DOI:
10.1039/D0RA03726E
(Paper)
RSC Adv., 2020,
10, 20264-20271
Site-selective, catalytic, and diastereoselective sp3 C–H hydroxylation and alkoxylation of vicinally functionalized lactams†
Received
26th March 2020
, Accepted 19th May 2020
First published on 27th May 2020
Abstract
The C–H bond functionalization of sp3 carbon centres presents a significant challenge due to the inert nature of hydrocarbons as well as the need to selectively functionalize one of the numerous aliphatic C–H bonds embodied in organic molecules. Here, we describe catalytic, diastereoselective, and site-selective sp3 C–H hydroxylation/alkoxylation protocols featuring dihydroisoquinolones, γ-, and δ-lactams, which bear vicinal stereocenters. The hydroxylation strategy utilizes oxygen, a waste-free oxidant and affords attractive fragments for potential drug discovery. Fe-catalyzed dehydrative coupling of the resulting tertiary alcohols with simple primary alcohols has led to the construction of highly versatile unsymmetrical dialkyl ethers.
Introduction
Site-selective functionalization of C–H bonds continues to provide chemists with transformative tools for editing and constructing complex molecular architectures. Indeed, nature is endowed with several enzymes that achieve direct and selective oxygenation of organic molecules.1 Benzylic C–H bonds are often the target of most of these enzymes in part due to their relatively low bond dissociation energy (BDE ∼90 kcal mol−1).2 Fittingly, functionalized benzylic cyclic amino tertiary alcohols constitute the core of several alkaloid natural products and pharmaceuticals, including anti-inflammatory agent Homocrepidine B,3 anti-HIV agent Nifeviroc,4 antipsychotic agent Haloperidol,5 and anticancer agent Donaxaridine6 (Fig. 1). Meanwhile, alcohol-bearing dihydroisoquinolones are resident in several natural products, including 6-oxocorynoline.7 We have therefore identified the development of a catalytic and stereoselective methodology for the site-selective hydroxylation and alkoxylation of tertiary C–H bonds in readily affordable dihydroisoquinolones (DHIQs) of type 1 and vicinally functionalized lactams such as 4 as an important research objective (Fig. 2A). Central among our objectives was the prospect of developing a protocol that enables the sp3 C–H hydroxylation of DHIQs while obviating the need for expensive metal catalysts, cryogenic conditions, and the reliance on directing groups. Such an efficient and selective sp3 C–H oxidation method would undeniably streamline the synthesis of drug metabolites, natural products, and fine chemicals.8 Despite rapid advances in the development of metal-catalyzed reactions,9 synthetically useful C(sp3)–H oxygenation chemistry is still in great demand.10 To the best of our knowledge, only a single report exists on the construction of reduced DHIQs (i.e., tetrahydroisoquinolines) bearing a tertiary alcohol motif at the C4 position of the azaheterocycle.11
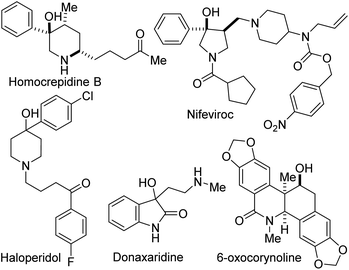 |
| Fig. 1 Examples of biologically active benzylic cyclic amino tertiary alcohols and hydroxylated dihydroisoquinolones. | |
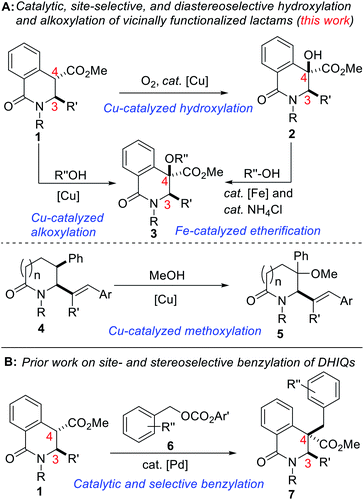 |
| Fig. 2 (A) Proposed plan for the construction of C4 hydroxylated dihydroisoquinolones and subsequent alkoxylation, (B) selective and catalytic benzylation of DHIQs. | |
Remarkable progress has been made using transition-metal catalysis with molecular O2 for selective sp3 C–H hydroxylation.12 The use of O2 for the synthesis of quaternary α-hydroxyl carbonyl compounds was first described by Ritter and co-workers using dinuclear palladium complexes.13 Herein, we report a site-selective, Cu-catalyzed, and diastereoselective approach to hydroxylated DHIQs with excellent functional group compatibility, which uses oxygen as the sole oxidant (see 2). Fe-catalyzed dehydrative coupling of these sterically congested alcohols has led to the synthesis of unsymmetrical alkyl ethers (see 3). Direct Cu-catalyzed sp3 C–H methoxylation of vicinally functionalized γ- and δ-lactams has also been accomplished (see 5). These selective C–O bond-forming reactions nicely complement our recently disclosed C–C bond-forming tactic (Fig. 2B).14
Results and discussion
The substitution of noble metals by earth-abundant, inexpensive and relatively less toxic metals is one of the most beneficial aspects of modern day transition metal-catalyzed processes. Accordingly, copper-catalyzed oxidative C–H functionalization protocols have emerged as alternatives to well-heeled but less practical Pd-catalyzed processes.15 Seeking to take advantage of the aforementioned merits of Cu-catalysis and inspired by the ubiquity of cyclic amine-bearing tertiary alcohols in bioactive compounds, the amenability of DHIQ 1 to diastereoselective and site-selective hydroxylation, with O2 as the oxidant, was explored. After surveying several conditions (Table 1), we were pleased to find that model DHIQ 1a undergoes efficient hydroxylation to furnish product 2a in 92% yield. Magnesium-mediated (entry 9), potassium-mediated (entry 10), and organocatalytic conditions (entry 11) were also evaluated, but they did not perform as well as these Schoenebeck-inspired,16 Cu-catalyzed conditions. Control experiments in the absence of base or oxygen revealed that both components are necessary.
