DOI:
10.1039/D0RA03905E
(Paper)
RSC Adv., 2020,
10, 26874-26879
A mitochondrial-targetable dual functional near-infrared fluorescent probe to monitor pH and H2O2 in living cells and mice†
Received
30th April 2020
, Accepted 29th June 2020
First published on 17th July 2020
Abstract
A lower pH level and high hydrogen peroxide (H2O2) concentration in mitochondria is closely associated with a variety of diseases including cancer and inflammation. Thus, determination of changes in the level of acidic pH and H2O2 is of great importance and could provide new insights into the key functions under both physiological and pathological conditions. Herein, we present a novel mitochondria-targetable probe NIR-pH-H2O2, as the first near infrared (NIR) fluorescent small molecule, to monitor changes of endogenous pH (pka = 6.17) and H2O2 with high sensitivity, good compatibility and low cytotoxicity. Futhermore, it was successfully employed to monitor pH and H2O2 in a mouse acute inflammation model. These results demonstrate that NIR-pH-H2O2 is a novel bifunctional mitochondrial-targeted NIR probe to sense acidic pH and H2O2 in vitro and in vivo, indicating its huge potential for the diagnosis of pH and H2O2-related diseases.
Introduction
Intracellular pH is an important parameter in mediating many physiological processes such as cell metabolism, proliferation, apoptosis, ion transport and homeostasis and so on.1–4 However, abnormal intracellular pH is associated with cellular dysfunctions such as cancer and neurodegenerative disorders.5,6 Hydrogen peroxide (H2O2), an important member of reactive oxygen species (ROS) mediates signal cascades involved in redox homeostasis.7 Substantial evidence has showed that excessive H2O2 is relevant to serious diseases including cardiovascular disorders, neurodegenerative diseases, cancer and diabetes.8–13 Wang et al. monitored low pH and high H2O2 in cancer cells, using dual responsive supramolecular polymer by the host–guest interactions of β-CD-hydrazone-DOX and PEG-Fc.14 Colon cancer cells were extremely sensitive to pH reduction, which naturally cause metabolic stress in the colon lumen, as well as H2O2 and ionizing radiation.15 A trend for reduced pH and increased H2O2 in lower airway inflammation and chronic obstructive pulmonary disease was observed.16,17 Mitochondria are cellular powerhouses that produce energy through the respiratory chain.18 Abnormal levels of pH and H2O2 in the mitochondria are associated with various diseases like cancer, Alzheimer's disease and cardiovascular diseases.19–22 Thus, determination of mitochondrial changes in pH and level of H2O2 is of great importance and could provide new insights into the key functions of mitochondria under both physiological and pathological conditions.
Recently, Zhou et al. developed a type of two-photon dyes with D–π–A–π–D structure, which possesses near-infrared (NIR) properties, large two-photon absorption cross-section ∼160 G and high fluorescence quantum yield ∼0.15.23 The effect of pH on this type of dyes had been studied, pH changes had significant effect on this kind of dyes fluorescence intensity, speculating that in a strong base condition the oxygen-bridge bond could be cut off and in acidic medium recovered easily. To the best of our knowledge, we firstly propose that oxygen-bridge group could be appropriate for the development of acidic pH fluorescent probes. In fluorescent detection field, boric acid group has widely been used as the recognition site toward H2O2.24–26 The chemo-selective deprotection of boric acid to phenols provides a useful reaction-based method which detects H2O2 over other ROS.27 Additionally, phenylboronic acid (pKa = 8.70) with relatively weak Lewis acidity is more stability to different pH.28 Moreover, carbonate as the self-immolative system was applied as a linker of probe which can release CO2 through a 1,6-benzyl elimination.29,30
In order to study the biological function or harmful effects of pH and H2O2 in mitochondria, we present the first mitochondria-immobilized NIR bifunctional fluorescent probe NIR-pH-H2O2 for monitoring pH and H2O2 (Table S1†). As illustrated in Scheme 1, NIR-pH-H2O2 consists of TP-NIR-OH fluorophore, phenylboronic acid and carbonate linker. The TP-NIR-OH fluorophore was predicted to display a NIR fluorescence “off–on” signal response to acidic pH and its positively charged was expected to display mitochondria-specific staining capability. Phenylboronic acid moiety was oxidized by H2O2 to release CO2 through a 1,6-benzyl elimination, resulting in the recovery of fluorescence. It was successfully applied for image of pH and H2O2 in mitochondria of live cells and monitoring inflammation in living mice. The present results strongly demonstrated that NIR-pH-H2O2 would serve as an excellent bifunctional fluorescent probe to monitor mitochondrial pH and H2O2 fluctuations in living biological samples.
