DOI:
10.1039/D0RA03664A
(Paper)
RSC Adv., 2020,
10, 18355-18359
Combining amino acids and carbohydrates into readily biodegradable, task specific ionic liquids†
Received
29th March 2020
, Accepted 28th April 2020
First published on 19th May 2020
Abstract
The growing interest in the application of ionic liquids (ILs) with simultaneous sustainability draws attention to their environmental impact in general and their biodegradability in particular. Considering this, we designed a series of novel bio-ionic liquids based on natural, abundant compounds: a carbohydrate [Carb], as the cation, and amino acids [AA], as the anions; these ILs can serve as viable alternatives to the well-known and utile cholinium AAILs. Several [Carb][AA] ILs were characterized by 1H and 13C NMR, mass spectrometry, thermogravimetry (TGA) and differential scanning calorimetry (DSC). The biodegradability properties of the [Carb][AA] ILs were elucidated as well and showed biodegradation readily occurred, decomposing within 5–6 days. These novel materials were successfully utilized as catalysts for the Knoevenagel condensation reaction, where conversion values of 67–94% were achieved under exceptionally mild conditions using water as the solvent and reaction times as short as 15 minutes. These sugar based ILs were easily separated and recycled.
Introduction
Increasing consumption of fossil fuels and its related impact on the environment have driven development of sustainable and environmentally friendly processes; ionic liquids (ILs) have contributed significantly to this development due to their intrinsic properties, which led to them being initially considered as “green”.1 However, poor biodegradability of conventional ILs and their notable toxicity toward the environment2 opened a route for development of biocompatible ILs (Bio-ILs) with the focus on using at least one ionic counterpart derived from biocompatible materials.3,4 Discovery of amino acid ILs (AAILs) by Fukumoto et al.5 led to intense replacement of conventional fossil cations and anions with amino acids that can be transformed into both ion types.6,7 An advance in this approach was recently observed making AAILs the most studied bio-derived ILs, with the most significant applications of these ILs coming in catalysis,8 CO2 capture,9 electrochemistry10 or energy storage.11 Additionally, amino acids reveal chirality so their IL derivatives constitute a diverse and sustainable pool of chiral solvents for application in catalysis and materials synthesis.12
On the other hand, despite origins of bio-ILs from biocompatible materials, they may not be fully biodegradable. Biodegradation aspects of different ILs classes were summarized in an extensive review by Jordan and Gathergood, who defined several principles necessary to obtain readily biodegradable ILs, i.e. combination of hydroxyl functionalities and carboxylate counterions.13 The cholinium family of ILs has emerged here as the most readily biodegradable ILs with nearly 50% of all readily biodegradable ILs comprising a cholinium cation and an organic acid anion.13 Choline-based ILs have also been combined with amino acids, whose low viscosities and ease of preparation have found a wide range of applications.14–16
Among other bio-renewable compounds facile for bio-ILs synthesis are carbohydrates, which due to abundance, renewability and environmental friendliness, have very recently been demonstrated as great candidates toward novel applications, which make use of their task-specific properties originating from rich hydrogen-bond network structure. In specific, carbohydrate-based ILs were used for metal complexation in thermochromic systems.17 Moreover, they were applied to catalytically enhance Diels–Alder transformations, where ILs possessing a carbohydrate cation were used as both the catalyst and the reaction solvent.18 Recently, the ability of sugar to form hydrogen bonds was used to favor gel formation between [NTf2−]-based ILs and gluconate-based ILs; in addition, it was further used for desulfurization of fuels.19 The coordinating abilities of carbohydrate-based ILs were also used for cellulose dissolution, while a combination of sugars with nitrogen rich ionic moieties resulted in precursors for N-doped carbons.20 Moreover, the presence of several OH groups on carbohydrate units contributed to a decrease in the toxicity of their corresponding ILs, making sugar moieties highly desirable functionalization agents from a “green chemistry” point of view.21
Considering the strategy of using both anionic and cationic components derived from biocompatible materials, principles for synthesis of biodegradable ionic systems outlined by Jordan and Gathergood and promising properties of carbohydrate-based ILs, we designed and developed readily biodegradable ILs by the first combination of carbohydrates (Carb) and amino acids (AA). Here, we report a comprehensive study reveling their thermal analysis and biodegradation patterns. Moreover, we demonstrate the catalytic performance of [Carb][AA] ILs towards the Knoevenagel condensation, featuring materials with hydrogen-bond-rich structures and amino acid based ionic moieties as task-specific ILs.
