DOI:
10.1039/D0RA03487H
(Paper)
RSC Adv., 2020,
10, 25100-25106
A novel catalytic kinetic method for the determination of mercury(II) in water samples
Received
18th April 2020
, Accepted 27th May 2020
First published on 1st July 2020
Abstract
Mercury(II) ions act as catalyst in the substitution of cyanide ion in hexacyanoruthenate(II) by pyrazine (Pz) in an acidic medium. This property of Hg(II) has been utilized for its determination in aqueous solutions. The progress of reaction was followed spectrophotometrically by measuring the increase in absorbance of the yellow colour product, [Ru(CN)5Pz]3− at 370 nm (λmax, ε = 4.2 × 103 M−1 s−1) under the optimized reaction conditions; 5.0 × 10−5 M [Ru(CN)64−], 7.5 × 10−4 M [Pz], pH 4.00 ± 0.02, ionic strength (I) = 0.05 M (KCl) and temp. 45.0 ± 0.1 °C. The proposed method is based on the fixed time procedure under optimum reaction conditions. The linear regression (calibration) equations between the absorbance at fixed times (t = 15, 20 and 25 min) and [Hg(II)] were established in the range of 1.0 to 30.0 × 10−6 M. The detection limit was found to be 1.5 × 10−7 M of Hg(II). The effect of various foreign ions on the proposed method was also studied and discussed. The method was applied for the determination of Hg(II) in different wastewater samples. The present method is simple, rapid and sensitive for the determination of Hg(II) in trace amount in the environmental samples.
1. Introduction
Mercury (Hg) occurs naturally in the earth's crust.1 Its concentration increases in the environment and water bodies due to the natural processes but mostly accelerated by the anthropogenic activities from pesticides, paints, batteries, industrial waste and land application of industrial or domestic sludge.1,2 Thus, it gradually concentrates and moves away through the surface run-off transporting to the aquatic systems as rivers, lakes, seas and oceans.2,3 Antagonistically to the organic contaminants, heavy metals such as Hg does not undergo microbial or chemical degradation and thus persist for a long time in the environment.3,4 Therefore, heavy metals including Hg are the most hazardous pollutants to the environment because of the rapid industrialization and urbanization.4 Thus, there has been continuous considerable emphasis on the Hg analysis in different type of samples.5 Among the various heavy metals, particularly Hg has been listed as a priority pollutant by the international environmental and health agencies because of its persistence, bioaccumulation and toxicity (PBT) in the environment.6 It not only causes serious health problems but also poses a great challenge to the environmental protection.7,8 Among other pollutants, Hg is of major concern in aquatic environments. Thus, Hg is considered as a global and recalcitrant pollutant due to its biogeochemical properties and its toxicity that can affect the health of human and ecosystems.4
Though metallic Hg is an insoluble element, it is easily oxidized to the soluble ionic form Hg2+ in the freshwater reservoirs and constitute a serious threat through the process of biomagnification.9,10 Thus, mercury is of major concern in aquatic environments.11 The consumption of contaminated fish, sea mammals and ground water are the prominent environmental sources of Hg exposure in humans.11,12 It forms quite stable complexes with sulfhydryl (–SH) groups in the human body forming mercaptides having mobility through the tissues,13 which lead to the inactivation of numerous enzyme reactions, amino acids and sulfur containing antioxidants such as N-acetyl cysteine (NAC), alpha-lipoic acid (ALA), and glutathione (GSH) and makes it quite toxic to the human being (cf. following general reaction).14
2R–SH + Hg2+ → (R–S)2Hg + 2H+ |
The accumulation of Hg in the body is associated with hazardous health effects, such as gastrointestinal and nervous system disorders, respiratory and acute renal failures, hypertension, coronary heart disease (CHD), and cardiovascular disease (CVD).15 Thus, even a small amount of Hg can have adverse effects on the human health.16 One of the most stable forms of Hg is the highly toxic, water-soluble, divalent mercuric ion (Hg2+), which is widely distributed in soil, air and aquatic environments as a result of illegal waste release from various anthropogenic and industrial activities. Hg2+ can cause serious environmental pollution and result in permanent damage to the biological organisms due to its acute and/or chronic toxicity.17 Thus, the health and environmental issues associated with Hg2+ has stimulated researchers to develop inexpensive, effective and reliable methods for the detection and determination of Hg2+ sensitively and selectively.5,7,8,17,18
Attempts have been made to determine Hg in the environmental,18 geological,19 food20,21 and biomedical22,23 samples. Majority of the analytical methods proposed for the determination of Hg are based on the sophisticated techniques viz. cold vapor atomic absorption spectrometry (CV-AAS),23–25 inductively coupled plasma mass spectrometry (ICP-MS),26,27 atomic fluorescence spectrometry (AFS),28,29 anodic stripping voltammetry (ASV),30–32 high performance liquid chromatography (HPLC),33,34 etc. All these techniques require expensive instrumentation, pre-concentration for the enrichment of analyte and its separation from the other constituents of the sample to minimize the matrix effect prior to their application. In addition, skilled operators, high cost, long time for running per sample, etc., have been other disadvantages. To overcome these problems, attempts have been made to develop analytical methods based on the kinetics and catalysis,5,18,35–38 formation of colored complexes of Hg(II) with other compounds,39,40 etc. We have also been interested in developing analytical methods for the determination as well as removal of various analytes and toxic species of environmental, biological and medicinal interest.5,18,35–38,41,42 Keeping the above explained backgrounds in mind, in the present communication, a successful attempt has been made to develop a novel, simple and precise catalytic kinetic method (CKM) for the trace level determination of Hg(II) in the aqueous samples which is based on the Hg(II) catalyzed substitution of (CN−) in the [Ru(CN)6]4− by pyrazine (Pz). The kinetics and mechanism of this reaction has already been reported by us43 and has now been exploited as an indicator reaction system for the CKM in the determination of Hg(II). The developed CKM has successfully been utilized for the determination of Hg(II) in the wastewater samples.