Table 1 Optimization of the diastereoselective and site-selective hydroxylation of DHIQ 1a
With efficient conditions for site-selective and catalytic hydroxylation of DHIQs in hand, the scope of the transformation with respect to the stereoelectronics of the DHIQ was next explored (Scheme 1). DHIQs bearing N-benzyl, alkyl, and allyl substituents were surveyed. Pleasingly, the hydroxylated DHIQs are obtainable in synthetically attractive yields when a wide range of diversely functionalized lactam esters are exposed to the identified reaction conditions (see 2a–s). The transformation fully tolerates C3 alkenyl substituents (entries 2a–h), which bodes well for late-stage diversification given that the alkenyl motif could pave the way for harnessing several reactivity modes, including hydroarylation,17 oxoamination,18 trifluoromethylation,19 oxacyclopropanation,20 and aziridination.21 Owing to the continuing emergence of C3-arylated dihydroisoquinolones and tetrahydroquinolines with medicinal potential, DHIQs bearing benzylic α-amino stereocenters were surveyed. In the event, we find that this site-selective oxygenation protocol proceeds with great tolerance for electron-neutral, electron-rich, and electron-deficient arenes. Of note, site-selective hydroxylation still occurs even when potentially problematic benzyloxy and allyloxy groups are employed (see 2k–n). Notably, ortho-substituted arenes are amenable to this hydroxylation reaction, which converts sp3 α-C–H bonds of hindered esters to C–O bonds (see 2n–p).
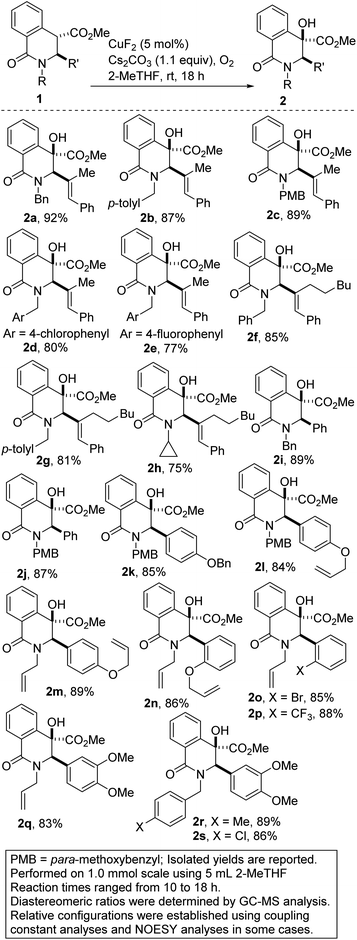 |
| Scheme 1 C4 hydroxylation of dihydroisoquinolones. | |
A hydroxylated DHIQ harboring a requisite halogen group for potential cross-coupling is obtainable in synthetically useful yield (see 2o). The incorporation of a fluorinated moiety into organic molecules generally increases the solubility, lipophilicity and metabolic stability of the parent molecules, thus, explaining why ∼25% of existing preclinical drugs and 40% of agrochemicals contain at least one fluorine atom.22 Specifically, the CF3 group enjoys a privileged role because its incorporation often enhances efficacy by promoting electrostatic interactions with targets, improving cellular membrane permeability, and increasing robustness toward oxidative metabolism of the drug.23 It is therefore noteworthy that DHIQs 2e (fluorine-containing) and 2p (CF3-containing), are obtainable in good yields.
Gleaning from prior detailed mechanistic studies on Cu-catalyzed hydroxylation or scission of enolizable and branched ketones,16 we propose that 2 arises from 1 via a sequence involving enolization and oxygenation of the ester enolate to peroxide 8, which either undergoes reduction or homolytic O–O cleavage (see 9) and concomitant hydrogen atom abstraction (Fig. 3). The diastereoselectivity of the hydroxylation is presumably governed by substituent effects, especially the vicinal C3 substituent.