Results and discussion
Synthesis of ILs
The synthesis of ILs was investigated using natural, abundant carbohydrate compound (glucose) and seven amino acids (L-glycine, L-leucine, L-serine, L-arginine, L-tyrosine, L-histidine, L-tryptophan). ILs were prepared using acylated N-[2-(D-glucopyranosyl)ethyl]-N,N,N-trimethylammonium bromide and a previously published procedure from our group.22 The bromide salt was transformed into the corresponding hydroxide using an ion exchange resin followed by neutralization with an amino acid as depicted in Scheme 1. That step was accompanied by acyl group hydrolysis to yield carbohydrate/amino acid-based ILs with yields of 67–87% and those structures are depicted in Fig. 1.
 |
| Scheme 1 General route for the synthesis of carbohydrate/amino acid-based ILs. Reaction conditions: (i) anion exchange resin; (ii) amino acid (1.2 eq.), ethanol, RT, 12–48 h. | |
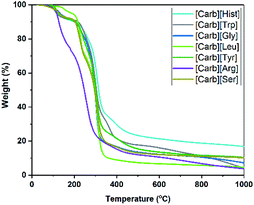 |
| Fig. 1 Thermogravimetric analysis curves of carbohydrate/amino acid-based ILs. | |
These ionic liquids (of the type [Carb][AAILs]) were very viscous; their viscosities were two orders of magnitude higher than corresponding cholinium ILs (Table 1).23,24 The profound influence on the increased viscosity had got the cation with multiple hydroxyl groups, responsible for hydrogen interactions. On the other hand, the differences in the viscosity among the particular IL types can be ascribed to larger anions and hence stronger intermolecular interactions (van der Waals, hydrogen bonding or π-stacking for derivatives with aromatic moieties). The IL with the lowest viscosity, similar to corresponding cholinium derivative was [Carb][Gly], while the highest was [Carb][Tyr]. The new ionic liquids were quite soluble in water and methanol, but not in other polar solvents like acetonitrile, ethyl acetate or DMF.
Table 1 [Carb][AA] ILs properties
ILs |
Tg (°C) |
Td (°C) |
η/(mPa s) |
50 °C |
70 °C |
[Carb][Gly] |
−19 |
198 |
32 211 |
10 196 |
[Carb][Ser] |
−18 |
198 |
147 953 |
28 645 |
[Carb][Leu] |
4 |
208 |
— |
359 841 |
[Carb][Arg] |
−15 |
107 |
641 665 |
247 626 |
[Carb][His] |
−9 |
207 |
159 815 |
106 930 |
[Carb][Trp] |
0 |
211 |
— |
408 284 |
[Carb][Tyr] |
6 |
209 |
17 700 353 |
1 476 023 |
Thermal properties
Differential scanning calorimetric measurements showed no melting points for these compounds, but glass transition temperatures (Tg) were observed and ranged from −19 to 6 °C (Table 1). The systems were stable up to 198 °C with the exception of [Carb][Arg], which decomposed at 107 °C (Td).