2. Experimental
2.1 Reagents and instrumentation
All the reagents used were of analytical grade and deionized distilled water (DDW) was used throughout the study for preparation of all the solutions. The stock solutions (1.0 × 10−2 M) of K4[Ru(CN)6]·3H2O, Pz and HgCl2 were prepared by dissolving their appropriate amounts in DDW. K4[Ru(CN)6]·3H2O solution was kept in a dark amber colour volumetric flask to prevent its photodecomposition. The working solutions of these reagents were prepared by their appropriate dilution from the respective stock solutions as required. Potassium hydrogen phthalate (PHP)–HCl buffer of pH 4.00 ± 0.02 was prepared according to the literature reported method.44
Kinetic measurements and recording of various spectral scans were carried out in 10 mm matched quartz cuvettes using a Shimadzu UV-240 double beam spectrophotometer equipped with a self-designed thermostatic cell compartment. A remi ultra-cryostat was used to maintain the temperature of the reaction system. All the pH measurements were made on a Toshniwal digital pH meter model CL46. A certified ‘A’ grade volumetric apparatus were used throughout the work.
2.2 Procedure
All the required solutions were first placed in the thermostat maintained at 45.0 ± 0.1 °C for 30 min prior to their use to attain thermal equilibrium. 2.0 mL each of the solutions of Pz, buffer, HgCl2 and K4[Ru(CN)6] was pipetted out and mixed in a 10 mL volumetric flask, which was also placed in the same thermostat, shaken quickly and transferred immediately into a 10 mm spectrophotometric cuvette placed in the cell compartment of the spectrophotometer at 45.0 ± 0.1 °C. The progress of the reaction was monitored spectrophotometrically by measuring the increase in absorbance due to the formation of the product [Ru(CN)5Pz]3− at its λmax of 370 nm. A fixed time procedure was used to record the absorbance as a function of the concentration of Hg(II).The cuvettes were cleaned with acetone after few kinetic runs in order to remove the deposited intense yellow-colored [Ru(CN)5Pz]3− complex.
3. Results & discussion
3.1 The indicator reaction
The detailed kinetics and mechanism of Hg(II) catalyzed substitution of CN− in the [Ru(CN)6]4− by Pz have been studied and reported by us earlier.43 The reaction product, [Ru(CN)5Pz]3− complex has strong absorption band at 370 nm and molar extinction coefficient ε = 4.2 × 103 M−1 s−1. The UV-visible spectra of the reactants and the product shown in Fig. 1 clearly shows no interference between the absorbance of the product and reactants i.e. [Ru(CN)6]4− and Pz. Hence, the progress of the reaction was easily followed spectrophotometrically by measuring the increase in absorbance at 370 nm i.e. λmax of [Ru(CN)5Pz]3− without making any corrections for the absorbance due to the reactants [Ru(CN)6]4− and Pz.