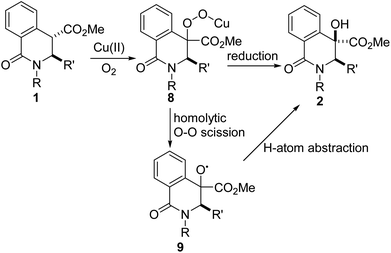 |
| Fig. 3 Possible mechanism for the formation of 2. | |
Post-diversification strategies that enable the step-economical incorporation of new latent functionality often provide an efficient strategy for rapidly accessing structural analogues of pharmaceutical candidates with a diverse array of chemical and biological properties. Fittingly, reduction of the ester and lactam groups resident in 2a/p affords tetrahydroisoquinolines (THIQs) of type 10 (Scheme 2). The reliable synthesis of THIQs bearing a hydroxymethyl group such as 10, is noteworthy given that this substructure constitutes the core of a few alkaloids, including calycotomine.24
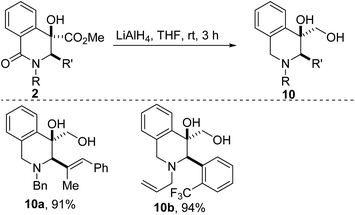 |
| Scheme 2 Synthesis of C4 hydroxylated tetrahydroisoquinolines. | |
Iron-catalyzed dehydrative etherification of 2
The development of effective coupling methods that rely on the cleavage of unactivated C(sp3)–O bonds is synthetically and theoretically appealing. However, formidable challenges remain to be addressed given that carbon–oxygen bonds are strong (∼95 kcal mol−1).25 Thus, forcing conditions are typically required to achieve useful conversions and even these are subject to limitations. Direct intermolecular dehydrative coupling of two alcohols to form unsymmetrical ethers is attractive from the standpoint of atom economy.26 This is all the more relevant since unsymmetrical alkyl ethers have widespread applications as solvents, plasticizers, disinfectants, herbicides, drug intermediates, fragrances, and as precursors for polymers.27 Moreover, in the realm of medicinal chemistry, hindered and unsymmetrical ethers are highly desirable given that extensive substitution about the ether bond prevents unwanted metabolic processes that can lead to rapid degradation in vivo. However, due to the poor departing ability of the hydroxyl functionality, hydroxide activation is challenging, particularly for nucleophilic substitution. These challenges notwithstanding, the construction of unsymmetrical ethers directly from two different alcohols, under transition-metal catalysis continues to garner attention from the synthesis community.28 However, Fe-catalyzed dehydrative etherification of two different alcohols has scarcely been investigated,29 which is unfortunate since iron is an earth abundant, less toxic, and environmentally benign metal that is capable of emulating or surpassing the catalytic activities of noble transition metals.30
Inspired by our prior success on Fe-catalyzed dehydrative intramolecular coupling of alkenols,31 and seeking to further demonstrate the synthetic utility of the catalytic hydroxylation protocol described herein, we interrogated the hindered benzylic alcohols depicted in Scheme 1 in an intermolecular, Fe-catalyzed cross-coupling protocol with simple alcohols. Pleasingly, we have found that these lactam-bearing alcohols are amenable to efficient Fe(OTf)3-catalyzed dehydrative coupling (Table 2 and Scheme 3). No reaction is observed in the absence of the iron catalyst and minimal coupling is observed when Fe(OTf)3 is replaced by FeCl3·6H2O. An excess of methanol is necessary to compensate for the formation of small amounts of dimethylether. Indeed, the homocoupling product was suppressed only when ammonium chloride was introduced. Several hydroxylated DHIQs react satisfactorily with methanol (see 3a–k). Isotopically labelled methanol reacts prudently and affords deuterated ether 3l. Benzyl alcohol is also a suitable alcohol for this etherification process (see 3m), which is noteworthy since organic and medicinal chemists routinely employ benzyl ethers as protecting/directing groups as they tackle synthetic targets of increasing complexity. The use of ligand-free, simple, cheap, and readily available Fe(OTf)3 as a catalyst makes this protocol highly attractive and endows it with a practical advantage over existing methodologies. This carbocation-driven process should in principle tolerate hindered nonbenzylic alcohols as reactive partners. Accordingly, the amenability of hindered secondary as well as tertiary alcohols to this intermolecular etherification process is currently under investigation and the results will be disclosed later.
Table 2 Optimization of the catalytic dehydrative etherification of 2i
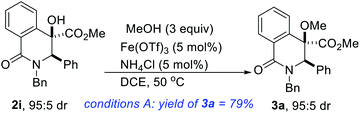
|
Entry |
Deviation from conditions A |
% yield as solvent |
1 |
PhMe as solvent |
0 |
2 |
CH2Cl2 as solvent |
67 |
3 |
2-MeTHF as solvent |
48 |
4 |
FeCl3·6H2O in place of Fe(OTf)3 |
39 |
5 |
Fe(NO3)3 (5 mol%) in place of Fe(OTf)3 |
0 |
6 |
No Fe(OTf)3 |
0 |
7 |
No NH4Cl |
0 |
8 |
Conditions B in place of conditions A |
57 |
9 |
Conditions C in place of conditions A |
Trace |
 |
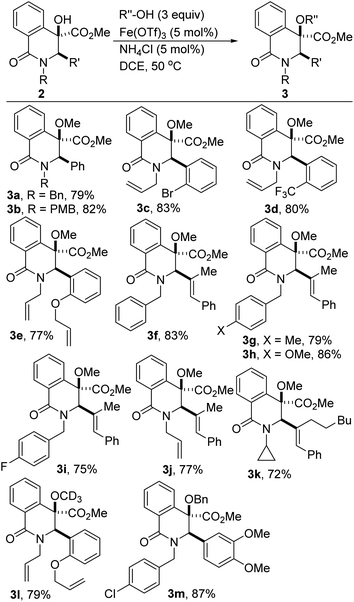 |
| Scheme 3 Fe-catalyzed intermolecular dehydrative coupling of tertiary dihydroisoquinolinols with simple alcohols. | |
Copper-catalyzed site-selective dehydrogenative etherification of vicinally functionalized lactams
The direct alkoxylation of sp3-hybridized C–H bonds (especially those that are not adjacent to a heteroatom) remains attractive, particularly in the context of late-stage modification of pharmaceutically-pertinent fragments.32 Specifically, the installation of a methoxy group is quite appealing given that in addition to introducing a potential hydrogen bond acceptor site, it has minimal impact on the overall lipophilicity (i.e., log
P).33 Accordingly, we sought a one-step protocol for the conversion of vicinally functionalized lactams such as 1 to unsymmetrical methyl ethers. We reasoned that the successful implementation of this mode of reactivity would obviate the need for pre-installation of the hydroxyl functionality resident in 2. Importantly, we desired to achieve direct sp3 α-C–H methoxylation of lactams of type 4 (Scheme 4) which resisted sp3 α-C–H hydroxylation under the conditions described in Scheme 1. We were however not oblivious to the challenges associated with realizing such an atom-economical transformation in sterically congested environments and with substrates that bear many active/labile sp3 C–H bonds.