Thermogravimetric analysis curves of carbohydrate/amino acid based ILs are depicted in Fig. 2 with data presented in Table 1. As reported previously,5,23 the AAILs Tg and Td values depended on the side-chain structure of the amino acid. The lowest Tg was observed for [Carb][Gly] (−19 °C), while elongating the chain resulted in higher Tg values. Similarly, the Td values corresponded well to this trend with the exception of [Carb][Arg], which showed reduced stability. Compared to imidazolium based AAILs, these novel ILs showed slightly lower thermal stabilities (up to 211 °C),5 though higher than the corresponding cholinium AAILs.23,24
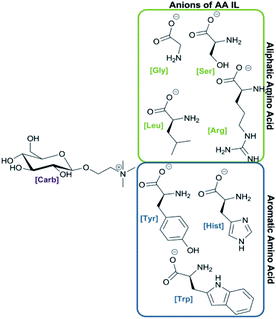 |
| Fig. 2 Chemical structures of new carbohydrate/amino acid based ILs. | |
Biodegradability
Biodegradability of novel materials is an important parameter that influences their environmental soundness. We determined whether these novel amino acid – sugar-based ILs readily decompose in controlled artificial waste waters. In general, it is worth noticing that biomass derived components, that are used to build ionic liquids, highly contribute to biodegradability increase, even when they are accompanied by a non-biodegradable component (i.e. tetrabutylammonium accompanied with a sugar25 or a fatty acid26). In our case both the hydroxy bond donors (HBD) and acceptors (HBA) were naturally sourced. Studies performed with activated sludge (Fig. 3 and ESI†) revealed that all ILs decomposed within 5–6 days. Therefore, all of these new derivatives can be classified as readily biodegradable.13 There were no obvious differences in degradation rates of the sugar component nor the amino acids for any IL (except for histidine). Also we did not notice any negative impact of HBD to HBA and vice versa on biodegradation rates, which correlated to other studies.13 Similarly, ionic liquids constructed with choline and amino acids were shown to be readily biodegradable, where authors used a closed bottle and CO2 headspace test accompanied with monitoring of the depletion of dissolved O2.27
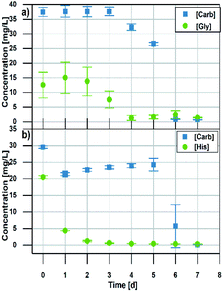 |
| Fig. 3 Biodegradation of (a) aliphatic (b) aromatic amino acid/carbohydrate-based ILs. | |
Application example
These novel ILs were investigated as organocatalysts in the Knoevenagel condensation, a facile method for the synthesis of valuable α,β-unsaturated carbonyl compounds widely utilized in the pharmaceutical industry.28 Amino acids catalyze the Knoevenagel condensation29 with various activities leading to conversions from 2 to 92% after 1 h using DMSO as the solvent.30 Recent studies have shown that transformation of an amino acid into an IL may lead to enhanced catalytic performance due to the role of the amino acid anion in the reaction mechanism.31 Thus, using amino acids which had shown poor catalytic activity towards the condensation reaction (such as Tyr 3%, His 6%, Leu 9%), resulted in reaction time reductions to 30 minutes and conversions increased to 80–89% when these amino acids were incorporated as ILs.31 In the Knoevenagel condensation, AAILs possessing an anionic amino acid moiety and bound with tetrabutylammonium31 and cholinium cations,32 or possessing a cation with an amino acid amide anion33 were investigated and served as easily separable and recyclable catalysts and solvents/co-solvents.
