DOI:
10.1039/D0RA03431B
(Paper)
RSC Adv., 2020,
10, 20827-20836
Kappa-carrageenan oligosaccharides retard the progression of protein and lipid oxidation in mackerel (Scomber japonicus) fillets during frozen storage†
Received
17th April 2020
, Accepted 24th May 2020
First published on 2nd June 2020
Abstract
The antioxidative effects of κ-carrageenan oligosaccharides (CO) on the stability of proteins and lipids in mackerel fillets were determined during frozen storage. Electronic nose analysis indicated that CO treatments maintained the stability of the overall volatile flavor profiles in frozen mackerel. Protein oxidation analysis suggested that the incorporation of CO significantly retarded the rapid decrease of Ca2+-ATPase activity and active sulphydryl (A-SH) contents while also effectively inhibiting the increases in carbonyl content and surface hydrophobicity of myofibrillar proteins (MPs) compared to the control treatments. Lipid stability results showed that the peroxide values (PVs), conjugated diene (CD) content, anisidine values (AVs), and thiobarbituric acid index (TBA-i) values of the extracted lipids were also clearly reduced by CO treatments during frozen storage. Fatty acid composition determinations further confirmed that the permeated CO molecules stabilized the polyunsaturated C22:6n3 (DHA) in the lipids, most likely due to their efficient free radical scavenging activities.
1. Introduction
Mackerel (Scomber japonicus) are one of the most important pelagic fish resources throughout the world. Mackerel are abundantly harvested in China, India, Southern Europe, and Iceland. High-fat mackerel provides a good source of easily digestible proteins, polyunsaturated fatty acids (e.g., EPA, DHA), minerals (e.g., calcium, selenium), and vitamins (e.g., vitamin A and D). Despite these obvious benefits, the use of fresh mackerel is greatly limited due to its highly perishable nature during postmortem aging. Therefore, the fish are commercially processed refrigerated or frozen as value-added products before transport and/or export. Frozen storage as the primary processing method is used to preserve the nutritional value of mackerel products; this effectively suppresses microbial growth and biochemical processes. However, decreased quality of mackerel products still occurs by cold-induced denaturation and oxidation of proteins and lipids during prolonged frozen storage.1 These events cause serious variation in physicochemical properties of muscle proteins (water-holding capacity, Ca2+-ATPase activity, and carbonyl content) and lipids (peroxide value and thiobarbituric acid index), resulting in deteriorated elasticity, juice, flavor, and nutritional value.2
Protein and lipid oxidation/degradation in mackerel muscle are the most important reasons for these deteriorative changes during long-term frozen storage. Protein and lipid oxidation are unavoidable processes, especially in high-fat fish species. Protein and lipid oxidation produce many kinds of compounds, most of which are susceptible to reacting with other molecules in the muscle tissues.3 During long-term frozen storage, the growth and recrystallization of ice crystals, reactive oxygen species (ROS) attack, and dehydration and solute concentration all promote the denaturation and oxidation of myofibrillar proteins (MPs), which apparently results in decreases of MPs and Ca2+-ATPase activity, formation of carbonyls and dityrosine, and increases in surface hydrophobicity.4 Similar to protein oxidation, lipid oxidation can also be induced by molecular oxygen and free radicals in muscle, leading to the generation of many oxidation products, e.g., malondialdehyde and cholesterol oxidation products.5 The progress of the lipid oxidation is commonly evaluated by the generation of the primary (e.g., conjugated diene, peroxidation) and secondary (anisidine, thiobarbituric acid-reactive substances) oxidation products. Moreover, the generated compounds from the lipid oxidation in turn modify the amino acids of MPs, thus affecting the solubility, fragmentation, and/or aggregation of proteins.6
Kappa-carrageenan oligosaccharides are degraded from carrageenan by acidic/enzymatic hydrolysis; they provide important physiological benefits in many biological processes. These processes include antiangiogenic effects, immune regulation, antitumor activity, and protein stabilization.7,8 More importantly, carrageenan oligosaccharides and their derivatives have reported good antioxidative activities in both organismal and in vitro systems; these effects are connected with the sulfate and reducing sugar content and polymerization degree.9 According to previous studies, the oligosaccharides can interact with ice crystals, similar to antifreeze proteins, and inhibit the growth and recrystallization of ice crystals in frozen muscle tissues during storage.10 Moreover, the oligosaccharides can also combine with other proteins such as myosin in porcine muscle.11 In our previous study, the cryoprotective effects (focused on MPs) of carrageenan oligosaccharides were evaluated in whole shrimp muscle tissues (low-fat content). The results indicated good control of drip loss, maintenance of muscle texture and color, and protection from the physical damage caused by ice crystals.7,12 Despite the findings of these previous studies, little information is available concerning the antioxidative effects of carrageenan oligosaccharides on the protein and lipid oxidation in the high-fat mackerel (Scomber japonicus) during frozen storage. This study therefore explored the potential applications of carrageenan oligosaccharides incorporated in frozen mackerel. Our focus was the relationship between oligosaccharide molecules and myofibrillar proteins/lipids in mackerel muscle during long-term frozen storage.
2. Materials and methods
2.1 Chemicals
Carrageenan oligosaccharides (CO; [C6H9O8SNa]n, n = 2–10; κ type; >99% purity) were purchased from Seebio Biotech Co., Ltd. (Shanghai, China). Maleate, tris(hydroxymethyl)aminomethane (Tris), adenosine 5′-triphosphate, guanidine, methanol, dichloromethane, sodium phosphate, and trichloroacetic acid (TCA) were obtained from Chemical Reagents Shanghai Co., Ltd. (Shanghai, China). Ferric thiocyanate, 2-nitro-5-thiosulphpbenzoate (NTSB), 2,4-dinitrophenyl hydrazine (DNPH), p-anisidine, acetic acid, 1-anilinonaphthalene-8-sul-fonic acid (ANS), and 2-thiobarbituric acid were procured from Shandong Tuopu Bio-engineering CO., Ltd., Zhaoyuan, China. All chemicals and reagents used in this study were of analytical grade.
2.2 Mackerel samples and treatments
Commercial frozen mackerel (Scomber japonicus) were kindly provided by Zhejiang Xingye Co., Ltd. (Zhoushan, China). Frozen mackerel samples (from fish caught in the East China Sea measuring 28–34 cm in length and 260–320 g in weight) were placed in a cooler filled with ice and transported to the lab within 20 min from the time of packaging. Upon arrival, the individuals were washed thoroughly with pre-cooled tap water to remove the debris. Next, the mackerel samples were defrosted, beheaded, filleted, and deboned manually, but not skinned off. The obtained fillets (with an average weight of 90 ± 9 g and length of 18 ± 4 cm) were rapidly cleaned using cold water. Subsequently, the drained fillet samples were further soaked (4 °C) in either distilled water (control), 1.5% (w/v) CO, 3.0% (w/v) CO, or 4.5% (w/v) CO solution (four soaking batches). After soaking at 4 °C for 3 h, mackerel fillets were drained at 4 °C for 3 min, and then kept at −30 °C for 3 h. Finally, the frozen samples were transferred to a refrigerator at −18 °C and maintained for 100 days (d). At 20 day intervals (0, 20, 40, 60, 80, and 100 d), the fillet samples were removed, thawed at 4 °C for 3 h, and then analyzed.
