DOI:
10.1039/D0RA03375H
(Paper)
RSC Adv., 2020,
10, 25529-25539
A multi-technique study of altered granitic rock from the Krunkelbach Valley uranium deposit, Southern Germany†
Received
15th April 2020
, Accepted 11th June 2020
First published on 6th July 2020
Abstract
Herein, a multi-technique study was performed to reveal the elemental speciation and microphase composition in altered granitic rock collected from the Krunkelbach Valley uranium (U) deposit area near an abandoned U mine, Black Forest, Southern Germany. The former Krunkelbach U mine with 1–2 km surrounding area represents a unique natural analogue site with the rich accumulation of secondary U minerals suitable for radionuclide migration studies from a spent nuclear fuel (SNF) repository. Based on a micro-technique analysis using several synchrotron-based techniques such as X-ray fluorescence analysis, X-ray absorption spectroscopy, powder X-ray diffraction and laboratory-based scanning electron microscopy and Raman spectroscopy, the complex mineral assemblage was identified. While on the surface of granite, heavily altered metazeunerite–metatorbernite (Cu(UO2)2(AsO4)2−x(PO4)x·8H2O) microcrystals were found together with diluted coatings similar to cuprosklodowskite (Cu(UO2)2(SiO3OH)2·6H2O), in the cavities of the rock predominantly well-preserved microcrystals close to metatorbernite (Cu(UO2)2(PO4)2·8H2O) were identified. The Cu(UO2)2(AsO4)2−x(PO4)x·8H2O species exhibit uneven morphology and varies in its elemental composition, depending on the microcrystal part ranging from well-preserved to heavily altered on a scale of ∼200 μm. The microcrystal phase alteration could be presumably attributed to the microcrystal morphology, variations in chemical composition, and geochemical conditions at the site. The occurrence of uranyl-arsenate-phosphate and uranyl-silicate mineralisation on the surface of the same rock indicates the signatures of different geochemical conditions that took place after the oxidative weathering of the primary U- and arsenic (As)-bearing ores. The relevance of uranyl minerals to SNF storage and the potential role of uranyl-arsenate mineral species in the mobilization of U and As into the environment is discussed.
Introduction
Uranium (U) is an important trace element and a contaminant representing a significant environmental hazard after the mining and ore reprocessing activities.1–3 U-containing natural systems, e.g. ore bodies and former mining sites, are often considered as natural analogues for investigations of potential radionuclide release and retardation processes expected in a real spent nuclear fuel (SNF) repository.4 In this context, several mineralogical studies have focused on the alteration and oxidative corrosion processes of a primary U mineral, uraninite (UO2+x), and SNF's components under ambient and extreme conditions.5–7 To assess the potential risks associated with the long-term storage and possible alteration of SNF, actinide- and lanthanide-containing systems have been intensively investigated to draw comparisons with analogue systems in a functional repository.8–10
Depending on the local geology and geochemical conditions, the alteration of uraninite results in the formation of various alteration products. Whilst the richest U mineral families are (oxyhydr)oxides, carbonates and silicates, a smaller number of uranyl minerals are represented by selenates (SeO42−), arsenates (AsO43−) and phosphates (PO43−) occurring under oxidizing conditions.11 Selenium (Se) and arsenic (As) are elements of environmental concern due to their high toxicity.12–16 The formation of uranyl selenates and arsenates is mainly associated with oxidation processes of sulfide (S) minerals and acidification of groundwaters followed by the subsequent release of S, Se, As and other trace elements along with U from associated mineralisation. Thus, U, S, As and traces of Se were identified to be simultaneously present in the ore material from the former Krunkelbach mine in both unaltered and altered ores.17 U and As are often associated together in organic-rich sediments where U occurs mainly as reduced U(IV) species, mine tailings and in abandoned mining sites after underground flooding activities. This causes additional hazards associated with the release of As into water aquifers.18–24 In cases when reduction conditions prevail, such as at the Ruprechtov site in the Czech Republic, As occurs in the form of arsenopyrite (FeAsS) in tertiary sediments forming layered aggregates with secondary uraninite and arsenopyrite.19 Mixed copper uranyl arsenate-phosphate species have been identified in the soils from an abandoned U mine in the UK as a result of many years