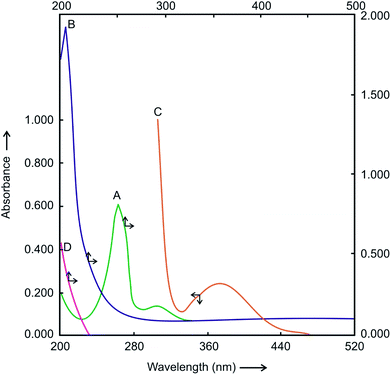 |
| Fig. 1 UV-visible absorption spectra of reactants and products under the conditions: (A) [Pz] = 2.5 × 10−6 M, (B) [Ru(CN)64−] = 1.25 × 10−5 M, (C) product [Ru(CN)5Pz3−] formed by mixing [Ru(CN)64−] = 5.0 × 10−5 M, [Pz] = 7.5 × 10−4 M, [Hg(II)] = 1.5 × 10−4 M, I = 0.05 M (KCl), pH = 4.00 ± 0.02 and temp. = 45.0 ± 0.1 °C, (D) [Hg(II)] = 1.0 × 10−4 M. | |
3.2 The rate law and its analytical application
Based on the kinetic and mechanistic studies,43 the overall rate of the indicator reaction comprises of the sum of the rate of the uncatalyzed as well as catalyzed reactions and is given by eqn (1). |
 | (1) |
If K[Ru(CN)64−] ≫ 1, eqn (1) reduces to eqn (2) as follows:
|
 | (2) |
In eqn (2), first term on the right hand side corresponds to the rate of the uncatalyzed path and
involves the rate constant for the slow decomposition of the activated complex (formed between [Ru(CN)6]4−, Hg2+ and H2O) and some other concentration terms.43 Eqn (2) clearly shows a direct correlation between the rate of the indicator reaction and the concentration of Hg(II) and envisages for the accurate determination of Hg(II). In the present study, the rate of the uncatalyzed reaction is almost negligible. Thus, the catalytic kinetic method (CKM) for the determination of Hg(II) based on the [Ru(CN)64−]–Pz indicator reaction will be more accurate in comparison to the other CKM involving the [Fe(CN)64−] – ligand indicator reactions.35–38
The increase in absorbance (At) at different time t (t = 15, 20 and 25 min) after mixing the reactants was recorded as a measure of the initial rate under the optimized reaction condition as 5.0 × 10−5 M [Ru(CN)54−], 7.5 × 10−4 M [Pz], pH 4.00 ± 0.02, ionic strength (I) 0.05 M (KCl) and temperature 45.0 ± 0.1 °C,43 using fixed time procedure. The calibration curves were obtained by plotting the values of At against different Hg(II) concentrations where the corresponding linear regression equations correlating At to the [Hg(II)] obtained are shown in eqn (3)–(5).
|
A15 = 3.1 × 103[Hg2+] + 0.009
| (3) |
|
A20 = 4.0 × 103[Hg2+] + 0.012
| (4) |
|
A25 = 6.36 × 103[Hg2+] + 0.016
| (5) |
3.3 Quantitative determination of Hg(II)
In order to validate the analytical applicability of the proposed CKM, recovery experiments were performed in various water samples spiked with Hg(II) and the results obtained for the three fixed times along with the standard deviations and the percentage errors are shown in Table 1. From Table 1, it is clear that the calibration curve corresponding to the A15 is a closer measure to the initial rate with the minimal percentage error as compared to A20 and A25. Time less than 15 min and more than 25 min were not taken to minimize the experimental error. Based on this observation, the A15 calibration curve is recommended for the Hg(II) determination in the aqueous samples.
Table 1 Evaluation of percentage error in the determination of Hg(II) under conditions: [Ru(CN)64−] = 5.0 × 10−5 M, [Pz] = 7.5 × 10−4 M, pH = 4.00 ± 0.02, I = 0.05 M (KCl), temp. = 45.0 ± 1.0 °C
[Hg2+] × 106 M (taken) |
A15 |
A20 |
A25 |
[Hg2+] × 106 M (found)b ± SDa |
Error (%) |
[Hg2+] × 106 M (found)b ± SDa |
Error (%) |
[Hg2+] × 106 M (found)b ± SDa |
Error (%) |
The ±SD values represent the % relative standard deviation of the mean for three determinations. Mean of three determinations. |
1.00 |
0.94 ± 0.08 |
−6.00 |
0.94 ± 0.02 |
−6.00 |
1.06 ± 0.05 |
+6.00 |
2.00 |
2.25 ± 0.06 |
+12.50 |
2.00 ± 0.06 |
0.00 |
1.88 ± 0.03 |
+6.00 |
3.00 |
3.00 ± 0.08 |
0.00 |
3.05 ± 0.02 |
+1.67 |
3.06 ± 0.06 |
+2.00 |
4.00 |
4.02 ± 0.02 |
+0.50 |
4.05 ± 0.01 |
+0.80 |
4.06 ± 0.05 |
+1.50 |
5.00 |
5.05 ± 0.05 |
+1.00 |
5.05 ± 0.02 |
+1.00 |
5.03 ± 0.09 |
+6.00 |
10.00 |
10.00 ± 0.07 |
0.00 |
10.05 ± 0.06 |
+0.50 |
10.05 ± 0.06 |
+0.50 |
15.00 |
15.25 ± 0.02 |
+1.70 |
15.25 ± 0.03 |
+1.70 |
15.13 ± 0.04 |
+0.87 |
20.00 |
20.00 ± 0.03 |
0.00 |
20.02 ± 0.03 |
+0.10 |
20.25 ± 0.04 |
+1.25 |
30.00 |
29.80 ± 0.05 |
−0.67 |
29.50 ± 0.04 |
−1.70 |
30.25 ± 0.02 |
+0.83 |
The detection limit (Xd), defined as three times standard deviation of the blank, was evaluated using Tanaka's method45 using eqn (6), where Xb is the average of the blank values, (SD)b is the standard deviation of blank and equal to R/d2, R is blank kmax − blank kmin and 1/d2 is a factor for obtaining (SD)b from the range R of n replicates whose value is recommended as 0.5908 for the use in three blank measurements.