2.3 Volatile flavor analysis
Volatile profiles of mackerel samples were measured by using a PEN3 electronic nose instrument equipped with ten metal oxide sensors (Airense, Schwerin, Germany) (details are presented in Table S1†). Briefly, thawed fillets were homogenized (8000 rpm, 4 °C) in a pre-cooled homogenizer (model MB 550, Shinetek Instruments Research Institute, Beijing, China) for 30 s. Next, 5 g of minced muscle were filled into a 30 mL glass vial and then sealed by an exclusive PTFE/silicone cap. The sealed vials were maintained at 25 °C for 20 min to allow equilibration before headspace sampling. Subsequently, volatile samples were absorbed by the electronic nose using the following conditions: room temperature, 25 °C; flushing time, 80 s; self-calibration zero time, 10 s; chamber flow, 300 mL min−1; measurement time, 80 s; sample interval, 1 s; and automatic dilution program. Each signal curve recorded by the electronic nose shows the changes of a sensor resistivity ratio (G/G0 represents the resistivities of a sensor when exposed to a sample gas and zero gas) of conductance during 80 s of measurement. Six replicates of each sample were analyzed, and the average of the values (each sample, 10 data points from 40 s to 50 s) was used for the statistical analysis.
2.4 Protein oxidation analysis
2.4.1 Myofibrillar Ca2+-ATPase activity. Myofibrillar proteins (MPs) extracted from mackerel muscle were prepared using the method of Zhang, et al.7 The samples were minced and homogenized in Tris–maleate buffer. After centrifugation, the resulting sediment was re-suspended, homogenized, and extracted again. The supernatant was considered as the extracted MPs. Subsequently, the extracted MPs were added to 0.50 mol L−1 Tris–maleate buffer (pH 7.0) containing 0.10 mol L−1 CaCl2 and 20 mmol L−1 adenosine 5′-triphosphate. The final concentrations of MPs in the buffer ranged from 1.0 to 2.0 mg mL−1 protein. After incubation at 30 °C for 5 min, 30% (w/v) of TCA was added to the mixture to terminate the reaction. This was followed by centrifugation at 5000 × g for 5 min. Ca2+-ATPase activity of MPs was calculated as micromoles of inorganic phosphate released in the supernatant per milligram of protein per minute (μmol Pi per mg per min).
2.4.2 Active sulfhydryl (A-SH) content analysis. Active sulphydryl (A-SH) content of MPs was analyzed using the NTSB assay described by Zhang, et al.13 Briefly, the extracted MPs were reacted with freshly prepared NTSB solution at 40 °C for 30 min. The absorbance of the mixture was measured at 412 nm. A-SH content was calculated using the molar absorption coefficient of 13.9 mmol−1 cm−1.
2.4.3 Carbonyl content analysis. Carbonyl content of MPs was evaluated by reaction with DNPH as previously described Zareian, et al.14 MP samples were added to 0.2% (w/v) DNPH solution (containing 2 mol L−1 HCl) and incubated in darkness at 25 °C for 60 min with vortexing every 15 min. The resulting reaction mixture was then terminated with 20% (w/v) TCA solution. The centrifuged sediment (12
000 × g, 3 min) was washed and dissolved in 20 mmol L−1 sodium phosphate buffer (containing 6 mol L−1 guanidine). Finally, the absorbance at 365 nm was determined, and the carbonyl content (nmol carbonyl per mg protein) was calculated with the molar absorption coefficient of 22 mmol−1 cm−1.
2.4.4 Surface hydrophobicity analysis. Surface hydrophobicity of MPs was analyzed using ANS as the fluorescence probe by the method of Zhang, et al.7 with some modifications. MP solution was mixed with 8 mmol L−1 ANS in 0.1 mol L−1 phosphate buffer (pH 7, containing 0.6 mol L−1 NaCl) and stirred at room temperature for 10 min. The relative intensity of the resulting solution was measured with a fluorescence spectrophotometer (model F9700, INESA Analytical Instrument Co., Ltd., Shanghai, China). The emission and excitation wavelengths were designated as 485 nm and 375 nm, respectively. The initial slope (SoANS) of the fluorescence intensity versus MP concentration was recorded from the linear regression analysis.
2.5 Lipid oxidation analysis
Lipids in mackerel fillets were extracted by a binary mixture of methanol and dichloromethane (v/v, 1
:
1) according to the procedure of Otero, et al.15 The obtained lipid extractions were immediately used for the following analyses.
2.5.1 Peroxide value (PV) and conjugated diene (CD) content analysis. PVs in extracted mackerel lipids were measured by peroxide reduction with ferric thiocyanate as described by Chapman & McKay.16 The calculated results are expressed as meq. active oxygen per kg lipids.CD formations were assessed in extracted mackerel lipids according to the method of Pérez-Andrés, et al.17 CD contents are expressed as absorption coefficients (AC): CD = A233 × V/W, where A233 is the absorbance at 233 nm of the lipid extraction, V (mL) represents the volume of sample, and W (mg) represents the mass of lipids in the extraction.
2.5.2 Anisidine value (AV) and thiobarbituric acid index (TBA-i) analysis. AVs of lipid samples were determined according to the procedure of Akcan, Estévez, & Serdaroğlu.18 Extracted lipids were dissolved in n-hexane, and the absorbance was recorded at 350 nm by a spectrophotometer (A1). The obtained solution was further mixed with p-anisidine in acetic acid. The absorbance of the mixture containing p-anisidine was measured at 350 nm (A2). AVs were calculated as AV = 25 × (1.2 × A2 − A1)/sample weight.TBA-i was analyzed in the samples by the method of Senphan & Benjakul.19 Lipid extract was mixed with 2-thiobarbituric acid solution. The mixture was further heated in a water bath. The absorbance of the cooled solution was measured at 538 nm in a spectrophotometer. TBA-i values were calculated as mg malonaldehyde (MDA) per kg mackerel muscle.
2.5.3 Fatty acid composition analysis. Firstly, extracted lipids dissolved in hexane were trans-esterified to fatty acid methyl esters (FAMEs). Then, the obtained FAMEs were determined by a gas chromatograph (model GC-2014C, Shimadzu (Shanghai) Co., LTD., Shanghai, China) equipped with a flame ionization detector (model FID-2014) and a Sh-Famewax™ capillary column (30 × 0.32 mm, 0.25 μm film thickness; Shimadzu (Shanghai) Co., LTD., Shanghai, China). Chromatography was performed by using the following parameters: carrier gas, helium at a flow of 1.0 mL min−1; injector temperature, 225 °C; detector temperature 285 °C; split ratio, 1
:
40; and injected sample-volume, 1 μL (50 mg mL−1). Fatty acid compositions in mackerel fillets were identified with a mixed FAMEs standard (containing 37 analytes, Dikma Technologies Inc., Beijing, China).
2.6 Data analysis
The results obtained from three replications (n = 3) of the measurements were subjected to statistical analysis using SPSS package 13.0 for Windows (SPSS Inc., Chicago, IL, USA). Comparison of treatment means was performed by Duncan's test, and a P-value of less than 0.05 indicated that the means differed significantly.
