X4 |
Peptidase M14, carboxypeptidase A |
ND in high-salt samples |
|
A0A0S2K2T5 |
M48 family peptidase |
ND in high-salt samples |
|
Serine protease |
A0A0S2K315 |
Signal peptidase I |
ND in normal-salt samples |
|
A0A0S2K7Y2 |
Tricorn protease homolog |
12.32 |
<0.0001 |
A0A0S2JYI3 |
Periplasmic serine endoprotease DegP-like |
10.41 |
<0.0001 |
A0A0S2JZP6 |
Peptidase S46 |
6.37 |
0.0001 |
A0A0S2K4K5 |
Carboxypeptidase |
3.57 |
0.001 |
A0A0S2K6T8 |
Peptidase S9 |
2.58 |
0.003 |
A0A0S2K558 |
Dipeptidyl peptidase IV |
1.82 |
0.02 |
A0A0S2K209 |
Carboxy-terminal protease |
1.63 |
0.03 |
A0A0S2K930 |
Peptidase S9 |
1.52 |
<0.0001 |
A0A0S2K2R5 |
Acyl-peptide hydrolase |
1.41 |
NS |
A0A0S2K1Z0 |
Peptidase |
1.08 |
NS |
A0A0S2K479 |
ATP-dependent Clp protease proteolytic subunit |
0.89 |
NS |
A0A0S2JXY2 |
Carboxyl-terminal protease |
0.59 |
NS |
A0A0S2K7G7 |
Peptidase S8/S53 subtilisin kexin sedolisin |
0.20 |
0.001 |
Other protease |
A0A0S2K7B7 |
Putative stomatin/prohibitin-family membrane protease subunit YbbK |
1.77 |
NS |
A0A0S2K8N7 |
Putative protease with the C-terminal PDZ domain protein |
1.15 |
NS |
A0A0S2K1A5 |
Glutamate carboxypeptidase II |
0.84 |
NS |
A0A0S2K5T7 |
ATP-dependent protease ATPase subunit HslU |
0.64 |
NS |
A0A0S2K0S2 |
Isoaspartyl peptidase |
ND in high-salt samples |
|
Additionally, this study monitored the changes in actual proteolytic activities of P. phenolica in response to salt stress. We measured the protease activities of the culture medium of P. phenolica incubated in a normal and high-salt medium and observed decreases of specific proteolytic activities according to treating a variety of protease inhibitors. First, the extracellular proteolytic activity of the high-salt culture medium was increased 4.1-fold over the normal-salt culture medium when not treating protease inhibitors (Fig. 5A). Moreover, the proteolytic activities treated with each protease inhibitor were normalized to vehicle-treated protease activities, and the amount of proteolytic activity relatively decreased for each protease inhibitor was measured (Fig. 5B). This method can compare the activities of each protease family under salt stress by analyzing the degree of activity decrease according to each protease inhibitor.25 As a result, the proteolytic activities of the serine and metalloprotease groups amounted to the majority of the overall proteolytic activity. This result is similar to the expression profiles of the extracellular proteases analyzed by LC–MS/MS-based proteomics. In addition, we observed significant changes in the treatments of 4 protease inhibitors (Antipain, Chymostatin, Bestatin, and Pepstatin). Meanwhile, the treatments of antipain, bestatin, and pepstatin did not decrease the protease activities more than 2-fold in the high-salt culture medium compared with the normal-salt culture medium; the treatment of chymostatin reduced the protease activity 25.7-fold. The function of chymostatin is the inhibition of various types of chymotrypsin among serine proteases. Thus, these results showed that the proteolytic activity of chymotrypsin is increased when P. phenolica is at high salinity. Hence, this study indicated that P. phenolica enhances the expression and activities of serine proteases, especially the chymotrypsin family, at high salinity using the analysis of extracellular proteome and specific proteolytic activities. As previously stated, serine proteases are considered valuable enzymes for the conversion of waste to useful biomass in waste treatment.24 In particular, chymotrypsin as a family of serine proteases is a type of digestive enzyme, and it has been used in various industries such as food applications.26 Therefore, this study suggests that P. phenolica could be used as an industrial strain for food waste treatment needing proteolytic activities at high salinity in the future because it has high proteolytic activities from serine proteases as well as an increase in overall proteolytic activities under salt stress.