|
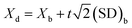 | (6) |
The detection limit for the present method corresponding to the A15 calibration curve under the optimized experimental conditions was found to be 1.5 × 10−7 M.
3.4 Interference study
The general precision and accuracy for the determination of Hg(II) by the proposed CKM was tested in the presence of several cations, anions and complexing agents. For this purpose, the recovery i.e. the determination of Hg(II) was performed using a fixed concentration of Hg(II), 4.0 × 10−6 M, in the presence of different known concentrations of individual cations, anions and complexing agents. The concentration of Hg(II) was determined using the A15 calibration eqn (3). The recovery results on the determination of 4.0 × 10−6 M Hg(II) in the presence of different individual cations, anions and complexing agents along with their concentrations have been presented in Table 2. Based on the results of Hg(II) recovered, it was confirmed that Mn2+, Ni2+, Ag+, Sn2+, Pb2+, Mg2+, Ca2+, Cd2+, Cu2+, Al3+, Fe3+, Br−, I−, NO3−, CO32−, C2O42−, SO42− ions did not interfere in the determination of Hg(II) but Li+, Co2+, Zn2+, NTA, IDA, EDTA interfered significantly at their concentrations reported in Table 2. Metal hexacyanoferrates are very efficient sorbents for the recovery of alkali metal ions and especially Li+, which leads to the formation of the corresponding lithium hexacyanoruthenate(II) complex as its Fe(II) counterpart in the solution.46 Hence, a low recovery of Hg(II) is very much expected in the presence of Li+ ions in the aqueous medium. The interference by NTA, IDA, EDTA may be attributed to the possible ligand substitution between the monodentate cyanide ligand in [Ru(CN)6]4− and the ligands viz. NTA, IDA, EDTA. Interference by Co2+, Cu2+, Zn2+ is probably due to the complex formation between these metal ions and [Ru(CN)6]4− or Pz.
Table 2 Effect of different foreign ions on the determination of Hg(II) under the conditions: [Ru(CN)64−] = 5.0 × 10−5 M, [Pz] = 7.5 × 10−4 M, [Hg2+] = 4.0 × 10−6 M, pH = 4.00 ± 0.02, I = 0.05 M (KCl), temp. = 45.0 ± 1.0 °C
Foreign ion |
[Foreign ion] (added), M |
[Hg2+] (found), M |
Error (%) |
Inference |
Mn2+ |
2 × 10−4 |
4.1× 10−6 |
+2.50 |
No inference |
Ni2+ |
4 × 10−4 |
3.9 × 10−6 |
−2.50 |
No inference |
Ag+ |
8 × 10−4 |
4.0 × 10−6 |
0.00 |
No inference |
Sn2+ |
8 × 10−4 |
4.1 × 10−6 |
+2.50 |
No inference |
Pb2+ |
4 × 10−4 |
3.8 × 10−6 |
−5.00 |
No inference |
Mg2+ |
4 × 10−4 |
4.1 × 10−6 |
+2.50 |
No inference |
Ca2+ |
4 × 10−4 |
3.9 × 10−6 |
−2.50 |
No inference |
Cd2+ |
4 × 10−4 |
4.0 × 10−6 |
0.00 |
No inference |
Cu2+ |
8 × 10−4 |
3.8 × 10−6 |
−5.00 |
No inference |
Al3+ |
4 × 10−4 |
4.0 × 10−6 |
0.00 |
No inference |
Fe3+ |
8 × 10−4 |
3.9 × 10−6 |
−2.50 |
No inference |
Li+ |
8 × 10−5 |
1.2 × 10−6 |
−70.00 |
Interfered |
Co2+ |
2 × 10−5 |
1.6 × 10−6 |
−60.00 |
Interfered |
Zn2+ |
4 × 10−5 |
1.9 × 10−6 |
−52.50 |
Interfered |
Br− |
4 × 10−4 |
4.1 × 10−6 |
+2.50 |
No inference |
I− |
8 × 10−4 |
3.9 × 10−6 |
−2.50 |
No inference |
NO3− |
8 × 10−4 |
4.0 × 10−6 |
0.00 |
No inference |
CO32− |
8 × 10−4 |
3.9 × 10−6 |
−2.50 |
No inference |
C2O42− |
4 × 10−4 |
4.0 × 10−6 |
0.00 |
No inference |
SO42− |
4 × 10−4 |
4.1 × 10−6 |
+2.50 |
No inference |
NTA |
2 × 10−4 |
2.4 × 10−6 |
−40.00 |
Interfered |
IDA |
2 × 10−4 |
2.2 × 10−6 |
−45.00 |
Interfered |
EDTA |
2 × 10−4 |
1.9 × 10−6 |
−52.50 |
Interfered |
3.5 Validation and analytical application of CKM
In order to validate the proposed method, five water spiked synthetic mixtures (SMs) were prepared, which contained other metal ions along with the catalyst Hg(II). This was followed by the determination of Hg(II) in five different synthetic mixtures (SM-1 to SM-5) using the proposed CKM. The results were further confirmed by atomic absorption spectrophotometry (AAS), as shown in Table 3. The results obtained by CKM are in close agreement with those determined by AAS (Table 3).