The catalytic performance of the carbohydrate AAILs was tested in the model reaction of benzaldehyde with malononitrile in water as the solvent/co-solvent. Application of water-soluble carbohydrate-based AAILs facilitated an easy separation of water-insoluble condensation product and readily recycling of the [Carb][AA] ILs for the next reaction cycle. The influence of the catalytic amounts of the IL on the example of [Carb][Leu], on the conversion of benzaldehyde is presented in Table 2. Furthermore, the influence of all investigated ILs on reaction rates are given in Fig. 4a. In the presence of all carbohydrate AAILs, high conversions of benzaldehyde (67–92%) were obtained immediately after 15 minutes with a 10% catalyst loading. The type of AAILs influenced the initial reaction rate with their activity corresponding to the activity of amino acids in the Knoevenagel condensation. In contrast to pristine amino acids, the use of AAILs resulted in shortened reaction times to 15 minutes. Longer reaction times did not improve product yields except for [Carb][Gly], which yielded a conversion of 94% after 30 min (only 67% after 15 min). The performance of investigated systems compared to other amino acid catalysts used in the Knoevenagel condensation is provided in the Table 1, ESI.† The products were exclusively E isomers, obtained with 100% selectivity. After separation of the water insoluble product, the aqueous phase was extracted with CH2Cl2 and reused in the next reaction cycle. Over four consecutive runs, no conversion drop was observed (Fig. 4b). Application of highly water soluble, hydrogen-bond-rich [Carb][AA] ILs in the Knoevenagel condensation afforded a clean, mild synthetic approach with the added benefit of product separation ease and short reaction time. Presented approach adhere to the Principles of Green Chemistry to a good extent, with Atom Economy (AE) of 90%, Reaction Mass Efficiency (RME) of 82%, Optimum Efficiency (OE) of 92% and Process Mass Intensity (PMI) of 15.3.34
Table 2 The influence of catalytic amounts of the IL on the conversion of benzaldehydea
Catalyst loading (mol%) |
Conversion (%) |
Reaction conditions: 25 °C; 1000 rpm; benzaldehyde (1 mmol, 0.106 g); malononitrile (1 mmol, 0.661 g); H2O 2 mL, catalyst: [Carb][Leu] (mol%); 15 min. |
0.5 |
24 |
1 |
37 |
2.5 |
73 |
5 |
84 |
10 |
92 |
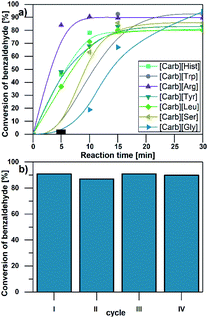 |
| Fig. 4 (a) Influence of the amino acid anion on the conversion of benzaldehyde in the reaction with malononitrile. (b) Yield of 2-benzylidenomalononitrile from condensation of benzaldehyde with malononitrile (25 °C, 10% catalyst loading, 30 min) in consecutive recycling cycles. | |
Experimental
Synthesis of ILs together with the characterization data is provided in ESI (S2).†
Characterization
1H NMR spectra were recorded at 400 MHz and 13C spectra were recorded at 100 MHz (Agilent spectrometer). Chemical shifts (ppm) are reported relative to tetramethylsilane (TMS) as an internal standard. High-resolution mass spectrometry (HRMS) analyses were performed on a Waters Xevo G2 Q-TOF mass spectrometer equipped with an ESI source operating in the positive negative ion mode. Accurate mass and molecular ion compositions were calculated using the MassLynx software incorporated with the instrument. Thermogravimetric analyses (TGA) were performed using a Mettler-Toledo TGA/DSC 3+ thermobalance. Samples (approximately 5–10 mg) were heated from 25–900 °C at a rate of 10 °C min−1 in standard 70 μL Al2O3 crucibles under a dynamic nitrogen flow of 80 mL min−1. Differential scanning calorimetry (DSC) were performed using a Mettler-Toledo DSC 822 analyzer. Samples were heated from −65–150 °C at a rate of 10 °C min−1 in standard 40 μL Al crucibles under a dynamic nitrogen flow of 80 mL min−1. Viscosity measurements were carried out using a Brookfield RST-CPS rheometer with a cone and plate measuring system. Due to high viscosities, samples were analyzed at 70 °C, and where it was possible at 50 °C.