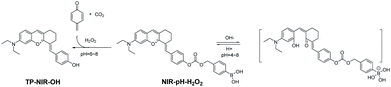 |
| Scheme 1 Proposed reaction mechanism of NIR-pH-H2O2 towards pH and H2O2. | |
Experimental section
Materials and instruments
Absorption spectra measurements were performed on a Shimadzu UV-vis spectrophotometer, UV-2550. Fluorescent spectra measurements were performed on a Thermo Scientific Lumina fluorescence spectrophotometer. The pH was carried out by a PB 10 digital pH meter. 1H NMR (400 MHz) and 13C NMR (125 MHz) spectra were measured on a Bruker ACF-300 spectrometer (Bruker Corp., Billerica, MA, USA) using TMS as internal reference. Mass spectra was carried on an Agilent 1100 Series (Agilent Technologies, Santa Clara, CA, USA) LC/MSD high performance ion trap mass spectrometer and a Mariner ESI-TOF spectrometer. All starting materials and reagents were purchased from commercial sources and used without further purification. Deionized water was prepared through a water purification system. Fluorescent microscopy imaging was carried out by a confocal laser scanning microscope (CLSM, LSM700, Zeiss, Germany). Fluorescent images of mice were taken by an IVIS Spectrum.
General procedure for fluorometric analysis
A stock solution of NIR-pH-H2O2 (1 mM) was prepared in DMSO. Stock solutions of H2O2 and other various analytes were prepared with distilled water freshly. A series of standard pH buffer solutions with different values were prepared by the standard procedures. Before absorption and fluorescence spectral measurements, all the resulting solutions were shaken well and incubated for 60 min at room temperature, all fluorescence measurements were executed with the excitation wavelength of 560 nm and the emission wavelength of 680 nm unless other noted.
The pKa values of NIR-pH-H2O2
The pKa values of NIR-pH-H2O2 were calculated using the Henderson–Hasselbalch equation: log[(Imax − I)/(I − Imin)] = pH − pKa. Where I was the observed fluorescence intensity, Imax and Imin were the corresponding maximum and minimum fluorescence intensity, respectively.31
Cytotoxicity assay and fluorescence imaging toward pH and H2O2 in living cells
The cytotoxic effect of NIR-pH-H2O2 was assessed by the MTT assay. After cells attachment, MCF-7 and Hela cell lines pretreated in medium with NIR-pH-H2O2 at different concentrations. 200 μL DMSO was added to each well and shaken for 20 min. The absorbance was measured at 490 nm with a microplate reader (Tecan US, Inc. USA). Cell viability was calculated using the following equation:
Cell viability (%) = optical density value (treatment group)/optical density value (control group) × 100%. |
Before imaging, cells were passed and seeded in glass dishes. For labeling, the cells were incubated with 10 μM of NIR-pH-H2O2 for the specific incubation time. Then, cells were washed three times with PBS (phosphate buffered saline). Cell images were then recorded using a confocal laser scanning microscope (CLSM, LSM700, Zeiss, Germany) and processed using the ZEN imaging software. Other information is available in the figure captions.
Animal models and in vivo animal studies
Athymic nude mice were purchased from Qinglongshan Animal Breeding Farm (Nanjing, China) for in vivo imaging studies. All animal procedures were performed in accordance with the Guidelines for Care and Use of Laboratory Animals of China Pharmaceutical University and the experiments were approved by the Animal Ethics Committee of China Pharmaceutical University.