Table 3 Determination of Hg(II) in synthetic mixtures under optimized reaction conditions: [Ru(CN)64−] = 5.0 × 10−5 M, [Pz] = 7.5 × 10−4 M, [Hg2+] = 4.0 × 10−6 M, pH = 4.00 ± 0.02, I = 0.05 M (KCl), temp. = 45.0 ± 1.0 °C and its comparison atomic absorption spectrometry (AAS) method
Synthetic mixtures (SM) |
Composition of SM (ng mL−1) |
[Hg2+] foundb ± SDa by CKM (ng mL−1) |
Recovery (%) |
[Hg2+] foundb ± SDa by AAS (ng mL−1) |
The ±SD values represent the % relative standard deviation of the mean for three determinations. Mean of three determinations. |
SM-1 |
Hg 1086.0 + Ca 4202.0 |
1086.2 ± 1.1 |
100.02 |
1086.1 ± 4.3 |
Cu 5006.0 + Cd 4808.0 |
SM-2 |
Hg 1086.0 + Ag 1206.5 |
1085.9 ± 1.2 |
99.99 |
1085.9 ± 5.2 |
Mg 4024.0 + Ni 1435.0 |
SM-3 |
Hg 1086.0 + Mn 3264.0 |
1086.6 ± 1.6 |
100.05 |
1086.1 ± 3.6 |
Ba 2244.0 + Cr 206.0 |
SM-4 |
Hg 1086.0 + Pd 2206.5 |
1085.2 ± 1.8 |
99.93 |
1086.3 ± 3.6 |
Al 1188.0 + Ag 608.0 |
SM-5 |
Hg 1086.0 + Ca 1224.0 |
1086.1 ± 1.1 |
100.01 |
1085.9 ± 5.5 |
Mg 1108.0 + Fe 906.0 |
After validation, the method was successfully applied for the determination of Hg(II) in the wastewater. For this, six wastewater samples (WWS-1 to WWS-6) were collected from our laboratory on different days and Hg(II) was determined using the proposed CKM. The results obtained for different WWS are shown in Table 4. The results obtained by CKM were also validated by AAS and were in excellent agreement with those determined by CKM (Table 3). The maximum error in the proposed CKM with respect to AAS is less than 5%, which is well accepted in the environmental analysis.
Table 4 Determination of Hg(II) in wastewater samples (WWS) under optimized reaction conditions: [Ru(CN)64−] = 5.0 × 10−5 M, [Pz] = 7.5 × 10−4 M, pH = 4.00 ± 0.02, I = 0.05 M (KCl), temp. = 45.0 ± 1.0 °C and its comparison with the (AAS) method
Wastewater sample (WWS) |
[Hg2+] foundb ± SDa by CKM (ng mL−1) |
[Hg2+] foundb ± SDa by AAS (ng mL−1) |
Error in CKM versus AAS (%) |
The ±SD values represent % relative standard deviation of the mean for three determinations. Mean of three determinations. |
WWS-1 |
224.06 ± 2.50 |
228.70 ± 3.26 |
+2.07 |
WWS-2 |
329.64 ± 4.35 |
331.65 ± 2.35 |
+1.44 |
WWS-3 |
244.16 ± 3.84 |
237.45 ± 4.50 |
−2.75 |
WWS-4 |
402.25 ± 4.36 |
408.24 ± 3.45 |
+1.50 |
WWS-5 |
398.17 ± 5.50 |
390.75 ± 3.60 |
−1.86 |
WWS-6 |
389.07 ± 5.50 |
407.08 ± 4.60 |
+4.63 |
4. Conclusions
Though there are many sophisticated methods available for the Hg(II) determination but the present CKM based on the indicator reaction between [Ru(CN)6]4−–Pz offers a number of advantages over them, such as the unanalyzed reaction rate is negligible under specified reaction conditions and only 15 min is required for the Hg(II) determination without using any costly solvents and instruments. In addition, the method can be successfully applied for the determination of Hg(II) in the presence of a number of cations, anions and complexing agents with very good sensitivity. In conclusion, the proposed CKM is quite sensitive, quick, economical and superior to few known methods including CKM.35–38 It can selectively be applied for the Hg(II) determination at micro-level in environmental water samples.