3. Results and discussions
3.1 Volatile flavor
Electronic nose analysis was performed to provide a comprehensive evaluation of the overall volatile flavor profiles in mackerel fillets during frozen storage (Fig. 1; quantified data are showed in Tables S2 and S3†). In the case of the control mackerel (Fig. 1A), clear differences were observed between the fresh and cold-stored fillets on days 60, 80, and 100 in relation to several flavor and odor compounds formed and/or changed during frozen storage. The fingerprint outlines of volatile flavors in mackerel were altered slightly during the initial 0–40 days, implying similar volatile ingredients and good quality of these mackerel samples. The initial total volatile basic nitrogen (TVBN) content of mackerel fillets was determined to be 2.8 ± 0.3 mg/100 g. The low TVBN content in fresh samples was also agreed with the obtained volatile flavor results.20 Moreover, the signals of sensor 2 (W5S, broad range sensitivity, sensitive to nitrogen oxides) were significantly (P < 0.05) higher than those of other sensors; nitrogen oxides are considered as the initial volatile compounds in the natural mackerel (0–40 days). However, during the following period (60–100 days), the signal values of sensor 2 decreased, while those values of other sensors (except sensor 6, W1S, sensitive to methane) all increased significantly (P < 0.05). These results demonstrated that ammonia (sensor 3, W3C), hydrogen sulfide (sensors 4 and 7, W6S and W1W), alcohol (sensor 8, W2S), sulfur volatiles (sensor 9, W2W), and methane compounds (sensor 10, W3S) were gradually formed and accumulated in mackerel fillets, likely leading to unpleasant odor (decreased flavor quality) at the end of storage. It was reported previously that the sulfur volatiles in iced tilapia fillets also increased with the storage time, accompanied by the development of off-flavor.21 These increased volatile flavor profiles in frozen mackerel might be related to denaturation and oxidation of muscle proteins and may also be associated with lipid oxidation mainly induced by cold stress and long-term storage.22
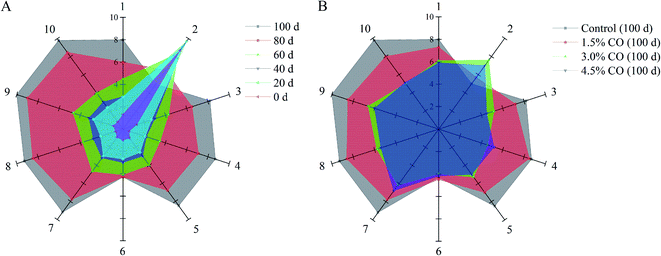 |
| Fig. 1 Radar charts of volatile flavor collected from the control mackerel (A) during 0–100 days of frozen storage. Radar charts of volatile flavor collected from the control, 1.5% CO soaked-, 3.0% CO soaked-, and 4.5% CO soaked-samples (B) after 100 days of frozen storage. | |
For CO-soaking treatments (Fig. 1B), all three mackerel samples maintained significantly higher response signals on sensor 2 and also showed lower response signals on the other sensors (P < 0.05) compared with the control after 120 days of storage. The development of the off-flavor in the frozen mackerel was significantly (P < 0.05) reduced when CO soaking was performed in the pre-treatment. Significantly, 4.5% and 3.0% CO treatments maintained the stability of the overall volatile flavor profiles in frozen mackerel; the profiles had similar patterns to that of the fresh sample. Additionally, the fingerprint outlines of the two samples were nearly completely overlapping, suggesting relatively similar volatile ingredients (no significant difference) in the treated mackerel after 100 days of frozen storage. The obtained results clearly indicated the benefits of CO treatment in maintaining the natural flavor characteristics and retarding the off-flavor development of mackerel samples during frozen storage. In the previous studies, carrageenan oligosaccharides provided useful antioxidant activity in vitro and scavenging activity towards DPPH·, O2−˙, and OH˙ radicals and suppressed the oxidation of myofibrillar proteins in frozen shrimp during long periods of frozen storage.7 The results of the current study suggest that the effective antioxidant activity of CO might play an important role in the maintenance of the initial (natural) volatile compounds in frozen-stored mackerel.
3.2 Myofibrillar Ca2+-ATPase activity
Ca2+-ATPase activity is an important indicator used for evaluating the structural integrity and denaturation of myofibril proteins (MPs) in frozen mackerel during frozen storage. In this study, changes of myofibrillar Ca2+-ATPase activity extracted from mackerel muscle with different treatments were determined (Fig. 2A). In control samples, Ca2+-ATPase activity exhibited a significant (P < 0.05) decrease to 25.0% of the initial value over 100 d of frozen storage. This could have resulted from the changes in the tertiary structure (denaturation) of the myosin globular head or from the protein–protein interactions occurring in the mackerel muscle during frozen storage.23 However, the activities of Ca2+-ATPase detected in 1.5%, 3.0%, and 4.5% CO treated samples were maintained at 41.6%, 52.7%, and 58.3% of the initial values when the storage time was 100 d. These values were all significantly (P < 0.05) higher than in the control treatment. Moreover, the activity preservation effects were not significant (P > 0.05) between the 3.0% and 4.5% CO treatments, although the effects were significantly higher than in the 1.5% CO and control treatments (P < 0.05). Here, the obtained results clearly indicated that CO showed better cryoprotection for the MPs extracted from mackerel subjected to a long period of frozen storage.
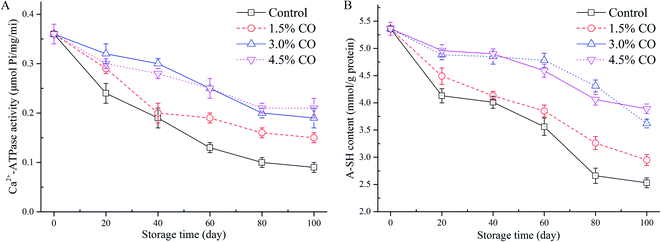 |
| Fig. 2 Myofibrillar Ca2+-ATPase activity (A) and A-SH content (B) in mackerel fillets, including the untreated control and treatments with 1.5% CO, 3.0% CO, and 4.5% CO during 100 days of frozen storage. | |
In general, the protein denaturation occurring in muscle during frozen storage can be evaluated by Ca2+-ATPase activity, total and active sulphydryl content, and protein solubility. Proteins can be denatured when hydrophobic interactions, hydrogen bonds, and hydration of polar residues are destroyed during processing and/or storage. These events lead to decreased quality parameters. In the current work, the improved Ca2+-ATPase activity observed in the CO treatments might be attributed to the hydrophilic hydroxyls located on the CO chains. The hydroxyls combine water molecules by non-covalent forces (water constraining effects), reduce the formation and growth of ice crystals in the muscle tissues, and circumvent the destruction of the conformational structure and interactions of MPs.12 Gao, Hou, & Zeng24 also indicated that saccharide treatments, such as alginate oligosaccharides, trehalose, and sodium lactate, could effectively mitigate the decrease of myofibrillar Ca2+-ATPase activity. Our findings are consistent with the results of these previous studies.