Before animal imaging, nude mice were given an intraperitoneal injection of lipopolysaccharide (2 mg mL−1) in 100 μL PBS for group A. NIR-pH-H2O2 (250 μM) in 200 μL buffered solution was injected in the same way for group B. Lipopolysaccharide (2 mg mL−1) in 100 μL PBS buffer solution was injected intraperitoneally firstly. After 12 h, NIR-pH-H2O2 (250 μM) in 200 μL of buffered solution was injected at the same region for group C. All fluorescence images were acquired with auto exposure (IVIS Spectrum) after incubation for 30 min by using the NIR fluorescence imaging system (excitation wavelength = 560 nm, emission wavelength = 680 nm).32
Synthesis of NIR-pH-H2O2
The probe NIR-pH-H2O2 was synthesized by the procedure shown in Fig. S1.† TP-NIR-OH and B1 was synthesized according to the reported methods.23,30
TP-NIR-OH (62 mg, 0.2 mmol), compound B1 (80 mg, 0.2 mmol) and K2CO3 (65 mg, 0.6 mmol) were dissolved in 5 mL anhydrous DMF under nitrogen. The mixture was allowed to stir at room temperature for 2 h. The solvent was removed under reduced pressure. Then the residue was purified on a silica gel column with CH2Cl2 to CH2Cl2/methanol (v/v, 200/1 to 15/1) as eluent to afford NIR-pH-H2O2 as black solid (30 mg, 21% yield). 1H NMR (500 MHz, DMSO-d6) δ 8.55 (s, 1H), 8.06 (s, 2H), 8.04 (s, 1H), 7.90 (d, J = 9.5 Hz, 1H), 7.79 (d, J = 7.7 Hz, 2H), 7.67 (d, J = 8.6 Hz, 2H), 7.48–7.46 (m, 1H), 7.38–7.36 (m, 4H), 7.30 (d, J = 1.7 Hz, 1H), 5.25 (s, 2H), 3.68 (m, 4H), 2.90–2.83 (m, 4H), 1.81 (m, 2H), 1.21 (t, J = 6.8 Hz, 6H). 13C NMR (125 MHz, DMSO-d6) δ 161.81, 159.16, 156.67, 153.13, 151.66, 149.34, 137.02, 134.82, 134.61, 133.65, 132.67, 132.45, 129.25, 127.66, 123.94, 122.15, 120.02, 119.37, 95.98, 70.51, 70.27, 55.40, 46.21, 40.52, 40.36, 40.19, 40.02, 39.86, 39.69, 39.52, 31.76, 30.88, 29.49, 27.19, 26.99, 22.57, 21.59, 14.42, 12.89. HRMS (ESI): calcd for C32H33BNO6+ [M]+ m/z 538.2395, found 538.2416. Purity: 98.5% (HPLC).
Results and discussion
UV-vis and fluorescence responses to pH
Scheme 2 shows the synthetic route adopted for the preparation of NIR-pH-H2O2, whose structure was further characterized by 1H NMR, 13C NMR and HRMS (Fig. S8–S10†). The fluorescence behaviors of NIR-pH-H2O2 (10 μM) towards pH were obtained containing 6% CH3CN (v/v). As shown in Fig. 1, a significantly large stokes shift of 120 nm was noticed of NIR-pH-H2O2 with an absorption maximum (λabs) of 560 nm (Fig. 1A) and an emission maximum (λem) of 680 nm (Fig. 1B). Both intensity of absorption at 560 nm and fluorescence at 680 nm were dramatically reduced as the value of pH (2.96–9.08) gradually increased (Fig. 1C). Moreover, there is an excellent linear relationship of pH over the range of 4.93 to 7.47 (Fig. 1C inset, R2 = 0.9937). By the nonlinear regression analysis, the pKa value was calculated to be 6.17, indicating that NIR-pH-H2O2 is capable of assessing acidic media. Additionally, the probe shows satisfactory reversibility between pH 4.0 and pH 8.0 (Fig. 1D).