Conflicts of interest
The authors are declare that there is not any conflict of interest including any financial, personal or other relationships with other people or organizations.
Acknowledgements
The authors are thankful to the Head, Department of Chemistry, University of Lucknow, for providing laboratory facility to perform this work. Authors are also thankful to Dr Sanjay Srivastava, Department of Medicine, Division of Cardiology, University of Louisville, KY, USA for providing potassium hexacyanoruthenate(II) as a gift. The author S. Prasad is grateful to The University of the South Pacific for support in various ways.
References
- M. Vandenbossche, M. Jimenez, M. Casetta and M. Traisnel, Remediation of heavy metals by biomolecules: a review, Crit. Rev. Environ. Sci. Technol., 2015, 45, 1644–1704 CrossRef CAS.
- S. Meland, R. Borgstrøm, L. S. Heier, B. O. Rosseland, O. Lindholm and B. Salbu, Chemical and ecological effects of contaminated tunnel wash water runoff to a small Norwegian stream, Sci. Total Environ., 2010, 408, 4107–4117 CrossRef CAS PubMed.
- M. Hamzeh, B. Ouddane, M. Daye and J. Halwani, Trace metal mobilization from surficial sediments of the Seine River Estuary, Water, Air, Soil Pollut., 2014, 225, 1–15 CrossRef CAS.
- J. Zhou, B. Du, L. Shang, Z. Wang, H. Cui, X. Fan and J. Zhou, Mercury fluxes, budgets, and pools in forest ecosystems of China: a review, Crit. Rev. Environ. Sci. Technol., 2020, 50, 1411–1450 CrossRef CAS.
- G. P. Pandey, A. K. Singh, S. Prasad, L. Deshmukh, A. Asthana, S. B. Mathew and M. Yoshida, Kinetic determination of trace amount of mercury(II) in environmental samples, Microchem. J., 2016, 128, 55–61 CrossRef CAS.
- M. A. Shreadah, S. A. A. Ghani, A. A. E. S. Taha, A. E. M. M. Ahmed and H. B. I. Hawash, Mercury and methyl mercury in sediments of Northern Lakes-Egypt, J. Environ. Prot., 2012, 3, 254–261 CrossRef CAS.
- Y. Xu, Y. Fan, L. Zhang, Q. Wang, H. Fub and Y. She, A novel enhanced fluorescence method based on multifunctional carbon dots for specific detection of Hg2+ in complex samples, Spectrochim. Acta, Part A, 2019, 220, 117109 CrossRef CAS PubMed.
- R. Z. Zhang and W. Chen, Nitrogen-doped carbon quantum dots: facile synthesis and applicationas a “turn-off” fluorescent probe for detection of Hg2+ ions, Biosens. Bioelectron., 2014, 55, 83–90 CrossRef CAS PubMed.
- J. C. Wasserman, L. de O. Silva, G. C. de Pontes and E. de P. Lima, Mercury contamination in the sludge of drinking water treatment plants dumping into a reservoir in Rio de Janeiro, Brazil, Environ. Sci. Pollut. Res., 2018, 35, 28713–28724 CrossRef PubMed.
- S. Jensen and A. Jernelov, Biological methylation of mercury in aquatic organisms, Nature, 1969, 223, 753–754 CrossRef CAS PubMed.
- B. Duval, A. Gredilla, S. F.-O. de Vallejuelo, E. Tessier, D. Amouroux and A. de Diego, A simple determination of trace mercury concentrations in natural waters using dispersive Micro-Solid phase extraction preconcentration based on functionalized graphene nanosheets, Microchem. J., 2020, 154, 104549 CrossRef CAS.
- T. W. Clarkson, L. Magos and G. J. Myers, The toxicology of mercury—current exposures and clinical manifestations, N. Engl. J. Med., 2003, 349, 1731–1737 CrossRef CAS PubMed.