of the mining activities at the site.18,20 Based on the results of these investigations, As is assumed to control U mobility by the formation of a sparingly soluble metazeunerite solid solution (Ksp = 10−49.2). Indeed uranyl arsenates form compounds that have much lower solubility products compared to other uranyl phases, i.e. U (oxyhydr)oxides with U being often incorporated into Fe (oxyhydr)oxides (Ksp = 10−37–10−44),25 thus limiting U and As release into the environment.18,26 The occurrence of mixed phases with a small amount of phosphate substituting for arsenate was first discussed by Frondel.27 Recent studies of U mineralisation from hydrothermal type U deposits in the Southern UK reported the occurrence of metazeunerite–metatorbernite species with a relatively high P content up to ∼20 at%.20 Mineralogical and chemical properties of such mixed phases, however, are still ill-defined. Therefore, studies of synthetic and natural species from different geological locations are necessary to provide additional information on the degradation properties of these compounds, i.e. phase dissolution, ion-exchange behavior depending on chemical composition and temperature, etc.
Both synchrotron and laboratory methods are extensively used separately and in combination for investigations of structural, redox and degradation properties of U minerals.18,20,23,28–30 Whilst synchrotron methods provide robust and fast analysis with higher sample penetration depth for elemental mapping and speciation as low as few tens of ppm for some heavier metals, e.g. uranium, laboratory tools provide more detailed analysis of the sample's morphology and more detailed speciation.31–33 The use of the combined experimental approach is often preferred due to the intricate U speciation in environmental systems, which helps to develop optimal strategies for contaminated site remediation.34,35 For example, the detailed speciation analysis at U contaminated sites in Ohio, at Oak Ridge and Hanford Site in the USA made it possible to develop effective engineering campaigns for reducing the U content in groundwaters by in situ sorption–precipitation or by utilizing permeable reactive barriers.36–38
Rich secondary U mineralisation represents high environmental significance of the location due to possible degradation of these phases and further migration of contaminants in the environment. In this context, one of the aims of the study is to find the evidence for alteration of one of the potentially hazardous secondary U phases using a combination of several techniques. To achieve this, we utilized a multitude of spectroscopic techniques that are both laboratory- and synchrotron-based. We first describe our characterisation efforts, and then put these into the context of SNF storage in a geological repository. Herein, we demonstrate how a combination of synchrotron and laboratory tools can be effectively utilized for the analysis of the crystalline environmental samples without any complicated sample preparation procedure. As a case study, we performed the elemental and microphase speciation on an altered granite rock collected near an abandoned U mine in Southern Germany. Micro-X-ray fluorescence (μ-XRF) coupled with U L3 edge micro-X-ray absorption near-edge structure (μ-XANES) spectroscopy and micro-powder X-ray diffraction (μ-PXRD) analyses, and laboratory Raman and scanning electron microscopy (SEM) with energy-dispersive X-ray (EDX) spectroscopic techniques used for the investigation of complex microphase U-mineral assemblages are highlighted.
Materials and methods
A. Sample description
For our study, a sample material (∼5 × 5 × 10 mm3) was collected near the Krunkelbach Valley uranium deposit (Fig. 1) in the 1980s during underground mining works and later stored in a mineral collection. It was originally described as a two-mica granite rock, Bärhalde granite, together with quartz (SiO2), U silicates – soddyite ((UO2)2SiO4·2H2O), redox mixed U (oxyhydr)oxide – ianthinite (U4+/5+(UO2)5O7·10H2O) and Fe (oxyhydr)oxide – goethite (α-FeO(OH)), forming a pseudomorph after ianthinite on the surface of a rock. The Krunkelbach U deposit is a hydrothermal vein-type deposit with late Carboniferous formation age of 295 ± 7 Ma and the age of secondary U mineralisation estimated at 300 ± 50 ka.39 The pilot exploration took place in the 1960s by shaft mining and U reserves estimated at 1000 tons of U3O8 at an average grade of 0.7 wt% (see geological map in Fig. 1).22,40 The host rock of the deposit is highly altered down to 240 m, which is due to the continuous interaction of the rock with intruding oxygenated ground waters, causing the formation of secondary U and Fe mineralization.