3.3 A-SH content
The sulphydryl group is the most reactive functional group in MPs; the groups include those buried in the proteins and those exposed on the surface that are susceptible to be attacked by the reactive oxygen species. They are easily oxidized during freezing and frozen storage, leading to the formation of disulphide bonds and decreases in A-SH levels.24 The lower A-SH content in muscle usually indicates a higher degree of MP oxidation. The initial A-SH content detected in the mackerel muscle was 5.36 mmol L−1 (Fig. 2B). After 100 d of storage, values in the control samples decreased to 2.53 mmol L−1, suggesting that protein denaturation (oxidation) had occurred in the muscle. Incorporation of 1.5% CO (2.95 mmol L−1), 3.0% CO (3.62 mmol L−1), and 4.5% CO (3.89 mmol L−1) all had significantly (P < 0.05) beneficial effects on the amount of A-SH in the frozen mackerel fillets. The variation of A-SH content in the 3.0% and 4.5% CO treatments showed similar trends during the entire duration of frozen storage; these treatments had higher sulphydryl values than the control and 1.5% CO treatments.
The loss of sulphydryl content was related to the formation of disulfide bonds between or within polypeptides, leading to protein oxidation and aggregation.24 Several studies have reported that CO molecules exhibited good capacities of radical scavenging and Fe2+-chelating activity in vitro and had positive effects on stability of MPs in shrimp (low-lipid species) muscle during frozen storage.7,25 In the current study, the permeated saccharides could effectively interact with the MPs in muscle tissues. The structural and functional stability of MPs was greatly improved by the hydroxyl groups in the saccharide molecules that formed hydrogen bonds with polar amino acids on the surface of the MPs.26 Additionally, oxidation of sulphydryl groups on the MPs (especially on myosin globular head) is also closely associated with a decrease of Ca2+-ATPase activity during processing and storage.27 The current results confirmed that the decrease of Ca2+-ATPase activity and the oxidation of hydroxyl groups were both mitigated by the antioxidant activity contributed by the incorporated CO molecules.
3.4 Carbonyl content
Carbonyl content determination was performed to evaluate the development of protein oxidation during frozen storage (Fig. 3A). From the results, the initial carbonyl content was 2.53 nmol mg−1 MPs in the fresh mackerel (0 d). The control sample value was increased significantly (P < 0.05) to 4.68 nmol mg−1 MPs after 100 d of storage, indicating that oxidation occurred during long-term cold conditions. Similar trends (increases) were also observed for the samples corresponding to the 1.5% CO, 3.0% CO, and 4.5% CO treatments. The carbonyl contents in these three treatments increased to 4.16 nmol mg−1, 3.51 nmol mg−1, and 3.45 nmol mg−1, respectively, which were significantly (P < 0.05) lower than control values at the end of frozen storage. The inhibition of the increase of carbonyl content was not significant (P > 0.05) between the 3.0% CO and 4.5% CO treatments, while the effects were significantly higher (lower carbonyl content) than in the 1.5% CO treatment (P < 0.05).
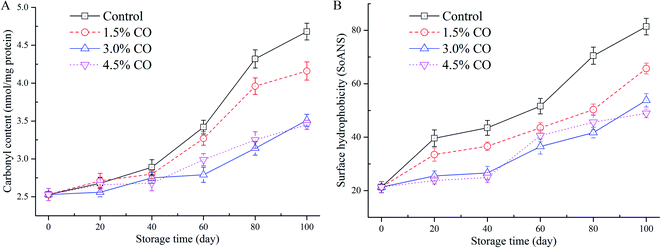 |
| Fig. 3 Carbonyl content (A) and surface hydrophobicity (B) in mackerel fillets, including the untreated control and treatments with 1.5% CO, 3.0% CO, and 4.5% CO during 100 days of frozen storage. | |
Carbonyl groups are mainly transformed from –NH and/or –NH2 groups on amino acid side chains when muscle proteins are exposed to oxidizing conditions. During frozen storage, carbonyl formation is an irreversible process in proteins. Oxidation leads to the fragmentation, aggregation, and loss of solubility and functionality of MPs.28 The polar amino acids on the protein surface exposed to the water phase would be more accessible to the potent oxidation promoters and are more susceptible to oxidative reactions to form the carbonyl derivatives. Additionally, the lipid oxidation in muscle could also catalyze the protein oxidation (carbonyl formation), and these processes could develop simultaneously. In the current study, the permeation of CO into the muscle tissues greatly inhibited carbonyl formation compared to the control treatments. The CO molecules could scavenge the free radicals and hydroperoxides in the muscle and reduce attacks on the polar amino acid residues. The hydrogen binding ability of CO with MPs likely decreases the exposure of hydrophilic (polar) amino acids (e.g., lysine, arginine, serine, and threonine residues) to the oxidation conditions.29
3.5 Surface hydrophobicity
The changes in surface hydrophobicity of MPs in frozen mackerel during storage are shown in Fig. 3B. At the beginning of storage, the mackerel muscle had a low SoANS value, indicating that a small amount of fluorescent probe was bound to the exposed hydrophobic groups with only slight denaturation in the MPs. In the control samples, SoANS values of the MPs increased rapidly (P < 0.05) during 100 d of frozen storage. The cold stress stretched and unfolded the MPs, resulting in loose and destabilized conformational structures with increased surface hydrophobicity.30 However, different changes in SoANS values as affected by the permeated CO molecules up to 100 d were detected, and their values were significantly (P < 0.05) lower than those of control samples. However, no significant difference was detected between these two CO soaking treatments (P > 0.05).
Surface hydrophobicity is closely correlated with the structure stabilization of MPs and is generally used to reflect subtle changes from the original protein structure. Compared to the control, higher concentrations of CO treatment showed the slow increases in SoANS values in MPs. This obtained result suggested less conformational changes with the exposure of hydrophobic portions occurring during frozen storage. These findings demonstrate that CO suppressed the progress of denaturation of MPs, presumably due to its good antioxidant activity and the potential interactions between saccharides and MPs formed by hydrophilic–hydrophilic interactions.7 Wu & Lin30 also suggested that hydrogen bonding between proteins and the hydroxyl groups of saccharides presumably is the mechanism that provides cryoprotection. Furthermore, Nikoo, Benjakul, & Rahmanifarah28 found that some saccharides and/or polyols, including sucrose/sorbitol, lactitol, and polydextrose, could stabilize the protein structure by intensifying the hydrophobic interactions inside the protein molecules. In the current study, incorporation of CO molecules to the muscle tissues significantly improved the stability of MPs during frozen storage. The interactions of CO molecules with the MPs led to reduced exposure of buried hydrophobic residues on the protein surface and thus improved conformational stability and structural integrity of the frozen proteins (reduced protein oxidation denaturation).31 Taken together, based on A-SH content, carbonyl content, and hydrophobicity data obtained, the CO treatments provided good cryoprotective and antioxidant effects.