- L. Schmidt, J. A. L. Figueroa, P. D. Vecchia, F. A. Duarte, P. A. Mello, J. A. Caruso and E. M. M. Flores, Bioavailability of Hg and Se from seafood after culinary treatments, Microchem. J., 2018, 139, 363–371 CrossRef CAS.
- J. T. Salonen, K. Seppanen, K. Nyyssonen, H. Korpela, J. Kauhanen, M. Kantola, J. Tuomilehto, H. Esterbauer, F. Tatzber and R. Salonen, Intake of mercury from fish, lipid peroxidation, and the risk of myocardial infarction and coronary, cardiovascular, and any death in eastern Finnish men, Circulation, 1995, 91, 645–655 CrossRef CAS PubMed.
- J. Risher and R. DeWoskin, Toxicological profile for mercury, U.S. Department of Health and Human Services, Public Health Service, Agency for Toxic Substances and Disease Registry, Atlanta, Georgia, USA, 1999 Search PubMed.
- K. H. Kim, E. Kabir and S. A. Jahan, A review on the distribution of Hg in the environment and its human health impacts, J. Hazard. Mater., 2016, 306, 376–385 CrossRef CAS PubMed.
- H. Chen, W. Hu and C. M. Li, Colorimetric detection of mercury(II) based on
2,2-bipyridyl induced quasi-linear aggregation of gold nanoparticles, Sens. Actuators, B, 2015, 215, 421–427 CrossRef CAS.
- S. Prasad, Kinetic determination of mercury(II) at trace level from its catalytic effect on a ligand substitution process, J. Anal. Chem., 2005, 60, 581–588 CrossRef CAS.
- C. H. Park, Y. Eom, L. J. Lee and T. G. Lee, Simple and accessible analytical methods for the determination of mercury in soil and coal samples, Chemosphere, 2013, 93, 9–13 CrossRef CAS PubMed.
- P. Liang, J. Yu, E. Yang and Y. Mo, Determination of mercury in food and water samples by displacement-dispersive liquid-liquid microextraction coupled with graphite furnace atomic absorption spectrometry, Food Analytical Methods, 2015, 8, 236–242 CrossRef.
- R. Koplik, I. Klimesova, K. Malisova and O. Mestek, Determination of mercury species in foodstuffs using LC-ICP-MS: the applicability and limitations of the method, Czech, J. Food Sci., 2014, 32, 249–259 CAS.
- O. Garcia-Beltran, N. Mena, T. A. Berrios, E. A. Castro, K. Cassels, M. T. Nunez and M. E. Aliaga, A selective fluorescent probe for the detection of mercury(II) in aqueous media and its applications in living cells, Tetrahedron Lett., 2012, 53, 6598–6601 CrossRef CAS.
- A. Sabouri and S. Nouroozi, Direct determination of total mercury in urine samples using flow injection catalytic cold vapor atomic absorption spectrometry (FI-CCV-AAS), RSC Adv., 2016, 6, 80354–80360 RSC.
- S. R. Segade and J. F. Tyson, Determination of inorganic mercury and total mercury in biological and environmental samples by flow injection-cold vapor-atomic absorption spectrometry using sodium borohydride as the sole reducing agent, Spectrochim. Acta, Part B, 2003, 58, 797–807 CrossRef.
- N. Pourreza and K. Ghanemi, Determination of mercury in water and fish samples by cold vapor atomic absorption spectrometry after solid phase extraction on agar modified with 2-mercaptobenzimidazole, J. Hazard. Mater., 2009, 161, 982–987 CrossRef CAS PubMed.
- B. Passariello, M. Barbaro, S. Quaresima, A. Casciello and A. Marabini, Determination of mercury by inductively coupled plasma—mass spectrometry, Microchem. J., 1996, 54, 348–354 CrossRef CAS PubMed.
- Y. Takaku, Determination of mercury in environmental water by inductively coupled plasma mass spectrometry, J. Environ. Chem., 2010, 20, 45–49 CrossRef CAS.
- W. Zhang, X. Yang, Y. Ma, H. Zhu and S. Wang, Analyst Continuous flow electrolytic cold vapor generation atomic fluorescence spectrometric determination of Hg in water samples, Microchem. J., 2011, 97, 201–206 CrossRef CAS.
- D. Sanchez-Rodas, W. T. Corns, B. Chen and P. B. Stockwell, Atomic fluorescence spectrometry: a suitable detection technique in speciation studies for arsenic, selenium, antimony and mercury, J. Anal. At. Spectrom., 2010, 25, 933–946 RSC.
- L. H. Marcolino-Junior, B. C. Janegitz, B. C. Lourencao and O. Fatibello-Filho, Anodic stripping voltammetric determination of mercury in water using a chitosan-modified carbon paste electrode, Anal. Lett., 2007, 40, 3119–3128 CrossRef CAS.