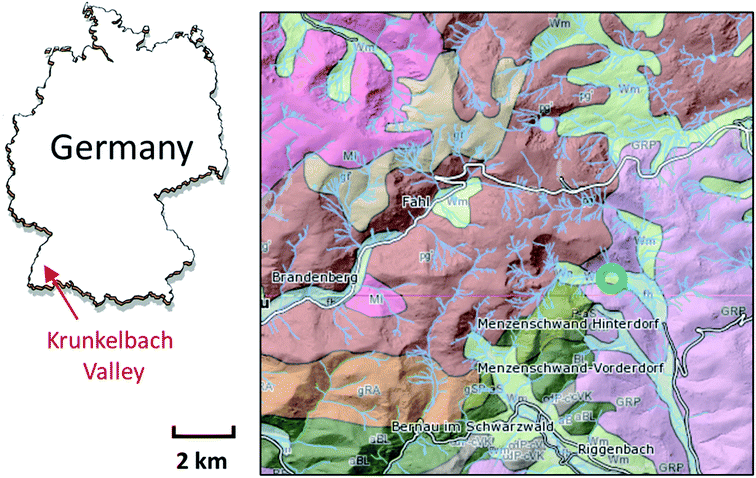 |
| Fig. 1 Location of the Krunkelbach Valley U deposit, Menzenschwand, Baden-Württemberg, Southern Germany (marked with a green circle). A geological relief map of the site is retrieved from the database of the Geological Survey of Germany (https://www.maps.lgrb-bw.de). The Krunkelbach Valley is situated mainly on the flood sediments (fh, light blue) on the border with paragneiss (pg′, brown region), granite plutons (GRP, light-pink region) and Würm-moraine sediments (Wm, light green). | |
The sample was first analysed using a Carl Zeiss STEMI 2000C stereomicroscope to select suitable parts and microcrystals for further investigations. The rock part containing several green microcrystals initially not described was subsequently selected for the bulk μ-XRF and U L3 edge μ-XANES analysis.
B. Synchrotron μ-XRF coupled with U L3 edge μ-XANES spectroscopy
The μ-XRF and U L3 edge μ-XANES measurements were performed at the DUBBLE BM26A beamline of the European Synchrotron Radiation Facility (ESRF).41 The incident energy was selected using a double Si(111) crystal monochromator. Rejection of higher harmonics was achieved with two Pt mirrors at an angle of 2 mrad relative to the incident beam. The dedicated micro-focus platform provided an 8 × 8 μm2 spot size at the sample position. XRF mappings were recorded at 17
177 eV with a 1 s dwell time and 20 μm step size. A 20 μm step size was found optimal based on the preliminary sample analysis using a stereomicroscope. U L3 edge μ-XANES spectra were recorded on nine different spots on the area of 1.5 × 2.5 mm2. Several spectra were recorded at each selected spot for each reference sample: metazeunerite (U–As), metatorbernite (U–P) and cuprosklodowskite (U–Si). All measurements have been performed under ambient conditions.