 |
| Scheme 2 Synthesis route for NIR-pH-H2O2. | |
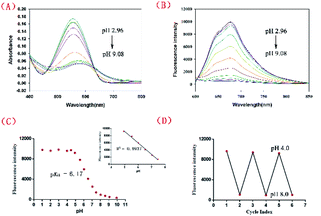 |
| Fig. 1 (A) Absorption and (B) fluorescence emission spectra of NIR-pH-H2O2 (10 μM) in the phosphate buffer (6% CH3CN) with various pH values. (C) Plot of I 680 vs. pH in the range of 1.02 to 9.94. Inset: the linear fitting curve of I680nm vs. pH from 4.93 to 7.47. (D) Reversible fluorescence changes of NIR-pH-H2O2 between pH 4.0 and 8.0 with λex at 560 nm. | |
UV-vis and fluorescence responses to H2O2
To investigate the interaction between H2O2 and NIR-pH-H2O2, UV titration was performed (Fig. 2A). The main absorption peak at 560 nm obviously increased with H2O2 (200 μM). Subsequently, the fluorescence of NIR-pH-H2O2 were explored (Fig. 2B). The free probe exhibited a weak fluorescence emission at 680 nm in PBS buffer. However, its fluorescence immediately increased in the presence of H2O2 (200 μM). This result was consistent with its UV absorption spectrum to H2O2. As expected, NIR-pH-H2O2 is weak-fluorescent (Fig. 2B) in NIR area. The introduction of H2O2 could specifically convert the phenylboronic acid unit into an electron-donor-OH group with CO2 released through a 1,6-benzyl elimination, which resulted a significant fluorescence enhancement at 680 nm. A fluorescence titration of NIR-pH-H2O2 was carried out to further investigate the quantitative relationship between NIR-pH-H2O2 and H2O2. With the increasing of H2O2, its fluorescence intensity at 680 nm gradually increased (Fig. 2C and D). When the concentration increased to 130 μM, the fluorescence almost kept stable. In addition, the fluorescence intensity of NIR-pH-H2O2 showed an excellent linear relationship with the H2O2 concentration (R2 = 0.9968 Fig. 2D inset). The detection limit (utilizing the 3σ/k method) for H2O2 was determined 2.097 μM. which was enough for direct image of intracellular H2O2 as its concentrations are usually in sub-micromolar range.25 To ensure whether NIR-pH-H2O2 could be applied for real-time detection, the kinetic experiments towards H2O2 were explored (Fig. S1†). It was displayed that the detection process balanced within 30 minutes, after adding H2O2 to the probe solution and a small quantity of enhancement was observed within the next 20 minutes.
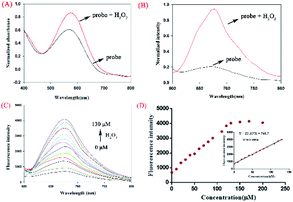 |
| Fig. 2 Absorption (A) and fluorescence (B) spectra of NIR-pH-H2O2 (10 μM) in PBS buffer (6% CH3CN). (C) Fluorescence spectra of NIR-pH-H2O2 (10 μM) in the presence of various amounts of H2O2 (0–130 μM) in PBS buffer. (D) The curve was plotted with fluorescence intensity vs. H2O2 concentration (0–200 μM) inset: linear relationship between fluorescence intensity of NIR-pH-H2O2 and H2O2 concentration (0–130 μM) at 680 nm (λex = 560 nm). | |
Sensitivity detection
Subsequently, the fluorescence specificity of NIR-pH-H2O2 was examined over various other species. As shown in Fig. 3, the fluorescence intensity at 680 nm of H+ and H2O2 are stronger than the other tested analytes in the phosphate buffer (6% CH3CN) indicating that NIR-pH-H2O2 was sensitive to protonic acid (pH = 4) and H2O2. These unique properties enable it to be feasible to accurately measure intracellular pH and H2O2 with high selectivity.
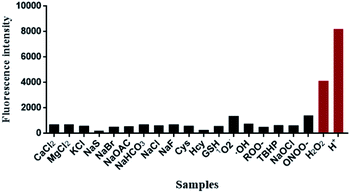 |
| Fig. 3 Fluorescence response of NIR-pH-H2O2 (10 μM) upon addition of various analytes (200 μM: CaCl2; MgCl2; KCl; NaS; NaBr; NaOAC; NaHCO3; NaCl; NaF; Cys; Hcy; GSH; 1O2; ·OH; ROO·; TBHP; NaOCl; ONOO–; H2O2 and H+ (pH = 4) in PBS buffer (6% CH3CN)). | |
The response between NIR-pH-H2O2 and H2O2 at different pH
It was of great significance to know the response between NIR-pH-H2O2 and H2O2 at different pH levels. The fluorescence intensity of NIR-pH-H2O2 and NIR-pH-H2O2 + H2O2 was then measured in PBS buffer from pH 1 to 10, respectively (Fig. S2†). It was found that fluorescence intensity of NIR-pH-H2O2 can increase upon the addition of H2O2 in the pH (1–10) range and the most obvious increase toward H2O2 could be observed from pH 6.0 to 8.0, indicating it can be applied in most living organisms (the physiological environment pH = 7.4).