3.6 PV and CD
The primary indicators of lipid oxidation, including PV and CD content in mackerel fillets, were measured during 0–100 days of frozen storage (Table 1). In the control samples at 0 d, PV (0.36 meq. active oxygen per kg lipids) and CD (absorption coefficient; 0.19) values represented a satisfactory lipid quality of the mackerel. These values are similar to those reported by Quitral, et al.32 During the following 100 d of frozen storage, the values of PV and CD in control samples significantly (P < 0.05) increased to 7.02 meq. active oxygen per kg and 4.78, respectively. This showed that primary lipid oxidation developed in the mackerel muscle during frozen storage. The values of PV and CD in 3.0% CO and 4.5% CO treatments were maintained at 4.18 meq. active oxygen per kg and 2.88 and 3.76 meq. active oxygen per kg and 3.11, respectively, which were significantly (P < 0.05) lower than the respective values for samples corresponding to the control and 1.5% CO (5.25 meq. active oxygen per kg and 3.75) treatments. Here, the overall changes in the 3.0% CO and 4.5% batches showed no significant differences (P > 0.05) during the whole storage period and exhibited better effects on the maintenance of the oxidative lipid stability than the control treatment.
Table 1 Effect of CO soaking treatments on the primary lipid oxidation (PV and CD values) development in frozen mackerel fillets during 0–100 days of storagea
Lipid oxidation index |
Storage time (days) |
Soaking conditions |
Control |
1.5% CO |
3.0% CO |
4.5% CO |
Data represent the means ± standard deviation of 3 replicates. The means with different lowercase letters in the same column for the PV and CD, respectively, were significantly different at P < 0.05; the means with different uppercase letters in the same row were significantly different at P < 0.05. |
PV (meq. active oxygen per kg lipids) |
0 |
0.36 ± 0.06aA |
0.36 ± 0.06aA |
0.36 ± 0.06aA |
0.36 ± 0.06aA |
20 |
1.88 ± 0.11bB |
1.65 ± 0.18bB |
0.69 ± 0.11bA |
0.62 ± 0.12bA |
40 |
2.03 ± 0.18bB |
1.89 ± 0.20bB |
1.12 ± 0.18cA |
1.36 ± 0.18cA |
60 |
4.11 ± 0.23cC |
2.88 ± 0.23cB |
1.99 ± 0.21dA |
2.31 ± 0.15dA |
80 |
5.23 ± 0.18dC |
4.45 ± 0.19dB |
3.26 ± 0.25eA |
3.28 ± 0.21eA |
100 |
7.02 ± 0.19eC |
5.25 ± 0.20eB |
4.18 ± 0.22fA |
3.76 ± 0.16fA |
CD (absorption coefficient) |
0 |
0.19 ± 0.04aA |
0.19 ± 0.04aA |
0.19 ± 0.04aA |
0.19 ± 0.04aA |
20 |
0.86 ± 0.09bC |
0.52 ± 0.11bB |
0.26 ± 0.09aA |
0.35 ± 0.08aAB |
40 |
1.76 ± 0.16cC |
1.36 ± 0.17cB |
0.92 ± 0.13bA |
0.89 ± 0.10bA |
60 |
2.09 ± 0.21cC |
1.50 ± 0.13cB |
1.08 ± 0.14bA |
1.19 ± 0.14bA |
80 |
2.89 ± 0.19dC |
2.19 ± 0.20dB |
1.59 ± 0.13cA |
1.68 ± 0.10cA |
100 |
4.78 ± 0.16eC |
3.75 ± 0.19eB |
2.88 ± 0.20dA |
3.11 ± 0.16dA |
PVs provide the initial evidence of rancidity stage in fish products. The maximum allowable levels of PV are recognized as being less than 5 meq. active oxygen per kg lipids according to the standard values defined by the Codex Alimentarius Commission.33 This primary lipid oxidation could be initiated by several conditions, including the production of free radicals and active oxygen. In the current study, all four mackerel fillet treatments had PVs (initial-phase oxidation) below the maximum limitations, suggesting that the stored samples showed low levels of rancidity. Significantly, the incorporated CO molecules successfully retarded primary lipid oxidation (PV and CD content) in frozen samples during storage. Herein, CO antioxidants incorporated into the muscle tissues likely acted as scavengers against free radicals and active oxygen and/or by reducing iron, resulting in the decreased development of the initial oxidation. In addition, after the soaking treatments, CO molecules on the surface of mackerel fillets might provide barriers to oxygen; this was likely associated with the inhibition of the primary lipid oxidation during frozen storage.
3.7 AV and TBA-i
Secondary lipid oxidation development in mackerel fillets was measured by the evaluation of the AV and TBA-i values during frozen storage (Table 2). The TBA-i values of mackerel lipids increased in a similar manner to those of AVs during whole frozen storage. In the control samples, the AV and TBA-i values were significantly (P < 0.05) increased from the initial 0.89 and 0.53 mg MDA per kg to 1.75 and 2.43 mg MDA per kg, respectively, indicating that relative lipid oxidation occurred during 100 days of frozen storage. However, no significant differences (P > 0.05) between the control and treated batches were observed for these secondary oxidation values during the initial 0–40 days of storage. Moreover, 3.0% CO and 4.5% CO treatments retarded the progress of the lipid oxidation, maintaining the AV and TBA-i values at 1.19 and 1.28 mg MDA per kg and 1.23 and 1.21 mg MDA per kg, respectively, after 100 days of frozen storage. These values were significantly (P < 0.05) lower than those obtained in the control samples. Additionally, the higher concentration of CO treatments (3.0% and 4.5%) showed comparatively better antioxidant activities than the lower concentration (1.5%) treatment, especially for the inhibition effect on the TBA-i values of mackerel lipids during frozen storage.