C. μ-PXRD
The μ-PXRD patterns were collected at the SUL-X beamline of a KIT synchrotron radiation source. Measurements were performed in transmission mode with beam size at the sample position of about 150 × 150 μm2 on grains using a CCD detector (Photonic Science XDI VHR-2 150). The beamline was operated at an energy of 17
000 eV. The D values were calibrated with LaB6 (NIST, 660b) (2 theta values correspond to λ = 0.729684 Å after calibration). Measurements were performed under ambient conditions. Data analysis was performed using the FIT2D program and DIFFRAC.EVA V4.3 (Bruker).42
D. Raman spectroscopy
Raman measurements were conducted at room temperature using a LabRam ARAMIS (Horiba Jobin Yvon) at an excitation wavelength of 532 nm (Nd:YAG). The machine was calibrated using a silicon wafer with the first-order Si line at 520.7 cm−1. For all measurements, an 1800 lines per mm diffraction grating was used with a slit of 100 μm, a hole of 300 μm, and a neutral density filter D 0.3 (50% transparency), respectively.
E. SEM-EDX
The SEM-EDX investigations were performed at Technical University Dresden using a QUANTA 250 FEG (FEI) microscope in LowVac mode combined with an EDX-system QUANTAX 400 (Bruker). The software Esprit 2.1 was used to evaluate the EDX data.
Results
A. Elemental and microphase analysis by μ-XRF and μ-PXRD
Attempts to detect needle-shaped violet ianthinite microcrystals initially described on the rock were not successful. Instead, several tiny platy-shaped vitreous green crystals were identified using an optical microscope on the surface and in the cavities of the sample. The μ-XRF element mapping distinguished regions of different sets of elements with varying signal intensities and areas. U and As were identified in concentrated regions that are associated with Cu (Fig. 2a, right column: EDX data with Cu–U–As RGB mapping). Less intense regions showed the occurrence of Cu, Fe, Pb and W. The latter three showed no correlation with U and As in the intensive signal regions (see left column XRF on Fig. 2a) followed by μ-XANES analysis (Fig. 2b). The occurrence of Cu, U and As agreed with copper-bearing uranyl arsenate, phase corresponding to (meta)zeunerite (Cu(UO2)2(AsO4)2·8−12H2O), one of the most common U mineralisations at the Krunkelbach site.43 The metazeunerite species (see Fig. 3a) were further confirmed by μ-PXRD on green crystals selected from the surface of the rock (Fig. 3b). The presence of W, Bi, Pb, and minor Ba indicated a possible occurrence of two relatively rare U species: uranotungstite ((Fe,Ba,Pb)(UO2)2(WO4)(OH)4·12H2O) and walpurgite ((BiO)4(UO2)(AsO4)2·2H2O). Both species were previously identified in the Krunkelbach