Mechanism studies
The phenylboronic acid group in NIR-pH-H2O2 was the reaction site with H2O2 (Scheme 1). The starting material TP-NIR-OH was produced through a 1,6-benzyl elimination. The result of the electrospray ionization mass spectrometry (ESI-MS) confirmed the mechanism of this reaction. Addition of H2O2 in the NIR-pH-H2O2 solution resulted in a new peak at 360.19578 which correspond to TP-NIR-OH [M + H+] (Fig. S12†). The same results were also shown in the HPLC analysis (Fig. S13†). Thus, the results were in line with the proposed mechanism.
Cytotoxicity and cell mitochondria-targeting specificity of NIR-pH-H2O2
Before intracellular experiments, a standard MTT assay of MCF-7 and Hela cell lines were carried out to evaluate the biocompatibility of NIR-pH-H2O2. When the probe concentration reached 50 μM, NIR-pH-H2O2 displayed negligible cell toxicity since nearly 80% of MCF-7 cells (Fig. S3A†) and 85% of HeLa cells (Fig. S3B†) survived and could be applied to biological cells. To confirm the presumed mitochondria-targeting specificity of NIR-pH-H2O2, the colocalization experiments were performed in MCF-7 cells using Mito Tracker Green (a commercial mitochondria-targeting dye). As expected, the fluorescence image produced using NIR-pH-H2O2 (Fig. 4A) overlaps with that obtained using Mito Tracker Green (Fig. 4B), the fluorescence distribution of NIR-pH-H2O2 overlaps well with that of Mito Green (Pearson’ s coefficient, 0.90; Fig. S4, parts D and E†). In summary, these data proved that NIR-pH-H2O2 was a good mitochondria-targeted fluorescent probe.
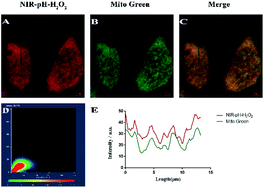 |
| Fig. 4 Colocalization experiments involving NIR-pH-H2O2 and Mito Tracker Green (MTG) in MCF-7 cells. The cells were incubated with NIR-pH-H2O2 (10 μM) and MTG (5 μM) for 30 min at 37 °C, followed by treatment with 200 μM H2O2 for 30 min at 37 °C. Images for NIR-pH-H2O2 (A) and MTG (B) were then recorded using excitation wavelengths of 555 nm and 485 nm, and band-path emission filters at 680 nm and 514 nm, respectively. Panels (C) shows a merged image of (A) and (B). (D) Intensity scatter plot of channels 1 and 2. (E) Intensity correlation plot of NIR-pH-H2O2 and MTG (Pearson's coefficient 0.90). Scale bar: 2 μm. | |
Cell imaging of NIR-pH-H2O2 for pH and H2O2
To explore capacity of NIR-pH-H2O2 for living cell fluorescence imaging of pH changes, MCF-7 cells and Hela cells were evaluated using the confocal fluorescence imaging. The fluorescence intensity of NIR-pH-H2O2 in MCF-7 cells (Fig. 5A) and Hela cells (Fig. S4A†) gradually decreased with increasing pH over the range of pH 5.08 to 7.84, indicated that NIR-pH-H2O2 could be applied for the detection of pH in living cells. We then employed NIR-pH-H2O2 to determine the pH change in mitochondria with a rapamycin model.32 Rapamycin, a widely used macrolide antibiotic, induces mitophagy in a variety of cell types and acidification of mitochondria has been observed during the mitophagy.33–35 MCF-7 cells and HeLa cells were treated with NIR-pH-H2O2 and rapamycin for 1 h then fluorescence images were recorded. As expected, the merged images of the rapamycin-treated MCF-7 cells (Fig. 5B) and HeLa cells (Fig. S4B†) exhibit more obvious colour change, which is ascribed to the rapamycin-induced generation of mitophagy and decrease of the mitochondrial pH.