Biodegradation test
Biodegradation studies were based on principles outlined in the OECD 301 test. Briefly, an adapted minimal salt medium (MSM) was used [0.085 g L−1 KH2PO4, 0.217 g L−1 K2HPO4, 0.34 g L−1 Na2HPO4·2H2O, 0.005 g L−1 NH4Cl, 0.0364 g L−1 CaCl2·2H2O, 0.0225 g L−1 MgSO4·7H2O, 0.00025 g L−1 FeCl3·6H2O]. Activated sludge (AS) was sourced from Waterworks Kraków, Poland. The inoculum (300 mL of AS) was preconditioned in the MSM (700 mL) for five days in the absence of a carbon source, with vigorous shaking (200 rpm) at a constant temperature of 20 °C in the absence of light. For the degradation studies, IL samples (50 mg L−1 in MSM) were inoculated with preconditioned activated sludge (30 mg L−1). Experiments were conducted with vigorous shaking (200 rpm) at a constant temperature of 20 °C in the dark for 21 days. During that period, samples were collected and analyzed by HPLC-MS as described below.
High pressure liquid chromatography with mass detection (HPLC-MS)
The analyses were performed by HPLC measurements on an Agilent 1290 Infinity System with an autosampler and a MS Agilent 6460 Triple Quad Detector equipped with an Agilent Zorbax Eclipse Plus C18 column (2.1 × 50 mm, 1.8 μm). To separate the IL components, the column was eluted at 30 °C, a flow rate of 0.8 mL min−1 and developed with an isocratic elution (95% solvent A, 0.1% formic acid in water; 5% solvent B, 0.1% formic acid in acetonitrile). The injection (2.5 μL) interval was 2.0 min. An MS Agilent 6460 Triple Quad tandem mass spectrometer with an Agilent Jet Stream ESI interface was used in positive ion mode for both HBDs and HBAs. Nitrogen (flow rate 10 L min−1) was used as the drying gas and for collision-activated dissociation. Drying gas and sheath gas temperatures were set to 350 °C. The capillary voltage was 3500 V; the nozzle voltage was set to 500 V. Compounds were monitored in multiple reaction monitoring mode (MRM) with the following transitions, polarity, fragmentor (F) and collision energies (CE): ESI+: carbohydrate (M + ACN + H) 266.1 → 104.1 m/z, F = 142 V, CE = 30 V; tyrosine (M + H) 182.1 → 91.1 m/z, F = 73 V, CE = 30 V; arginine (M + H) 175.1 → 70.1 m/z, F = 93 V, CE = 26 V; histidine (M + H) 156.1 → 110.1 m/z, F = 78 V, CE = 10 V; leucine (M + H) 132.1 → 86.1 m/z, F = 78 V, CE = 6 V; serine (M + H) 106.1 → 42.1 m/z, F = 64 V, CE = 22 V; tryptophan (M + H) 205.1 → 118.1 m/z, F = 73 V, CE = 26 V and glycine (M + H) 76.1 → 30.8 m/z, F = 44 V, CE = 30 V. Standard curves were prepared in a premix of solvents A and B (95
:
5% v/v) and were used for quantitative analysis. MassHunter software (Agilent) was used for HPLC-MS system control, data acquisition and data processing.
Knoevenagel condensation
All reactions were carried out in a 10 mL round bottom flask, where benzaldehyde (1 mmol), malononitrile (1 mmol), AAIL (0.1 mmol) and 2 mL of water were placed. The mixture was vigorously stirred (1000 rpm) at 25 °C for 5–60 min. Afterwards, the mixture was homogenized with 5 mL of ethanol and the benzaldehyde conversion was monitored by GC-FID analysis with n-decane as an internal standard (50 μL of sample diluted with 1.5 mL of ethanol).
IL recycling
In typical IL recycling experiments, reactions were scaled up by a factor of ten. A mixture of benzaldehyde (10 mmol), malononitrile (10 mmol) and IL (10 mol%) were stirred in water for 20 min at 25 °C. The solid product was separated and the aqueous phase was extracted with CH2Cl2 (4 × 25 mL) and reused in the next reaction.