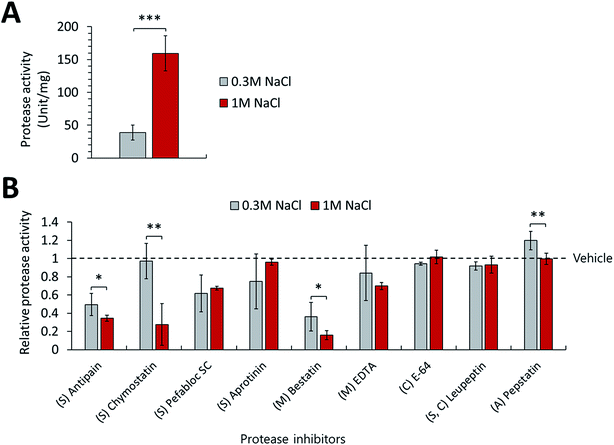 |
| Fig. 5 (A) Comparison of proteolytic activities in the normal-salt (0.3 M NaCl) and high-salt (1 M NaCl) culture medium. (B) Relative proteolytic activities in the normal and high-salt culture medium according to treating each protease inhibitor (S, serine protease inhibitor; M, metalloprotease inhibitor; C, cysteine protease inhibitor; A, aspartate protease inhibitor; *p value < 0.05, **p value < 0.01, ***p value < 0.001). Each experiment performed in triplicates. | |
3.5. Other significant proteins
We also observed expression changes in other major proteins according to salt stress. OmpA domain-containing protein, Tol-Pal system protein TolB and peptidoglycan-associated protein (Pal) were upregulated in the samples of the high-salt medium compared with the normal-salt medium (Table 3). These proteins contribute to the outer membrane integrity of P. phenolica, and they are reported to improve the outer membrane integrity of other bacteria under salt stress.27,28 The osmotic shock changes the compositions of fatty acids and phospholipids in the bacterial membrane, leading to damage to the outer membrane integrity.29 Thus, overexpression of these proteins was shown to restore the outer membrane integrity of P. phenolica at high salinity. If these proteins are overexpressed or enhanced by genetic manipulation in P. phenolica, an industrial strain that has further tolerance under salt stress could be developed.
Table 3 The differentially expressed proteins related to the outer membrane integrity in response to salt stress (NS, not significant)
Unitprot accession |
Protein name |
Function |
Cellular location |
Fold change (high-salt/normal-salt) |
p value |
A0A0S2K429 |
OmpA domain-containing protein |
Outer membrane integrity |
Intracellular |
51.38 |
<0.0001 |
Extracellular |
43.04 |
<0.0001 |
A0A0S2K380 |
Tol-Pal system protein TolB |
Outer membrane integrity |
Intracellular |
13.08 |
<0.0001 |
Extracellular |
3.55 |
0.01 |
A0A0S2K2C8 |
Peptidoglycan-associated protein |
Outer membrane integrity |
Intracellular |
2.09 |
0.001 |
Extracellular |
1.30 |
NS |
4. Conclusions
This study explored the resistance mechanism of P. phenolica for osmotic stress using an LC–MS/MS-based multi-omics analysis prior to industrial application for recycling high-salt organic wastes in the future. First, we showed that P. phenolica maintained the typical bacterial growth profile under salt stress. This study confirmed that P. phenolica activated the osmoprotectant synthetic pathways related to producing betaine and proline at high salinity. Furthermore, we supposed that proline would secreted and accumulated outside the cell as well as overproduced in the cell under salt stress, but betaine was accumulated in the cell through uptake of exogenous betaine by the LC–MS/MS based metabolic analysis. In addition, this study showed that the expression of serine proteases and the proteolytic activities of P. phenolica were increased under salt stress through the extracellular proteomic analysis and specific proteolytic activity assay. This study indicates that a better understanding of the osmoprotective mechanism and protease activities of P. phenolica may be helpful in its application as an industrial strain for recycling high-salt organic wastes.
Conflicts of interest
The authors have no conflict of interest to declare.
Acknowledgements
This work was supported by the Basic Science Research Program through the National Research Foundation of Korea (NRF-2015M1A5A1037196, NRF-2018R1D1A1B07048185, NRF-2019M2C8A2058418, NRF-2017M3A9E4077235).
References
- P. W. Westerman and J. R. Bicudo, Bioresour. Technol., 2005, 96, 215–221 CrossRef CAS PubMed.
- S. Tafdrup, Biomass Bioenergy, 1995, 9, 303–314 CrossRef CAS.
- A. Khalid, M. Arshad, M. Anjum, T. Mahmood and L. Dawson, Waste Manage., 2011, 31, 1737–1744 CrossRef CAS.
- M. D. Kim, M. Song, M. Jo, S. G. Shin, J. H. Khim and S. Hwang, Appl. Microbiol. Biotechnol., 2010, 85, 1611–1618 CrossRef CAS PubMed.
- D. Kondusamy and A. S. Kalamdhad, J. Environ. Chem. Eng., 2014, 2, 1821–1830 CrossRef.