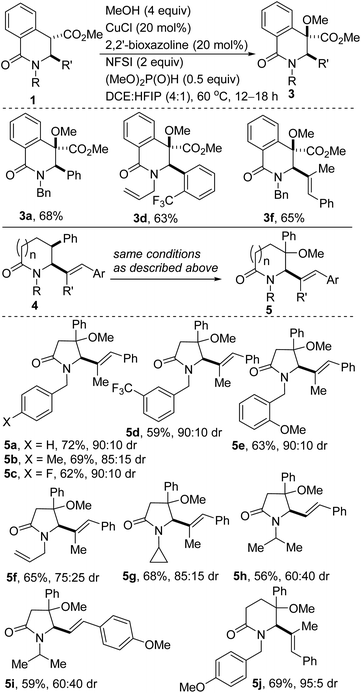 |
| Scheme 4 Site-selective methoxylation of vicinally functionalized lactams. | |
In keeping with our interest in Cu-catalyzed direct sp3 α-C–H oxygenation, these studies have revealed that DHIQs of type 1 undergo site-selective benzylic C–H methoxylation with methanol to afford benzyl methyl ethers (Scheme 4, see 3a/d/f). Importantly, these Stahl-inspired conditions34 allow for direct site-selective benzylic methoxylation of sp3 C–H bonds resident in vicinally functionalized γ-lactams (see 5a–i). Remarkably, site-selective benzylic methoxylation of the heterocycles occurs even when potentially active side-chain α-amino-benzyl (see 5a–e), allyl (see 5f), and tertiary (see 5g–i) C–H bonds are present. A vicinally functionalized δ-lactam is also amenable to this site-selective dehydrogenative methoxylation (see 5j). The relative configuration of 5 has not been fully established at this point but the diastereomeric ratios of most of the products are synthetically useful.
Conclusions
In summary, Cu-catalyzed and site-selective conversion of sp3 α-C–H bonds of hindered benzylic esters to C–O bonds in vicinally functionalized dihydroisoquinolones has been accomplished. Environmentally benign molecular oxygen is employed as the sole oxidant. The reaction shows compatibility with alkenes, which are typically sensitive to oxidative conditions and not tolerated in most aliphatic C–H oxidations. Substrates bearing multiple benzylic positions, including α-amino and α-alkoxy benzylic positions, undergo site-selective hydroxylation only at the benzylic position alpha to the ester. Post-diversification of the tertiary alcohols to unsymmetrical alkyl ethers by Fe-catalyzed dehydrative coupling with simple primary alcohols has also been achieved. Dehydrogenative methoxylation of vicinally functionalized lactams has led to the rapid construction of a small library of benzylic lactam ethers. The application of this strategies to simplify the synthesis of medicinally important entities and the extension of the Fe-catalyzed dehydrative coupling protocol to primary and tertiary alcohols are ongoing and the results will be disclosed in due course.
Experimental
All experiments involving air and moisture sensitive reagents were carried out under an inert atmosphere of nitrogen and using freshly distilled solvents. Column chromatography was performed on silica gel (230–400 mesh). Thin-layer chromatography (TLC) was performed using Silicycle Siliaplate™ glass backed plates (250 μm thickness, 60 Å porosity, F-254 indicator) and visualized using UV (254 nm) or KMnO4 stain. Unless otherwise indicated, 1H, 13C, and DEPT-135 NMR, and NOESY spectra were acquired using CDCl3 solvent at room temperature. Chemical shifts are quoted in parts per million (ppm). HRMS-EI+ data were obtained using either electronspray ionization (ESI) or electron impact (EI) techniques. High-resolution ESI was obtained on an LTQ-FT (ion trap; analyzed using Excalibur). High resolution EI was obtained on an Autospec (magnetic sector; analyzed using MassLynx). Representative GC-MS traces are provided to substantiate the diastereomeric ratios.
General procedure A: conversion of 1 to 2
To a solution of lactam ester 1 (1 mmol) and CuF2 (5 mol%) in anhydrous 2-methyltetrahydrofuran (5 mL) was added cesium carbonate (1.1 mmol, 1.1 equiv.). The reaction vessel was sealed, evacuated, refilled with oxygen gas, and stirred at room temperature for the desired time period (TLC and GC-MS monitoring) under oxygen atmosphere (which was maintained with an O2-filled balloon). The reaction mixture was diluted with an aqueous solution of hydrochloric acid (0.50 M, 10 mL) and extracted with ethyl acetate (3 × 20 mL). The combined organic phases were dried (MgSO4), filtered, and concentrated under reduced pressure. The crude product was purified by flash column chromatography eluting with hexanes/EtOAc to give the pure alcohol.