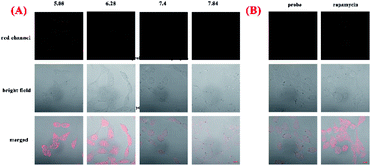 |
| Fig. 5 (A) Fluorescent microscopic images of MCF-7 cells with NIR-pH-H2O2. Incubated cells with 10 μL of NIR-pH-H2O2 (10 μM) under the pH 5.08, 6.28, 7.4, 7.84 and (B) rapamycin (10 μM) of the culture medium for 30 min and observed under the microscope (λex = 555 nm, λem = 680 nm for the red channel). Scale bar: 10 μm. | |
Next, we investigated the fluorescence imaging of NIR-pH-H2O2 for H2O2 in MCF-7 (Fig. 6a–d) cells and Hela cells (Fig. S5a–d†). After incubation with probe NIR-pH-H2O2 for 30 min at 37 °C, almost no fluorescence could be observed for non-H2O2. However, fluorescence intensities became increased after treated with increased exogenous H2O2. The results were consistent with our previous results of fluorescence spectra, showing that NIR-pH-H2O2 could be used for H2O2 detection in living cells. Next, application of NIR-pH-H2O2 to visualize endogenous H2O2 in MCF-7 cells and Hela cells by confocal fluorescence microscopy. To induce acute endogenous H2O2 production, the nonspecific protein kinase C activator phorbol myristate acetate (PMA; 200 ng mL−1) was co-incubated with NIR-pH-H2O2 for 30 min, followed by confocal imaging.36,37 Fluorescence intensity of NIR-pH-H2O2 sharply enhanced due to PMA treatment triggered an oxidative burst in for MCF-7 cells (Fig. 6e) and Hela cells (Fig. S5e†) compared with the control group. These results indicate the capability of NIR-pH-H2O2 in determining the endogenous changes of H2O2 in living cells. Dynamic fluorescence imaging for NIR-pH-H2O2 was further conducted. After incubated with exogenous H2O2 and probe NIR-pH-H2O2 in MCF-7 (Fig. S6†) and Hela cell lines (Fig. S7†), red fluorescence was enhanced gradually after incubated for 0 min, 10 min, 20 min and 30 min. The results were consistent with our previous results of fluorescence spectra.
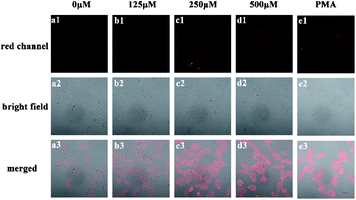 |
| Fig. 6 Fluorescence images of NIR-pH-H2O2 (10 μM) with various concentrations of H2O2 solution and PMA (λex = 555 nm, λem = 680 nm for the red channel). The MCF-7 was treated with NIR-pH-H2O2 and different concentration of H2O2 and PMA for 30 min. Scale bar: 10 μm. | |
Fluorescence imaging of NIR-pH-H2O2 in mice
Having achieved successful cell imaging with NIR-pH-H2O2, a further exploratory effort was made to determine whether it would be competent of fluorescence imaging in vivo and reflect occurrence of biological processes induced by exogenous stimulation. Since abdominal inflammation induced by lipopolysaccharide (LPS) could cause changes of pH and overproduction of hydrogen peroxide,38–40 we investigated the application of NIR-pH-H2O2 to visualize fluorescence changes in an abdominal inflammation mouse model induced by LPS. As shown in Fig. 7b, the mouse treated with saline and NIR-pH-H2O2 exhibited low fluorescence intensity. However, the fluorescence intensity in the mouse treated with LPS and NIR-pH-H2O2 changed evidently (Fig. 7c), which was attributed to that the inflammatory response could reduce the local pH and increased the level of H2O2. As the control, the mouse treated with LPS but no NIR-pH-H2O2 injection showed no fluorescence (Fig. 7a). Therefore, these results demonstrate that NIR-pH-H2O2 is a prominent fluorescent probe for imaging inflammation in vivo.
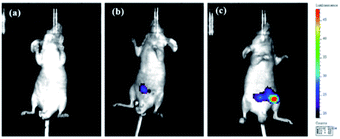 |
| Fig. 7 In vivo fluorescence images in the peritoneal cavity of mice injected with NIR-pH-H2O2 during LPS-induced inflammatory response. (a) Only LPS was injected for control. (b) Saline was injected in the peritoneal cavity of mouse, followed by injection of NIR-pH-H2O2 (50 μM). (c) LPS was in-jected into the peritoneal cavity of the mouse, followed by injection with NIR-pH-H2O2 (50 μM). The mice were imaged with an excitation filter at 560 nm and emission filter at 680 nm. | |
Conclusions
In summary, we firstly reported a novel “off–on” dual functional NIR fluorescent probe NIR-pH-H2O2 to monitor pH and H2O2 in vitro and in vivo. The cellular assay confirmed that NIR-pH-H2O2 is co-localized in mitochondria with low cytotoxicity. Furthermore, the probe shows excellent pH sensitivity, good response to H2O2, and successful imaging of pH and H2O2 in mice inflammation model induced by LPS. Mitochondria acidification is seen during the mitophagy. Abnormal levels of mitophagy and H2O2 are associated with various pathological conditions such as cancer, inflammation and neurodegenerative diseases. On the basis of these results, we envision that NIR-pH-H2O2 could be employed to understand the roles of reduced pH and increased H2O2 in pathological conditions and further for the pH and H2O2 related diseases diagnosis and real-time monitoring.