Table 2 Effect of CO soaking treatments on the secondary lipid oxidation (AV and TBA-i) development in frozen mackerel fillets during 0–100 days of storagea
Lipid oxidation index |
Storage time (days) |
Soaking conditions |
Control |
1.5% CO |
3.0% CO |
4.5% CO |
Data represent the means ± standard deviation of 3 replicates. The means with different lowercase letters in the same column for the AV and TBA-i, respectively, were significantly different at P < 0.05; the means with different uppercase letters in the same row were significantly different at P < 0.05. |
AV |
0 |
0.89 ± 0.13aA |
0.89 ± 0.13aA |
0.89 ± 0.13aA |
0.89 ± 0.13aA |
20 |
0.96 ± 0.20aA |
0.81 ± 0.15aA |
0.92 ± 0.18aA |
0.81 ± 0.11aA |
40 |
0.92 ± 0.19aA |
0.95 ± 0.07aA |
0.97 ± 0.17aA |
0.96 ± 0.09aA |
60 |
1.12 ± 0.11abA |
1.01 ± 0.09abA |
0.89 ± 0.11aA |
1.00 ± 0.06aA |
80 |
1.30 ± 0.09bB |
1.15 ± 0.07bcA |
1.08 ± 0.07abA |
0.98 ± 0.13aA |
100 |
1.75 ± 0.16cB |
1.35 ± 0.13cA |
1.19 ± 0.06bA |
1.23 ± 0.07bA |
TBA-i (mg MDA per kg muscle) |
0 |
0.53 ± 0.04aA |
0.53 ± 0.04aA |
0.53 ± 0.04aA |
0.53 ± 0.04aA |
20 |
0.61 ± 0.07aA |
0.59 ± 0.06abA |
0.50 ± 0.07aA |
0.59 ± 0.03aA |
40 |
0.87 ± 0.03bA |
0.71 ± 0.08bA |
0.62 ± 0.10aA |
0.68 ± 0.07aA |
60 |
0.93 ± 0.08bB |
0.80 ± 0.09bAB |
0.69 ± 0.09aA |
0.71 ± 0.05aA |
80 |
1.66 ± 0.10cC |
1.36 ± 0.10cB |
1.05 ± 0.06bA |
1.09 ± 0.07bA |
100 |
2.43 ± 0.05dC |
1.79 ± 0.11dB |
1.28 ± 0.06bA |
1.21 ± 0.09bA |
In the current study, all the mackerel samples had relatively low AV (0.89–1.75) and TBA-i (0.53–2.43 mg MDA per kg) values during the entire duration of storage, although the values increased with storage time. According to the literature, similar increases with a range of 1.5–3.0 mg MDA per kg in TBA-i values were observed in frozen minced mackerel during storage at −20 °C,34 consistent with the current findings. Moreover, it was suggested that TBA-i values of 1–2 mg MDA per kg are the limits beyond which the fish muscle would produce an unpleasant volatile flavor.17 Thus, the obtained TBA-i values in the current study agreed with the volatile flavor results assayed by the electronic nose, indicating that lipid oxidation most likely promoted the changes of volatile flavor of frozen mackerel fillets when the storage time increased. For CO soaking treatments, the radical scavenging activity of CO7 might be mainly responsible for the inhibitory effects on the lipid oxidation (AV and TBA-i) in frozen mackerel. Additionally, the oxidation of lipids in muscle is undoubtedly interlinked with the protein oxidation through the chain reaction of radicals, and these processes affect each other during processing, transport, and storage. Hence, the retarded lipid oxidation by the CO soaking treatments in turn inhibited the development of MP oxidation, resulting in the maintenance of Ca2+-ATPase activity and A-SH content and decreases in carbonyls and surface hydrophobicity in the mackerel during frozen storage.
3.8 Fatty acid composition
The fatty acid (FA) compositions of extracted lipids from mackerel fillets were measured after 100 days of frozen storage and were compared with the fresh samples (Table 3). For the fresh and treated mackerel fillets, the proportion of polyunsaturated FAs in the extracted lipids constituted approximately 58% of the total fatty acids, where the monounsaturated and saturated FAs comprised 20.2–24.3% and 17.7–21.8% of the total fatty acids, respectively. Among these, C20:5n3 (EPA), C22:6n3 (DHA), and C18:1n9c (oleic acid) covered a large proportion of the total FAs in the mackerel lipids. These results were in accordance with the previous findings obtained in Indian mackerel (Rastrelliger kanagurta)35 and minced horse mackerel (Trachurus trachurus).6
Table 3 Effect of CO soaking treatments on the fatty acid composition (mg kg−1 muscle) in frozen mackerel fillets after 100 d of storage, compared with that of the fresh samples (0 d)a
Fatty acid composition (mg/100 g) |
Fresh sample (0 d) |
Control (100 d) |
1.5% CO (100 d) |
3.0% CO (100 d) |
4.5% CO (100 d) |
Data represent the means ± standard deviation of 3 replicates; the means with different uppercase letters in the same row were significantly different at P < 0.05. C8:0, C10:0, C11:0, C12:0, C13:0, C14:1, C15:1, C18:1n9t, C18:2n6t, C21:0, C20:0, C22:1n9, C22:2, C22:1n9, C23:0, and C24:0 were detected while not showed in this table, the concentrations of which were less than 10 mg/100 g. 1511.86. |
C14:0 |
75.87 ± 4.63A |
88.25 ± 3.36B |
78.99 ± 4.11A |
74.69 ± 3.26A |
77.15 ± 2.99A |
C15:0 |
20.99 ± 1.88A |
19.89 ± 1.29A |
21.22 ± 1.62A |
20.68 ± 1.99A |
19.39 ± 2.01A |
C16:0 |
58.67 ± 3.01A |
67.28 ± 2.59B |
66.21 ± 1.99B |
60.12 ± 2.16A |
61.25 ± 3.01A |
C18:0 |
117.18 ± 6.12A |
129.11 ± 6.33B |
124.36 ± 5.55AB |
119.69 ± 4.69A |
121.22 ± 5.01A |
C20:0 |
11.05 ± 0.89A |
12.02 ± 1.12A |
11.56 ± 0.98A |
10.99 ± 1.13A |
12.15 ± 1.01A |
ΣSaturated |
283.76 ± 16.58A |
316.55 ± 13.68B |
301.34 ± 14.19A |
286.17 ± 13.64A |
291.16 ± 14.11A |
C16:1 |
93.94 ± 5.87A |
94.33 ± 3.26A |
92.11 ± 4.11A |
91.52 ± 2.89A |
92.64 ± 4.95A |
C17:1 |
12.17 ± 0.96A |
11.55 ± 1.11A |
11.46 ± 1.32A |
12.65 ± 0.99A |
12.34 ± 2.01A |
C18:1n9c |
106.88 ± 6.78A |
105.62 ± 5.25A |
104.89 ± 6.11A |
107.58 ± 5.49A |
107.93 ± 4.83A |
C20:1 |
63.92 ± 3.58A |
63.88 ± 2.69A |
64.25 ± 3.01A |
64.56 ± 2.22A |
65.12 ± 1.19A |
C24:1 |
29.26 ± 0.49A |
30.33 ± 1.15A |
28.85 ± 0.99A |
28.99 ± 1.48A |
30.18 ± 2.01A |
ΣMonounsaturated |
306.17 ± 17.42A |
305.71 ± 13.02A |
301.56 ± 15.32A |
305.3 ± 13.11A |
308.21 ± 14.09A |
C18:2n6c |
60.44 ± 3.22A |
58.11 ± 2.89A |
61.22 ± 3.11A |
62.33 ± 1.22A |
59.86 ± 2.35A |
C18:3n3 |
48.89 ± 0.81A |
49.69 ± 1.28A |
47.29 ± 1.11A |
50.03 ± 2.01A |
48.71 ± 1.36A |
C20:3n6 |
12.58 ± 0.48A |
13.25 ± 1.00A |
12.88 ± 1.18A |
13.06 ± 1.13A |
12.64 ± 0.97A |
C20:4n6 |
29.18 ± 1.33A |
28.66 ± 2.02A |
30.11 ± 1.59A |
28.60 ± 1.98A |
29.27 ± 1.23A |
C20:5n3 (EPA) |
203.48 ± 9.47B |
180.05 ± 7.71A |
188.12 ± 5.32AB |
195.09 ± 6.00B |
192.66 ± 4.85B |
C22:6n3 (DHA) |
567.36 ± 7.01A |
538.25 ± 7.05A |
544.69 ± 5.22A |
536.01 ± 5.65A |
538.10 ± 5.00A |
ΣPolyunsaturated |
921.93 ± 19.08C |
868.01 ± 17.88A |
884.31 ± 17.36AB |
895.12 ± 17.64B |
899.24 ± 15.76B |
During frozen storage, the overall variation of FA composition was relatively similar in all the mackerel samples. Notably, the amount of C22:6n3 (DHA) and Σpolyunsaturated FAs were decreased in the control samples, while the amounts of C14:0, C16:0, C18:0, and Σsaturated FAs were clearly increased after 100 days of storage. Mbarki, Sadok, & Barkallah36 also suggested that the polyunsaturated FAs underwent oxidation to a greater extent than the saturated FAs, which was confirmed by the decrease in polyunsaturated FAs and increase in saturated FAs during chilled storage. The current results were consistent with this report. Moreover, the CO soaking treatments showed good maintenance capacity on the polyunsaturated C22:6n3 (DHA) in the lipids, most likely due to its good free radical scavenging activity resulting in enhanced stability of the unsaturated lipid during the frozen storage.