General procedure B: conversion of 2 to 8
To a 10 mL round-bottomed flask equipped with a magnetic stir bar under a N2 atmosphere, in a 0 °C ice/water bath, was added the lactam (0.25 mmol) and THF (10 mL). LiAlH4 (44 mg, 1.12 mmol) was then added portion-wise. The reaction mixture was allowed to warm to room temperature for 3 h (as judged complete by GC-MS analysis). After this time, the reaction mixture was cooled to 0 °C and quenched by slow addition of a solution of 2 N NaOH(aq.) (1 mL). The organic layer was decanted and the aqueous layer was extracted with EtOAc (3 × 5 mL). The combined organic layers were dried over anhydrous Na2SO4 and concentrated in vacuo to yield the crude tertiary aminodiol, which was purified by flash chromatography on silica.
General procedure C: conversion of 2 to 3
To the hydroxylated dihydroisoquinolone 2 (0.5 mmol), dissolved in 1,2-dichloroethane (2 mL), was added the primary alcohol (1.5 mmol, 3 equiv.), ammonium chloride (1.3 mg, 0.025 mmol), and Fe(OTf)3 (12.6 mg, 5 mol%) in a vial equipped with a magnetic stir bar, under open atmosphere. The reaction mixture was stirred at 50 °C. After completion of the reaction (TLC and GC-MS monitoring), the mixture was cooled to room temperature. The solvents were removed in vacuo and the crude mixture was dissolved in ethyl acetate and subjected to flash column chromatography on silica gel.
General procedure D: conversion of 4 to 5
To an oven-dried vial equipped with a stir bar was added copper(I) chloride (10.0 mg, 0.100 mmol, 20 mol%), 4,4′,5,5′-tetrahydro-2,2′-bioxazole (14 mg, 0.100 mmol, 10 mol%), and NFSI (315.2 mg, 1.0 mmol, 2.0 equiv.). The capped vial was placed under nitrogen followed by addition of 5 mL of a mixture of 1,2-dichloroethane and HFIP (4
:
1). Lactam 4 (0.50 mmol, 1.0 equiv.) dissolved in 1 mL DCE, methanol (84 μL, 2.0 mmol, 4.0 equiv.) and dimethyl phosphonate (24 μL, 0.25 mmol, 0.5 equiv.) were added to the septum-capped vial under nitrogen. The contents were then heated to 60 °C under stirring for the desired length of time. After completion of the reaction (TLC and GC-MS monitoring), the mixture was cooled to room temperature and triethylamine (0.5 mL) was added to quench any unreacted NFSI. The solvents were removed in vacuo and crude product 5 was dissolved in ethyl acetate and subjected to flash column chromatography on silica gel.
Synthesis of C4 hydroxylated DHIQ 2a
Prepared from ester 1a (411.5 mg, 1.0 mmol) using general procedure A. Purification: flash chromatography on silica eluting with hexane/EtOAc (80
:
20). Oily substance. Yield = 393 mg, 92%. 1H NMR (400 MHz, CDCl3) δ 8.26 (dd, J = 6.9, 2.1, 1H), 7.52–7.40 (m, 2H), 7.39–7.13 (m, 11H), 6.36 (s, 1H), 5.56 (d, J = 15.1 Hz, 1H), 4.17 (s, 1H), 4.08 (d, J = 15.1 Hz, 1H), 3.85 (br s, 1H), 3.64 (s, 3H), 1.47 (s, 3H). 13C NMR (101 MHz, CDCl3) δ 172.96, 163.16, 136.58, 136.41, 135.82, 134.07, 132.36, 132.07, 129.57, 129.22, 129.04, 129.01, 128.99, 128.77, 128.70, 128.67, 128.65, 128.59, 128.48, 128.43, 128.40, 128.37, 127.61, 127.38, 127.06, 76.97, 74.27, 53.32, 48.18, 13.60. HRMS calc. for C27H25NO4 427.1784, found 427.1788. FTIR (KBr): 3384.5506, 2924.8333, 1642.2515, 1494.9545, 1448.8548, 1427.0419, 1393.4602, 1361.6968, 1328.7144, 1289.7737, 1223.6425, 1198.9141, 1130.0001, 1074.1578, 1030.4745, 988.561, 966.1662, 925.5022, 741.6755, 693.4562.
Note: All other hydroxylated products depicted in Scheme 1 were prepared as described above. Spectroscopic data can be found in the ESI.†
Synthesis of alcohol 10a
Prepared from ester 2a (0.5 mmol) using general procedure B. Purification: flash chromatography on silica eluting with hexane/EtOAc (50
:
50). Oily substance. Yield = 175.4 mg, 91%. 1H NMR (400 MHz, CDCl3) δ 7.66 (dd, J = 7.5, 1.6 Hz, 1H), 7.49–7.29 (m, 12H), 7.05 (dd, J = 7.6, 1.5 Hz, 1H), 6.47 (s, 1H), 4.30 (d, J = 10.7 Hz, 1H), 4.06 (d, J = 13.3 Hz, 1H), 3.98 (d, J = 16.0 Hz, 1H), 3.80–3.65 (m, 3H), 3.62 (s, 1H), 3.56 (d, J = 13.3 Hz, 1H), 3.30 (s, 1H), 1.93 (s, 3H). 13C NMR (101 MHz, CDCl3) δ 138.05, 138.04, 137.11, 134.37, 133.12, 132.46, 129.30, 128.77, 128.34, 127.80, 127.63, 127.32, 127.05, 125.99, 125.22, 75.30, 72.14, 67.80, 60.19, 53.60, 18.48. HRMS calc. for C26H27NO2 385.2042, found 385.2047.