Conclusions
Novel bio-ILs were synthesized using nontoxic, biodegradable and abundant materials (amino acids and glucose). A series of [Carb][AA] ILs was synthesized by neutralization of an N-[2-(D-glucopyranosyl)ethyl]-N,N,N-trimethylammonium hydroxide solution with seven different amino acids. Replacement of conventional, petroleum-derived imidazolium/quaternary ammonium cations with carbohydrates improved biodegradability of the ILs; most of the novel ILs decomposed within 5 to 6 days in an activated sludge environment which categorizes them as readily biodegradable. These ILs were successfully applied as catalysts for the Knoevenagel condensation reaction; conversion yields of 67–94% were achieved under exceptionally mild conditions. The use of these water soluble AAILs afforded easy separation of the products insoluble in water. Sugar based AAILs can serve as viable alternatives to cholinium AAILs for different task-specific applications and utilize their hydrogen-bond-rich structures.
Conflicts of interest
There are no conflicts to declare.
Acknowledgements
This work was financed by the National Science Centre Poland (Grant no. UMO-2018/29/B/ST8/01784). We greatly acknowledge the joint consortium “Interdisciplinary Centre of Physical, Chemical and Biological Sciences” of ICSC PAS and INP PAS for providing access to the Agilent 1290 Infinity System with the autosampler and MS Agilent 6460 Triple Quad Detector.
Notes and references
- M. Kar, K. Matuszek, and D. R. MacFarlane, Kirk-Othmer Encyclopedia of Chemical Technology, 2019, p. 1 Search PubMed.
- J. Pernak, J. Rogoża and I. Mirska, Eur. J. Med. Chem., 2001, 36, 313–320 CrossRef CAS PubMed.
- J. Hulsbosch, D. E. De Vos, K. Binnemans and R. Ameloot, ACS Sustainable Chem. Eng., 2016, 4, 2917–2931 CrossRef CAS.
- J. M. Gomes, S. S. Silva and R. L. Reis, Chem. Soc. Rev., 2019, 48, 4317–4335 RSC.
- K. Fukumoto, M. Yoshizawa and H. Ohno, J. Am. Chem. Soc., 2005, 127, 2398–2399 CrossRef CAS PubMed.
- L. He, G.-H. Tao, D. A. Parrish and J. M. Shreeve, J. Phys. Chem. B, 2009, 113, 15162–15169 CrossRef CAS PubMed.
- Y. Qian, S. Xiao, L. Liu and Y. Wang, Tetrahedron: Asymmetry, 2008, 19, 1515–1518 CrossRef CAS.
- M. P. Pereira, R. de Souza Martins, M. A. L. de Oliveira and F. I. Bombonato, RSC Adv., 2018, 8, 23903–23913 RSC.
- F.-F. Chen, K. Huang, Y. Zhou, Z.-Q. Tian, X. Zhu, D.-J. Tao, D. Jiang and S. Dai, Angew. Chem., Int. Ed., 2016, 55, 7166–7170 CrossRef CAS PubMed.
- X. Lu, X. Wang, J. Jin, Q. Zhang and J. Chen, Biosens. Bioelectron., 2014, 62, 134–139 CrossRef CAS PubMed.
- H. Zhou, Y. Zhou, L. Li, Y. Li, X. Liu, P. Zhao and B. Gao, ACS Sustainable Chem. Eng., 2019, 7, 9281–9290 CrossRef CAS.
- F. Tang, Q. Zhang, D. Ren, Z. Nie, Q. Liu and S. Yao, J. Chromatogr. A, 2010, 1217, 4669–4674 CrossRef CAS PubMed.
- A. Jordan and N. Gathergood, Chem. Soc. Rev., 2015, 44, 8200–8237 RSC.
- R. Wang, Y. Chang, Z. Tan and F. Li, Sep. Sci. Technol., 2016, 51, 1093–1102 CrossRef.
- Z. Zhang, N. Kang, J. Zhou, X. Li, L. He and H. Sui, Nanomaterials, 2019, 9, 504 CrossRef CAS PubMed.