According to the previous studies, the polyunsaturated FAs are especially prone to lipid oxidation and/or degradation. This is a rather complex process of unsaturated FAs reacting with molecular oxygen through free radical chains to form fatty hydroperoxides as well as nonvolatile and volatile hydroperoxide.37 It has been suggested that the reaction rate of oxidation commonly tends to increase with the storage time and temperature. A similar study by Secci, et al.38 suggested that significant increases of saturated FAs together with significant decreases of docosapentaenoic acid (C22:5n3) were found in Atlantic horse mackerel (Trachurus trachurus) attributed to the storage effect. In contrast, a previous report indicated that no significant changes occurred in the FA compositions in variously treated mackerel (Scomber scombrus, L.) during refrigerated storage.15 These conflicting results might be due to the different fish species and lipid compositions, the presence of prooxidants and/or inhibitors, and storage time or temperature. In any case, the oxidation development of the saturated, monounsaturated, and polyunsaturated FAs in high-fat fish during chilling and frozen storage needs further study.
4. Conclusions
We incorporated κ-carrageenan oligosaccharides (CO) into mackerel (Scomber japonicus) fillets. The oxidation of myofibrillar proteins (MPs) and lipids in mackerel fillets were determined during 100 days of frozen storage. Compared to the control, CO soaking treatment conferred significant protection to the MPs, including maintenance of CA2+-ATPase activity and A-SH content and inhibition of carbonyl content and surface hydrophobicity. CO also retarded the increase in peroxide values (PVs), conjugated diene (CD) content, anisidine values (AVs), and thiobarbituric acid index (TBA-i) values and improved the stability of polyunsaturated fatty acids during frozen storage. The antioxidant and cryoprotection effects of CO on the stability of MPs and lipids in frozen mackerel could be used to extend the shelf-life and maintain the quality of frozen high-fat fish products.
Conflicts of interest
All authors have no conflicts of interest to declare.
Acknowledgements
This study was funded by the National Natural Science Foundation of China (No. 31871871), the Zhejiang Natural Science Foundation of China (No. LY18C200008), and the Key Scientific and Technological Innovation Project of Wenzhou (No. ZS2019001 and ZD202003). We thank LetPub (https://www.letpub.com) for its linguistic assistance during the preparation of this manuscript.
References
- J. Cropotova, R. Mozuraityte, I. B. Standal, S. Ojha, T. Rustad and B. Tiwari, Influence of high-pressure processing on quality attributes of haddock and mackerel minces during frozen storage, and fishcakes prepared thereof, Innovative Food Sci. Emerging Technol., 2020, 59, 102236 CrossRef CAS.
- A. Arulkumar, S. Paramasivam and J. M. Miranda, Combined effect of icing medium and red alga Gracilaria verrucosa on shelf life extension of Indian mackerel (Rastrelliger kanagurta), Food Bioprocess Technol., 2018, 11, 1911–1922 CrossRef CAS.
- M. Trigo, A. Rodríguez, G. Dovale, A. Pastén, A. Vega-Gálvez and S. P. Aubourg, The effect of glazing based on saponin-free quinoa (Chenopodium quinoa) extract on the lipid quality of frozen fatty fish, LWT–Food Sci. Technol., 2018, 98, 231–236 CrossRef CAS.
- C. Leygonie, T. J. Britz and L. C. Hoffman, Impact of freezing and thawing on the quality of meat: Review, Meat Sci., 2012, 91(2), 93–98 CrossRef PubMed.
- A. Soyer, B. Özalp, U. Dalmış and V. Bilgin, Effects of freezing temperature and duration of frozen storage on lipid and protein oxidation in chicken meat, Food Chem., 2010, 120(4), 1025–1030 CrossRef CAS.
- K. H. S. Farvin, H. D. Grejsen and C. Jacobsen, Potato peel extract as a natural antioxidant in chilled storage of minced horse mackerel (Trachurus trachurus): Effect on lipid and protein oxidation, Food Chem., 2012, 131, 843–851 CrossRef.
- B. Zhang, C. D. Fang, G. J. Hao and Y. Y. Zhang, Effect of kappa-carrageenan oligosaccharides on myofibrillar protein oxidation in peeled shrimp (Litopenaeus vannamei) during long-term frozen storage, Food Chem., 2018, 245, 254–261 CrossRef CAS PubMed.
- Y. J. Sun, X. Y. Cui, M. M. Duan, C. Q. Ai, S. Song and X. F. Chen, In vitro fermentation of κ-carrageenan oligosaccharides by human gut microbiota and its inflammatory effect on HT29 cells, J. Funct. Foods, 2019, 59, 80–91 CrossRef CAS.
- Y. J. Sun, B. Y. Yang, Y. M. Wu, Y. Liu, Y. Gu and H. Zhang, Structural characterization and antioxidant activities of kappa-carrageenan oligosaccharides degraded by different methods, Food Chem., 2015, 178, 311–318 CrossRef CAS PubMed.
- A. Kamińska-Dwórznicka, P. Skrzypczak and E. Gondek, Modification of kappa carrageenan by β-galactosidase as a new method to inhibit recrystallization of ice, Food Hydrocolloids, 2016, 61, 31–35 CrossRef.
- T. Pan, H. Guo, Y. Li, J. Song and F. Ren, The effects of calcium chloride on the gel properties of porcine myosin-κ-carrageenan mixtures, Food Hydrocolloids, 2017, 63, 467–477 CrossRef CAS.
- B. Zhang, H. C. Yang, H. Tang, G. J. Hao, Y. Y. Zhang and S. G. Deng, Insights into cryoprotective roles of carrageenan oligosaccharides in peeled whiteleg shrimp (Litopenaeus vannamei) during frozen storage, J. Agric. Food Chem., 2017, 65(8), 1792–1801 CrossRef CAS PubMed.
- T. Zhang, Y. Xue, Z. J. Li, Y. M. Wang, W. Yang and C. H. Xue, Effects of ozone on the removal of geosmin and the physicochemical properties of fish meat from bighead carp (Hypophthalmichthys nobilis), Innovative Food Sci. Emerging Technol., 2016, 34, 16–23 CrossRef CAS.
- M. Zareian, T. Tybussek, P. Silcock, P. Bremer, J. Beauchamp and N. Böhner, Interrelationship among myoglobin forms, lipid oxidation and protein carbonyls in minced pork packaged under modified atmosphere, Food Packaging and Shelf Life, 2019, 20, 100311 CrossRef.