- M. Vanitha, N. Balasubramanian, I. M. Joni and C. Panatarani, Detection of mercury ions using L-cysteine modified electrodes by anodic stripping voltammetric method, AIP Conf. Proc., 2018, 1927, 030001 CrossRef.
- A. Giacomino, A. R. Redda, S. Squadrone, M. Rizzi, M. C. Abete, C. L. Gioiae, R. Toniolo, O. Abollino and M. Malandrino, Anodic stripping voltammetry with gold electrodes as an alternative method for the routine determination of mercury in fish. Comparison with spectroscopic approaches, Food Chem., 2017, 221, 737–745 CrossRef CAS PubMed.
- T. Hashempur, M. K. Rofouei and A. R. Khorrami, Speciation analysis of mercury contaminants in water samples by RP-HPLC after solid phase extraction on modified C18 extraction disks with 1,3-bis(2-cyanobenzene)triazene, Microchem. J., 2008, 89, 131–136 CrossRef CAS.
- S. S. De Souza, A. D. Campiglia and F. Barbosa, A simple method for methylmercury, inorganic mercury and ethylmercury determination in plasma samples by high performance liquid chromatography-cold-vapor-inductively coupled plasma mass spectrometry, Anal. Chim. Acta, 2013, 761, 11–17 CrossRef CAS PubMed.
- R. M. Naik, A. Agarwal and S. Prasad, Determination of trace amounts of mercury(II) in water samples using a novel kinetic catalytic ligand substitution reaction of hexacyanoruthenate(II), Spectrochim. Acta, Part A, 2009, 74, 887–891 CrossRef PubMed.
- R. M. Naik, P. K. Singh, R. Rastogi, R. Singh and A. Agarwal, Kinetic-catalytic and spectrophotometric determination of Hg(II) using its catalytic effect on ligand substitution reaction between hexacyanoferrate(II) and pyrazine, Ann. Chim., 2007, 97, 1169–1179 CrossRef CAS.
- R. M. Naik and J. Sarkar, Kinetic determination of mercury(II) at trace level from its catalytic effect on ligand substitution reaction between hexacyanoferrate(II) and nitroso-R-salt, Indian J. Chem. Technol., 2005, 12, 563–566 CAS.
- S. Prasad, Catalytic abstraction of cyanide in hexacyanoferrate(II) by mercury(II) in the presence of α-nitroso-β-naphthol as indicator reaction for determination of mercury(II) by kinetic method, Anal. Lett., 2004, 37, 2851–2867 CrossRef CAS.
- A. H. Al-Bagawi, W. Ahmad, Z. M. Saigl, H. Alwael, E. A. Al-Harbi and M. S. El-Shahawi, A simple and low cost dual-wavelength β-correction spectrophotometric determination and speciation of mercury(II) in water using chromogenic reagent 4-(2-thiazolylazo) resorcinol, Spectrochim. Acta, Part A, 2017, 187, 174–180 CrossRef CAS PubMed.
- S. V. Babu and K. H. Reddy, Direct spectrophotometric determination of mercury(II) using 2-acetylpyridine thiosemicarbazone in environmental samples, Indian J. Adv. Chem. Sci., 2012, 1, 65–72 Search PubMed.
- A. Agarwal, S. Prasad and R. M. Naik, Inhibitory kinetic spectrophotometric method for the quantitative estimation of d-penicillamine at micro levels, Microchem. J., 2016, 128, 181–186 CrossRef CAS.
- R. Verma, A. Asthana, A. K. Singh and S. Prasad, An arginine functionalized magnetic nano-sorbent for simultaneous removal of three metal ions from water samples, RSC Adv., 2017, 7, 51079–51089 RSC.
- R. M. Naik, A. K. Verma, A. Agarwal and A. Asthana, Kinetic and mechanistic study of the mercury(II)-catalyzed substitution of cyanide in hexacyanoruthenate(II) by pyrazine, Transition Met. Chem., 2009, 34, 209–215 CrossRef CAS.
- CRC Handbook of Chemistry and Physics, Chemical Rubber Publishing Company, USA, 60th edn, 1979, p. D148 Search PubMed.
- N. Tanaka, Kyoto-Fu Eisci Kogai, Kenkyusho Nemp., 1977, 22, 121 (Chem. Abstr., 1979, 90, 114401v) CAS.
- A. O. Tirler, I. Pearsson, T. S. Hofer and B. M. Rode, Is the hexacyanoferrate(II) anion stable in aqueous solution? A combined theoretical and experimental study, Inorg. Chem., 2015, 54, 10335–10341 CrossRef CAS PubMed.
|
This journal is © The Royal Society of Chemistry 2020 |
Click here to see how this site uses Cookies. View our privacy policy here.