Note: All other alcohols depicted in Scheme 2 were prepared as described above. Spectroscopic data can be found in the ESI.†
Synthesis of ether 3a
Prepared in 0.5 mmol scale using general procedure C. Purification: flash chromatography on silica eluting with hexane/EtOAc (25
:
75). Oily substance. Yield = 158.6 mg, 79%. 1H NMR (400 MHz, CDCl3) δ 8.49 (d, J = 7.7 Hz, 1H), 7.65 (td, J = 7.6, 1.3 Hz, 1H), 7.57 (td, J = 7.6, 1.3 Hz, 1H), 7.45 (d, J = 7.3 Hz 1H), 7.32–7.27 (m, 8H), 6.85 (d, J = 7.2 Hz, 2H), 5.54 (d, J = 15.2 Hz, 1H), 4.85 (s, 1H), 3.82 (d, J = 15.2 Hz, 1H), 3.44 (s, 3H), 3.02 (s, 3H). 13C NMR (101 MHz, CDCl3) δ 169.57, 162.56, 136.33, 135.27, 131.18, 131.12, 130.18, 129.81, 129.79, 129.24, 128.93, 128.67, 128.54, 128.30, 127.98, 127.44, 83.35, 68.62, 52.89, 52.30, 48.76. HRMS calc. for C25H23NO4 401.1627, found 401.1633. FTIR (KBr): 2976.0754, 2927.2335, 1721.7979, 1650.1792, 1492.0415, 1438.4625, 1362.2698, 1320.5399, 1290.1484, 1206.364, 1180.3512, 1146.7618, 1132.397, 995.8166, 918.8793, 700.1334.
Note: All other ethers depicted in Scheme 3 were prepared as described above. Spectroscopic data can be found in the ESI.†
Synthesis of ether 5j
Prepared in 0.5 mmol scale using general procedure D. Purification: flash chromatography on silica eluting with hexane/EtOAc (75
:
25). Oily substance. Yield = 152.3 mg, 69%, 95
:
5 dr. 1H NMR (400 MHz, CDCl3) δ 7.30–7.17 (m, 10H), 6.90–6.85 (m, 4H), 6.12 (s, 1H), 5.22 (d, J = 17.1 Hz, 1H), 4.67 (s, 1H), 3.85–3.76 (m, 4H), 3.59 (s, 3H), 2.79–2.70 (m, 2H), 2.62–2.42 (m, 2H), 1.06 (s, 3H). 13C NMR (101 MHz, CDCl3) δ 169.08, 158.13, 136.41, 135.58, 134.11, 132.29, 131.94, 129.89, 129.58, 129.35, 129.02, 128.78, 128.47, 128.38, 127.35, 126.92, 114.10, 72.84, 67.90, 55.35, 53.34, 48.43, 29.59, 23.59, 17.32. HRMS calc. for C29H31NO3 441.2304, found 441.2309.
Note: All other methyl ethers depicted in Scheme 4 were prepared as described above. Spectroscopic data can be found in the ESI.†
Conflicts of interest
There are no conflicts of interest to declare.
Acknowledgements
We are grateful to Central Washington University for financial support to T. K. B. The School of Graduate Studies and Research is thanked for partial support of this work through a Faculty Research Award to T. K. B. V. S. thanks the McNair Scholars Program for a research fellowship.
Notes and references
- P. R. Ortiz de Montellano, Substrate Oxidation by Cytochrome P450 Enzymes, in Cytochrome P450: Structure, Mechanism, and Biochemistry, Springer International Publishing, Cham, 2015, pp. 111−176 Search PubMed.
- T. N. Thompson, Med. Res. Rev., 2001, 21, 412–449 CrossRef CAS.
-
(a) C. Decker, N. T. Viet, D. Decker and E. WeberKoehl, Polymer, 2001, 42, 5531 CrossRef CAS;
(b) Y. Hu, C. Zhang, Z. Zhao, Y. Wang, D. Feng, M. Zhang and H. Xie, J. Nat. Prod., 2016, 79, 252–256 CrossRef CAS PubMed.
- D. Ma, S. Yu, B. Li, L. Chen, R. Chen, K. Yu, L. Zhang, Z. Chen, D. Zhong, Z. Gong, R. Wang, H. Jiang and G. Pei, ChemMedChem, 2007, 2, 187–193 CrossRef CAS PubMed.
- M. W. Tyler, J. Zaldivar-Diez and S. J. Haggarty, ACS Chem. Neurosci., 2017, 8, 444–453 CrossRef CAS PubMed.
- H. B. Rasmussen and J. K. Macleod, J. Nat. Prod., 1997, 60, 1152–1154 CrossRef CAS.
- M. Cushman, A. Abbaspour and Y. P. Gupta, J. Am. Chem. Soc., 1983, 105, 2873 CrossRef CAS.