Conflicts of interest
There are no conflicts to declare.
Acknowledgements
This work was supported by the National Natural Science Foundation of China (81973187), “Six Talent Peaks” Project of Jiangsu Province (2016-YY-042), National Key Research and Development Program (2017YFC0107700), “Double First-Class” University project (CPU2018GY24, CPU2018GY38) and National Found for Fostering Talents of Basic Science (NFFTBS, No. J1310032).
Notes and references
- W. J. Waddell and R. G. Bates, Physiol. Rev., 1969, 49, 285–329 CrossRef CAS PubMed.
- R. A. Gottlieb and A. Dosanjh, Proc. Natl. Acad. Sci. U. S. A., 1996, 93, 3587–3591 CrossRef CAS PubMed.
- D. Pérez-Sala, D. Collado-Escobar and F. Mollinedo, J. Biol. Chem., 1995, 270, 6235–6242 CrossRef PubMed.
- P. Donoso, M. Beltrán and C. Hidalgo, Biochemistry, 1996, 35, 13419–13425 CrossRef CAS PubMed.
- K. Ji, L. Mayernik, K. Moin and B. F. Sloane, Cancer Metastasis Rev., 2019, 38, 103–112 CrossRef PubMed.
- J. M. Holopainen, J. Saarikoski, P. K. Kinnunen and I. Järvelä, Eur. J. Biochem., 2001, 268, 5851–5856 CrossRef CAS PubMed.
- T. Finkel, M. Serrano and M. A. Blasco, Nature, 2007, 448, 767–774 CrossRef CAS PubMed.
- C. H. Byon, J. M. Heath and Y. Chen, Redox Biol., 2016, 9, 244–253 CrossRef CAS PubMed.
- E. Niedzielska, I. Smaga, M. Gawlik, A. Moniczewski, P. Stankowicz, J. Pera and M. Filip, Mol. Neurobiol., 2016, 53, 4094–4125 CrossRef CAS PubMed.
- M. P. Murphy, A. Holmgren, N.-G. Larsson, B. Halliwell, C. J. Chang, B. Kalyanaraman, S. G. Rhee, P. J. Thornalley, L. Partridge, D. Gems, T. Nyström, V. Belousov, P. T. Schumacker and C. C. Winterbourn, Cell Metab., 2011, 13, 361–366 CrossRef CAS PubMed.
- L. Park, P. Zhou, R. Pitstick, C. Capone, J. Anrather, E. H. Norris, L. Younkin, S. Younkin, G. Carlson, B. S. McEwen and C. Iadecola, Proc. Natl. Acad. Sci. U. S. A., 2008, 105, 1347–1352 CrossRef CAS PubMed.
- N. Houstis, E. D. Rosen and E. S. Lander, Nature, 2006, 440, 944–948 CrossRef CAS PubMed.
- C. Behl, Cell, 1994, 77, 817–827 CrossRef CAS PubMed.
- Y. Wang, H. Wang, Y. Chen, X. Liu, Q. Jin and J. Ji, Colloids Surf., B, 2014, 121, 189–195 CrossRef CAS PubMed.
- M. S. Moon, E. W. Cho, H. S. Byun, I. L. Jung and I. G. Kim, Arch. Biochem. Biophys., 2004, 430, 229–236 CrossRef CAS PubMed.
- M. Duz, A. G. Whittaker, S. Love, T. D. H. Parkin and K. J. Hughes, Res. Vet. Sci., 2009, 87, 307–312 CrossRef CAS PubMed.
- K. Murata, K. Fujimoto, Y. Kitaguchi, T. Horiuchi, K. Kubo and T. Honda, Chronic Obstr. Pulm. Dis., 2014, 11, 81–87 CrossRef PubMed.
- L. Ernster and G. Schatz, J. Cell Biol., 1981, 91, 227s–255s CrossRef CAS PubMed.