- L. Otero, M. Pérez-Mateos, F. Holgado, G. Márquez-Ruiz and M. E. López-Caballero, Hyperbaric cold storage: pressure as an effective tool for extending the shelf-life of refrigerated mackerel (Scomber scombrus, L.), Innovative Food Sci. Emerging Technol., 2019, 51, 41–50 CrossRef.
- R. Chapman and J. McKay, The estimation of peroxides in fats and oils by the ferric thiocyanate method, J. Am. Oil Chem. Soc., 1949, 26, 360–363 CrossRef CAS.
- J. M. Pérez-Andrés, M. de Alba, S. M. Harrison, N. P. Brunton, P. J. Brunton and B. K. Tiwari, Effects of cold atmospheric plasma on mackerel lipid and protein oxidation during storage, LWT–Food Sci. Technol., 2020, 118, 108697 CrossRef.
- T. Akcan, M. Estévez and M. Serdaroğlu, Antioxidant protection of cooked meatballs during frozen storage by whey protein edible films with phytochemicals from Laurus nobilis L. and Salvia officinalis, LWT–Food Sci. Technol., 2017, 77, 323–331 CrossRef CAS.
- T. Senphan and S. Benjakul, Compositions and yield of lipids extracted from hepatopancreas of Pacific white shrimp (Litopenaeus vannamei) as affected by prior autolysis, Food Chem., 2012, 134(2), 829–835 CrossRef CAS PubMed.
- B. Zhang, L. K. Ma, S. G. Deng, C. Xie and X. H. Qiu, Shelf-life of pacific white shrimp (Litopenaeus vannamei) as affected by weakly acidic electrolyzed water ice-glazing and modified atmosphere packaging, Food Control, 2015, 51, 114–121 CrossRef CAS.
- Z. C. Wang, Y. Q. Lu, Y. Z. Yan, T. Nisar, Z. X. Fang, N. Xia, Y. Guo and D. W. Chen, Effective inhibition and simplified detection of lipid oxidation in tilapia (Oreochromis niloticus) fillets during ice storage, Aquaculture, 2019, 511, 634183 CrossRef CAS.
- I. Albertos, I. Jaime, A. M. Diez, L. González-Arnáiz and D. Rico, Carob seed peel as natural antioxidant in minced and refrigerated (4 °C) Atlantic horse mackerel (Trachurus trachurus), LWT–Food Sci. Technol., 2015, 64(2), 650–656 CrossRef CAS.
- L. F. Jiang and S. J. Wu, Pullulan suppresses the denaturation of myofibrillar protein of grass carp (Ctenopharyngodon idella) during frozen storage, Int. J. Biol. Macromol., 2018, 112, 1171–1174 CrossRef CAS PubMed.
- W. H. Gao, R. Hou and X. A. Zeng, Synergistic effects of ultrasound and soluble soybean polysaccharide on frozen surimi from grass carp, J. Food Eng., 2019, 241, 1–8 CrossRef.
- H. Yuan, W. Zhang, X. Li, X. Lu, N. Li, X. Gao and J. Song, Preparation and in vitro antioxidant activity of kappa-carrageenan oligosaccharides and their oversulfated, acetylated, and phosphorylated derivatives, Carbohydr. Res., 2005, 340(4), 685–692 CrossRef CAS PubMed.
- B. Zhang, H. X. Wu, H. C. Yang, X. W. Xiang, H. B. Li and S. G. Deng, Cryoprotective roles of trehalose and alginate oligosaccharides during frozen storage of peeled shrimp (Litopenaeus vannamei), Food Chem., 2017, 228, 257–264 CrossRef CAS PubMed.
- Y. Shao, L. Wang, C. S. Chen, G. Q. Xiong, Y. Q. Hu, Y. Qiao, W. J. Wu, X. Li, J. Wang, L. Liao and A. Ding, Antioxidant capacity of fermented soybeans and their protective effect on protein oxidation in largemouth bass (Micropterus salmoides) during repeated freezing–thawing (FT) treatments, LWT–Food Sci. Technol., 2018, 91, 213–221 CrossRef CAS.
- M. Nikoo, S. Benjakul and K. Rahmanifarah, Hydrolysates from marine sources as cryoprotective substances in seafoods and seafood products, Trends Food Sci. Technol., 2016, 57, 40–51 CrossRef CAS.
- B. Zhang, G. J. Hao, H. J. Cao, H. Tang, Y. Y. Zhang and S. G. Deng, The cryoprotectant effect of xylooligosaccharides on denaturation of peeled shrimp (Litopenaeus vannamei) protein during frozen storage, Food Hydrocolloids, 2018, 77, 228–237 CrossRef CAS.
- Y. B. Wu and K. W. Lin, Influences of xylooligosaccharides on the quality of Chinese-style meatball (kung-wan), Meat Sci., 2011, 88, 575–579 CrossRef CAS PubMed.
- F. G. C. Ekezie, J. H. Cheng and D. W. Sun, Effects of atmospheric pressure plasma jet on the conformation and physicochemical properties of myofibrillar proteins from king prawn (Litopenaeus vannamei), Food Chem., 2019, 276, 147–156 CrossRef CAS PubMed.
- V. Quitral, M. L. Donoso, J. Ortiz, M. V. Herrera, H. Araya and S. P. Aubourg, Chemical changes during the
chilled storage of Chilean jack mackerel (Trachurus murphyi): effect of a plant-extract icing system, LWT–Food Sci. Technol., 2009, 42, 1450–1454 CrossRef CAS.
- Codex Alimentarius Commision, Standard for Fish Oils. CODEX STAN, 329, Rome, Italy, 2017 Search PubMed.
- I. Sánchez-Alonso, A. Jiménez-Escrig, F. Saura-Calixto and A. J. Borderías, Effect of grape antioxidant dietary fibre on the prevention of lipid oxidation in minced fish: evaluation by different methodologies, Food Chem., 2007, 101, 372–378 CrossRef.
- F. Sahena, I. S. M. Zaidul, S. Jinap, A. M. Yazid, A. Khatib and N. A. N. Norulaini, Fatty acid compositions of fish oil extracted from different parts of Indian mackerel (Rastrelliger kanagurta) using various techniques of supercritical CO2 extraction, Food Chem., 2010, 120(3), 879–885 CrossRef CAS.
- R. Mbarki, S. Sadok and I. Barkallah, Quality changes of the Mediterranean horse mackerel (Trachurus mediterraneus) during chilled storage: the effect of low-dose gamma irradiation, Radiat. Phys. Chem., 2009, 78, 288–292 CrossRef CAS.
- I. Medina, M. J. González, J. Iglesias and N. D. Hedges, Effect of hydroxycinnamic acids on lipid oxidation and protein changes as well as water holding capacity in frozen minced horse mackerel white muscle, Food Chem., 2009, 114, 881–888 CrossRef CAS.
- G. Secci, M. Borgogno, S. Mancini, G. Paci and G. Parisi, Mechanical separation process for the value enhancement of Atlantic horse mackerel (Trachurus trachurus), a discard fish, Innovative Food Sci. Emerging Technol., 2017, 39, 13–18 CrossRef CAS.
Footnote |
† Electronic supplementary information (ESI) available. See DOI: 10.1039/d0ra03431b |
|
This journal is © The Royal Society of Chemistry 2020 |