-
(a) T. Newhouse and P. S. Baran, Angew. Chem., Int. Ed., 2011, 50, 3362 CrossRef CAS PubMed;
(b) M. C. White, Science, 2012, 335, 807 CrossRef CAS PubMed;
(c) J. Genovino, D. Sames, L. G. Hamann and B. B. Toure, Angew. Chem., Int. Ed., 2016, 55, 14218 CrossRef CAS PubMed;
(d) P. A. Wender, M. K. Hilinski and A. V. W. Mayweg, Org. Lett., 2005, 7, 79 CrossRef CAS PubMed;
(e) K. Chen and P. S. Baran, Nature, 2009, 459, 824 CrossRef CAS PubMed;
(f) E. M. Stang and M. C. White, Nat. Chem., 2009, 1, 547 CrossRef CAS.
-
(a) D. Yang, M.-K. Wong, X.-C. Wang and Y.-C. Tang, J. Am. Chem. Soc., 1998, 120, 6611 CrossRef CAS;
(b) B. H. Brodsky and J. Du Bois, J. Am. Chem. Soc., 2005, 127, 15391 CrossRef CAS PubMed;
(c) K. Chen, J. M. Richter and P. S. Baran, J. Am. Chem. Soc., 2008, 130, 7247 CrossRef CAS PubMed;
(d) N. D. Litvinas, B. H. Brodsky and J. Du Bois, Angew. Chem., Int. Ed., 2009, 48, 4513 CrossRef CAS PubMed;
(e) S. Kasuya, S. Kamijo and M. Inoue, Org. Lett., 2009, 11, 3630 CrossRef CAS PubMed;
(f) A. M. Adams and J. Du Bois, Chem. Sci., 2014, 5, 656 RSC.
-
(a) X. Li, X. Che, G.-H. Chen, J. Zhang, J.-L. Yan, Y.-F. Zhang, L.-S. Zhang, C.-P. Hsu, Y. Q. Gao and Z.-J. Shi, Org. Lett., 2016, 18, 1234 CrossRef CAS PubMed;
(b) J. Ozawa, M. Tashiro, J. Ni, K. Oisaki and M. Kanai, Chem. Sci., 2016, 7, 1904 RSC;
(c) A. Hollister, E. S. Conner, M. L. Spell, . Deveaux, L. Maneval, M. W. Beal and J. R. Ragains, Angew. Chem., Int. Ed., 2015, 54, 7837 CrossRef PubMed.
- D. W. Low, G. Pattison, M. D. Wieczysty, G. H. Churchill and H. W. Lam, Org. Lett., 2012, 14, 2548–2551 CrossRef CAS PubMed.
-
(a) M. T. Rahman and H. Nishino, Org. Lett., 2003, 5, 2887 CrossRef CAS PubMed;
(b) H. Wang, Y. Wang, D. Liang, L. Liu, J. Zhang and Q. Zhu, Angew. Chem., Int. Ed., 2011, 50, 5678 CrossRef CAS PubMed;
(c) Z.-Q. Wang, W.-W. Zhang, L. B. Gong, R.-Y. Tang, X.-H. Yang, Y. Liu and J.-H. Li, Angew. Chem., Int. Ed., 2011, 50, 8968 CrossRef CAS PubMed;
(d) C. Zhang and N. Jiao, J. Am. Chem. Soc., 2010, 132, 28 CrossRef CAS PubMed;
(e) C. Zhang, Z. Xu, L. Zhang and N. Jiao, Angew. Chem., Int. Ed., 2011, 50, 11088 CrossRef CAS PubMed;
(f) L. Zhang, X. Bi, X. Guan, X. Li, Q. Liu, B.-D. Barry and P. Liao, Angew. Chem., Int. Ed., 2013, 52, 11303 CrossRef CAS PubMed;
(g) J. M. Hoover, B. L. Ryland and S. S. Stahl, J. Am. Chem. Soc., 2013, 135, 2357 CrossRef CAS PubMed;
(h) A. N. Campbell and S. S. Stahl, Acc. Chem. Res., 2012, 45, 851 CrossRef CAS PubMed;
(i) E. Boess, C. Schmitz and M. Klussmann, J. Am. Chem. Soc., 2012, 134, 5317 CrossRef CAS PubMed.
- G. J. Chuang, W. Wang, E. Lee and T. Ritter, J. Am. Chem. Soc., 2011, 133, 1760 CrossRef CAS PubMed.
- T. K. Beng and A. Moreno, RSC Adv., 2020, 10, 8805–8809 RSC.
- S. Thapa, B. Shrestha, S. K. Gurung and R. Giri, Org. Biomol. Chem., 2015, 13, 4816–4827 RSC.
- A. S.-K. Tsang, A. Kapat and F. Schoenebeck, J. Am. Chem. Soc., 2016, 138, 518 CrossRef CAS PubMed.
- K. Brak and J. A. Ellman, Org. Lett., 2010, 12, 2004–2007 CrossRef CAS PubMed.
- B. Ye and N. Cramer, Acc. Chem. Res., 2015, 48, 1308–1318 CrossRef CAS PubMed.
- P. K. Prasad, R. N. Reddi and A. Sudalai, Org. Lett., 2016, 18, 500–503 CrossRef CAS PubMed.
- A. Deb, S. Manna, A. Modak, T. Patra, S. Maity and D. Maiti, Angew. Chem., Int. Ed., 2013, 52, 9747–9750 CrossRef CAS PubMed.
- D. T. Smith and J. T. Njardarson, Angew. Chem., Int. Ed., 2014, 53, 4278–4280 CrossRef CAS PubMed.
- T. Liang, C. N. Neumann and T. Ritter, Angew. Chem., Int. Ed., 2013, 52, 8214–8264 CrossRef CAS PubMed.