- L. Jiang, L. Li, X. He, Q. Yi, B. He, J. Cao, W. Pan and Z. Gu, Biomaterials, 2015, 52, 126–139 CrossRef CAS PubMed.
- G. E. Weitsman, R. Koren, E. Zuck, C. Rotem, U. A. Liberman and A. Ravid, Free Radical Biol. Med., 2005, 39, 266–278 CrossRef CAS PubMed.
- I. A. Pomytkin, Curr. Neuropharmacol., 2012, 10, 311–320 CrossRef CAS PubMed.
- J. Marx, Science, 2005, 307, 334–335 CrossRef CAS PubMed.
- L. Zhou, D. Lu, Q. Wang, S. Liu, Q. Lin and H. Sun, Biosens. Bioelectron., 2017, 91, 699–705 CrossRef CAS PubMed.
- K. Liu, H. Shang, X. Kong, M. Ren, J.-Y. Wang, Y. Liu and W. Lin, Biomaterials, 2016, 100, 162–171 CrossRef CAS PubMed.
- G. C. van de Bittner, E. A. Dubikovskaya, C. R. Bertozzi and C. J. Chang, Proc. Natl. Acad. Sci. U. S. A., 2010, 107, 21316–21321 CrossRef CAS PubMed.
- X.-Q. Zhan, B.-Y. Su, H. Zheng and J.-H. Yan, Anal. Chim. Acta, 2010, 658, 175–179 CrossRef CAS PubMed.
- L. Zhou, H. Ding, W. Zhao and S. Hu, Spectrochim. Acta, Part A, 2019, 206, 529–534 CrossRef CAS PubMed.
- Z. Guo, I. Shin and J. Yoon, Chem. Commun., 2012, 48, 5956–5967 RSC.
- H. Y. Lee, X. Jiang and D. Lee, Org. Lett., 2009, 11, 2065–2068 CrossRef CAS PubMed.
- P. Wang, K. Wang and Y. Gu, Sens. Actuators, B, 2016, 228, 174–179 CrossRef CAS.
- B. Dong, X. Song, C. Wang, X. Kong, Y. Tang and W. Lin, Anal. Chem., 2016, 88, 4085–4091 CrossRef CAS PubMed.
- X. Li, Y. Hu, X. Li and H. Ma, Anal. Chem., 2019, 91, 11409–11416 CrossRef CAS PubMed.
- Y.-Q. Shen, A. Guerra-Librero, B. I. Fernandez-Gil, J. Florido, S. García-López, L. Martinez-Ruiz, M. Mendivil-Perez, V. Soto-Mercado, D. Acuña-Castroviejo, H. Ortega-Arellano, V. Carriel, M. E. Diaz-Casado, R. J. Reiter, I. Rusanova, A. Nieto, L. C. López and G. Escames, J. Pineal Res., 2018, 64, 1–18 CrossRef PubMed.
- F. Hu, X. Cai, P. N. Manghnani, Kenry, W. Wu and B. Liu, Chem. Sci., 2018, 9, 2756–2761 RSC.
- H. Yuan, H. Cho, H. H. Chen, M. Panagia, D. E. Sosnovik and L. Josephson, Chem. Commun., 2013, 49, 10361–10363 RSC.
- S. Ye, J. J. Hu and D. Yang, Angew. Chem., Int. Ed. Engl., 2018, 57, 10173–10177 CrossRef CAS PubMed.
- P. Wu, Z. Cai, Y. Gao, H. Zhang and C. Cai, Chem. Commun., 2011, 47, 11327–11329 RSC.
- Y. Li, Y. Wang, S. Yang, Y. Zhao, L. Yuan, J. Zheng and R. Yang, Anal. Chem., 2015, 87, 2495–2503 CrossRef CAS PubMed.
- N. Karton-Lifshin, E. Segal, L. Omer, M. Portnoy, R. Satchi-Fainaro and D. Shabat, J. Am. Chem. Soc., 2011, 133, 10960–10965 CrossRef CAS PubMed.
- L. Fan, X. Wang, J. Ge, F. Li, C. Zhang, B. Lin, S. Shuang and C. Dong, Chem. Commun., 2019, 55, 6685–6688 RSC.
Footnote |
† Electronic supplementary information (ESI) available. See DOI: 10.1039/d0ra03905e |
|
This journal is © The Royal Society of Chemistry 2020 |