- S. Bhattacharyya and F. U. Shah, ACS Sustainable Chem. Eng., 2016, 4, 5441–5449 CrossRef CAS.
- F. Billeci, H. N. Gunaratne, F. D'Anna, G. G. Morgan, K. R. Seddon and N. V. Plechkova, Green Chem., 2019, 21, 1412–1416 RSC.
- K. Erfurt, I. Wandzik, K. Walczak, K. Matuszek and A. Chrobok, Green Chem., 2014, 16, 3508–3514 RSC.
- F. Billeci, F. D'Anna, H. N. Gunaratne, N. V. Plechkova and K. R. Seddon, Green Chem., 2018, 20, 4260–4276 RSC.
- A. Brzeczek-Szafran, K. Erfurt, A. Blacha-Grzechnik, M. Krzywiecki, S. Boncel and A. Chrobok, ACS Sustainable Chem. Eng., 2019, 7, 19880–19888 CrossRef CAS.
- F. Billeci, F. D’Anna, M. Feroci, P. Cancemi, S. Feo, A. Forlino, F. Tonnelli, K. R. Seddon, H. N. Gunaratne and N. V. Plechkova, ACS Sustainable Chem. Eng., 2020, 8, 926–938 CrossRef CAS.
- J. Pernak, K. Czerniak, A. Biedziak, K. Marcinkowska, T. Praczyk, K. Erfurt and A. Chrobok, RSC Adv., 2016, 6, 52781–52789 RSC.
- Q.-P. Liu, X.-D. Hou, N. Li and M.-H. Zong, Green Chem., 2012, 14, 304–307 RSC.
- D.-J. Tao, Z. Cheng, F.-F. Chen, Z.-M. Li, N. Hu and X.-S. Chen, J. Chem. Eng. Data, 2013, 58, 1542–1548 CrossRef CAS.
- N. Ferlin, M. Courty, S. Gatard, M. Spulak, B. Quilty, I. Beadham, M. Ghavre, A. Haiß, K. Kümmerer, N. Gathergood and others, Tetrahedron, 2013, 69, 6150–6161 CrossRef CAS.
- K. Haraźna, K. Walas, P. Urbańska, T. Witko, W. Snoch, A. Siemek, B. Jachimska, M. Krzan, B. D. Napruszewska, M. Witko and others, Green Chem., 2019, 21, 3116–3126 RSC.
- X.-D. Hou, Q.-P. Liu, T. J. Smith, N. Li and M.-H. Zong, PLoS One, 2013, 8, e59145 CrossRef CAS PubMed.
- Y. Ono, J. Catal., 2003, 216, 406–415 CrossRef CAS.
- F. S. Prout, J. Org. Chem., 1953, 18, 928–933 CrossRef CAS.
- Y.-H. He, Y. Hu and Z. Guan, Synth. Commun., 2011, 41, 1617–1628 CrossRef CAS.
- P. Ossowicz, Z. Rozwadowski, M. Gano and E. Janus, Pol. J. Chem. Technol., 2016, 18, 90–95 CAS.
- P. Moriel, E. J. Garcia-Suarez, M. Martinez, A. B. Garcia, M. Montes-Morán, V. Calvino-Casilda and M. A. Bañares, Tetrahedron Lett., 2010, 51, 4877–4881 CrossRef CAS.
- P. A. Burate, B. R. Javle, P. H. Desale and A. K. Kinage, Catal. Lett., 2019, 149, 2368–2375 CrossRef CAS.
- C. R. McElroy, A. Constantinou, L. C. Jones, L. Summerton and J. H. Clark, Green Chem., 2015, 17, 3111–3121 RSC.
Footnote |
† Electronic supplementary information (ESI) available: Synthetic procedures, NMR spectra, biodegradation patterns. See DOI: 10.1039/d0ra03664a |
|
This journal is © The Royal Society of Chemistry 2020 |