- K. Muller, C. Faeh and F. Diederich, Science, 2007, 317, 1881–1886 CrossRef PubMed.
- A. Chatterjee and N. Adityachaudhury, J. Org. Chem., 1962, 27, 309–310 CrossRef CAS.
- J. B. Pedley, R. D. Naylor and S. B. Kirby, Thermochemical Data of Organic Compounds, 2nd edn, Chapman and Hall, New York, 1986 Search PubMed.
-
(a) P. A. Wender and B. L. Miller, Nature, 2009, 460, 197–201 CrossRef CAS PubMed;
(b) B. M. Trost, Acc. Chem. Res., 2002, 35, 695 CrossRef CAS PubMed;
(c) B. M. Trost, Science, 1991, 254, 1471 CrossRef CAS PubMed.
-
(a) S. Mandal, S. K. Ghosh, P. Sar, A. Ghosh and R. Saha, RSC Adv., 2016, 6, 69605–69614 RSC;
(b) M. Pagliaro, R. Ciriminna, H. Kimura, M. Rossi and C. D. Pina, Angew. Chem., Int. Ed., 2007, 46, 4434–4440 CrossRef CAS PubMed;
(c) W. H. Miles and K. B. Connell, J. Chem. Educ., 2006, 83, 285–286 CrossRef CAS.
-
(a) R. M. P. Veenboer and S. P. Nolan, Green Chem., 2015, 17, 3819–3825 RSC;
(b) A. B. Cuenca, G. Mancha, G. Asensio and M. Medio-Simón, Chem.–Eur. J., 2008, 14, 1518–1523 CrossRef CAS PubMed;
(c) J. Kim, D.-H. Lee, N. Kaluntharage and C. S. Yi, ACS Catal., 2014, 4, 3881–3885 CrossRef CAS;
(d) G. V. M. Sharma and A. K. Mahalingam, J. Org. Chem., 1999, 64, 8943–8944 CrossRef CAS PubMed;
(e) Z. Zhu and J. H. Espenson, J. Org. Chem., 1996, 61, 324–328 CrossRef CAS;
(f) O. V. Khazipov, D. V. Nykytenko, T. V. Krasnyakova, A. N. Vdovichenko, D. S. F. Frias and S. A. Mitchenko, J. Mol. Catal. A: Chem., 2017, 426, 490–498 CrossRef CAS;
(g) Q. Xu, H. Xie, P. Chen, L. Yu, J. Chen and X. Hu, Green Chem., 2015, 17, 2774–2779 RSC.
-
(a) B. N. Moghadam, B. Akhlaghinia and S. Rezazadeh, Res. Chem. Intermed., 2016, 42, 1487–1501 CrossRef CAS;
(b) L. Busetto, R. Mazzoni, M. Salmi, S. Zacchini and V. Zanotti, RSC Adv., 2012, 2, 6810–6816 RSC;
(c) A. Mirzaei, S. Biswas and J. S. M. Samec, Synthesis, 2012, 44, 1213–1218 CrossRef CAS;
(d) V. Namboodiri and R. S. Varma, Tetrahedron Lett., 2002, 43, 4593–4595 CrossRef CAS;
(e) P. Salehi, N. lranpoor and F. K Behbahani, Tetrahedron, 1998, 54, 943–948 CrossRef CAS.
- A. Fürstner, ACS Cent. Sci., 2016, 2, 778–789 CrossRef PubMed.
- K. Hovenkotter, H. Braunstein, S. Langevin and T. K. Beng, Org. Biomol. Chem., 2017, 15, 1217–1221 RSC.
-
(a) K. Ponsold and H. Kasch, Tetrahedron Lett., 1977, 46, 4463–4464 Search PubMed;
(b) G. Pandey, S. Pal and R. Laha, Angew. Chem., Int. Ed., 2013, 52, 5146–5149 CrossRef CAS;
(c) H. Im, D. Kang, S. Choi, S. Shin and S. Hong, Org. Lett., 2018, 20, 7437–7441 CrossRef CAS PubMed;
(d) Y. Li and W. Bao, Adv. Synth. Catal., 2009, 351, 865–868 CrossRef CAS;
(e) S. Y. Zhang, H. Gang, Y. Zhao, K. Wright, W. A. Nack and G. Chen, J. Am. Chem. Soc., 2012, 134, 7313–7316 CrossRef CAS PubMed;
(f) F.-J. Chen, S. Zhao, F. Hu, K. Chen, Q. Zhang, S.-Q. Zhang and B.-F. Shi, Chem. Sci., 2013, 4, 4187–4192 RSC;
(g) G. Shan, X. Y. Yang and Y. Rao, Angew. Chem., Int. Ed., 2013, 52, 13606–13610 CrossRef CAS.
- T. Cernak, K. D. Dykstra, S. Tyagarajan, P. Vachal and S. W. Krska, Chem. Soc. Rev., 2016, 45, 546–576 RSC.
- H. Hu, S. Chen, M. Mandal, S. M. Pratik, J. A. Buss, S. W. Krska, C. J. Cramer and S. S. Stah, Nat. Catal., 2020, 3, 358–367 CrossRef CAS PubMed.
Footnote |
† Electronic supplementary information (ESI) available: Experimental procedures and spectroscopic data. See DOI: 10.1039/d0ra03726e |
|
This journal is © The Royal Society of Chemistry 2020 |