- J. B. Holm-Nielsen, T. Al Seadi and P. Oleskowicz-Popiel, Bioresour. Technol., 2009, 100, 5478–5484 CrossRef CAS PubMed.
- J. Y. Seo, J. S. Heo, T. H. Kim, W. H. Joo and D. M. Crohn, Waste Manage., 2004, 24, 981–987 CrossRef CAS PubMed.
- J.-J. Lee, R.-D. Park, Y.-W. Kim, J.-H. Shim, D.-H. Chae, Y.-S. Rim, B.-K. Sohn, T.-H. Kim and K.-Y. Kim, Bioresour. Technol., 2004, 93, 21–28 CrossRef CAS PubMed.
- J. Johnson, P. D. V. N. Sudheer, Y.-H. Yang, Y.-G. Kim and K.-Y. Choi, Biotechnol. Bioprocess Eng., 2017, 22, 450–461 CrossRef CAS.
- J. Johnson, Y.-H. Yang, D.-G. Lee, J.-J. Yoon and K.-Y. Choi, Protein Expression Purif., 2018, 152, 46–55 CrossRef CAS PubMed.
- C. Cupp-Enyard, J. Visualized Exp., 2008, e899 Search PubMed.
- T. Tanaka, J. Burgess and P. Wright, Appl. Microbiol. Biotechnol., 2001, 57, 200–204 CrossRef CAS PubMed.
- W. Zhang, L. Chen and D. Liu, Appl. Microbiol. Biotechnol., 2012, 93, 1305–1314 CrossRef CAS PubMed.
- S. Cayley, B. A. Lewis and M. T. Record, J. Bacteriol., 1992, 174, 1586–1595 CrossRef CAS PubMed.
- A. Zaprasis, M. Bleisteiner, A. Kerres, T. Hoffmann and E. Bremer, Appl. Environ. Microbiol., 2015, 81, 250 CrossRef PubMed.
- D. Paul, J. Basic Microbiol., 2013, 53, 101–110 CrossRef CAS PubMed.
- G. He, C. Wu, J. Hunag and R. Zhou, J. Microbiol. Biotechnol., 2017, 27, 1681–1691 CrossRef CAS PubMed.
- M. Tsuzuki, O. V. Moskvin, M. Kuribayashi, K. Sato, S. Retamal, M. Abo, J. Zeilstra-Ryalls and M. Gomelsky, Appl. Environ. Microbiol., 2011, 77, 7551–7559 CrossRef CAS PubMed.
- Z. Ignatova and L. M. Gierasch, Proc. Natl. Acad. Sci. U. S. A., 2006, 103, 13357–13361 CrossRef CAS PubMed.
- P. Subramanian and J. Gurunathan, Appl. Biochem. Biotechnol., 2020, 190, 391–409 CrossRef CAS PubMed.
- S. D. McNeil, M. L. Nuccio and A. D. Hanson, Plant Physiol., 1999, 120, 945–949 CrossRef CAS PubMed.
- B. Perroud and D. Le Rudulier, J. Bacteriol., 1985, 161, 393–401 CrossRef CAS PubMed.
- S. Li, L. Wang, J. Yang, J. Bao, J. Liu, S. Lin, J. Hao and M. Sun, J. Sep. Sci., 2016, 39, 2050–2056 CrossRef CAS PubMed.
- M. Sharma, Y. Gat, S. Arya, V. Kumar, A. Panghal and A. Kumar, Ind. Biotechnol., 2019, 15, 69–78 CrossRef CAS.
- Y.-G. Kim, A. M. Lone, W. M. Nolte and A. Saghatelian, Proc. Natl. Acad. Sci. U. S. A., 2012, 109, 8523–8527 CrossRef CAS PubMed.
- N. F. Haard, J. Aquat. Food Prod. Technol., 1992, 1, 17–35 CrossRef CAS.
- U. Choi and C.-R. Lee, Front. Microbiol., 2019, 10, 953 CrossRef PubMed.
- R. Lloubès, E. Cascales, A. Walburger, E. Bouveret, C. Lazdunski, A. Bernadac and L. Journet, Res. Microbiol., 2001, 152, 523–529 CrossRef.
- A. Gandhi and N. P. Shah, J. Dairy Sci., 2016, 99, 2594–2605 CrossRef CAS PubMed.
Footnotes |
† Electronic supplementary information (ESI) available. See DOI: 10.1039/d0ra04034g |
‡ These authors contributed equally to this work. |
|
This journal is © The Royal Society of Chemistry 2020 |
Click here to see how this site uses Cookies. View our privacy policy here.