and Schneeberg U deposits, respectively.40,44 In the Schneeberg deposit, walpurgite is described to occur together with metazeunerite species. Considering that the XRF measurement is limited to minimum energy of ∼6 keV due to photon self-absorption in air, the detection of some elements, i.e. Na, K, P, Al, Si, etc., is hindered. Hence, the possible presence of another U–As mineral phase – nielsbohrite (K(UO2)3(AsO4)(OH)4·H2O), reported for the Krunkelbach deposit cannot be excluded.45 Other microphases identified on the rock correspond to quartz and goethite (Fig. 3c). No U species were found associated with goethite needles. The energy-dispersive X-ray (EDX) analysis of selected goethite microcrystals did not detect any U assuming its pseudomorphic nature. In previously reported studies, U is found incorporated into a goethite ore as a result of the oxidation and dissolution/reprecipitation events in a U deposit.17 Similarly, the correlation of U with Fe minerals, metatorbernite and akaganeite (β-FeO(OH)), was identified in the soils after intensive U mining activities in the Southern UK.18
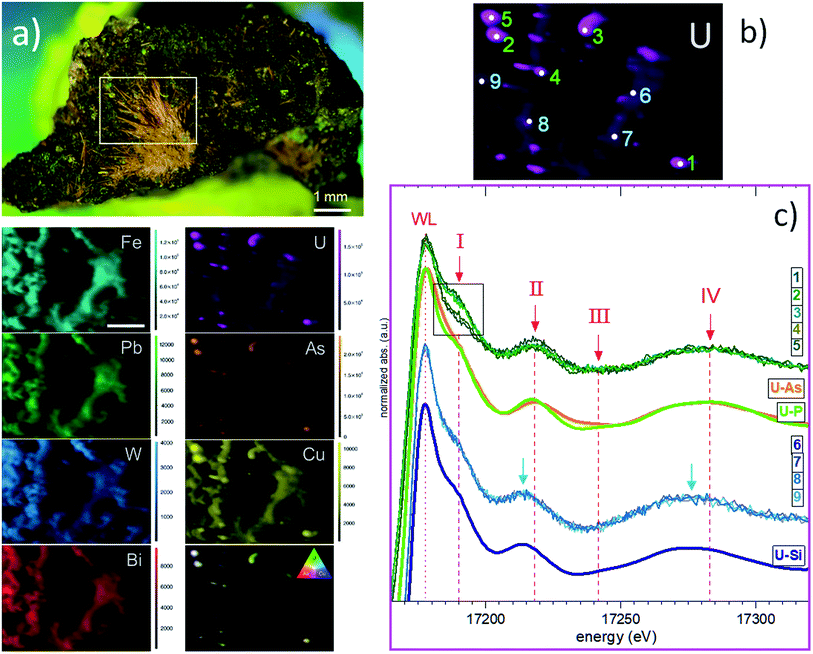 |
| Fig. 2 Photograph of the rock sample with the outlined region (2.5 × 1.5 mm2) selected for μ-XRF/μ-XANES analysis includes fibrous goethite aggregates mixed with green microcrystals, μ-XRF based element mapping (left column: Fe, Pb, W, Bi; right column: U, As, Cu and RGB map for U, As, Cu), scale bar shown at 1 mm (a); U μ-XRF map with nine spots selected for μ-XANES analysis (b); U L3 edge μ-XANES spectra recorded on spots selected from U μ-XRF map, spectra of metatorbernite (U–P), metazeunerite (U–As) and cuprosklodowskite (U–Si) reference samples (c). | |
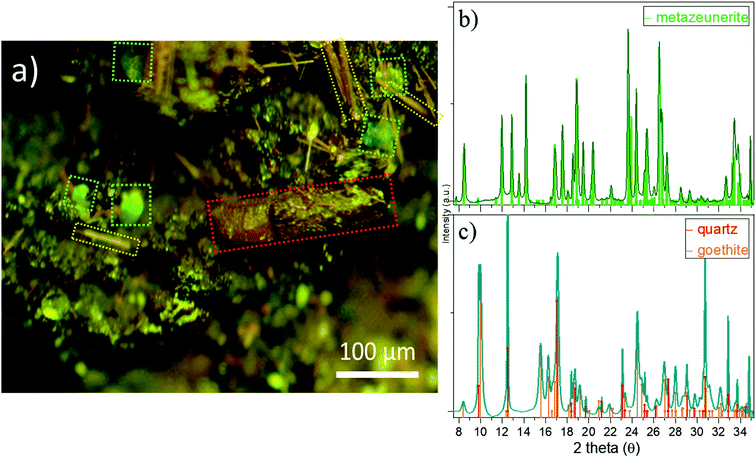 |
| Fig. 3 Microphotograph of the surface: green crystals of metazeunerite, dark-brown quartz, and light-brown, needle-shaped goethite crystals (a); μ-PXRD patterns of green crystals selected from the surface of the granitic rock and database metazeunerite (ICDD – 40148463) (b); and light- and dark-brown crystals correspond to goethite (ICDD – 290713) and quartz (ICDD – 0898935), respectively (c). | |
B. U speciation by U L3 edge μ-XANES
Based on the U μ-XRF map (see Fig. 2b), U L3 edge μ-XANES spectra were recorded on two different regions exhibiting varying U intensity: five selected spots with higher U contents (marked 1–5) and four zones exhibiting less intense U signals (marked 6–9) (Fig. 2c). To compare these sets of spectra, we marked four spectral features as I, II, III and IV within ∼100 eV range, above the white line (WL). The intensity of the WL differs for two sets of spectra and exhibits a higher intensity for the spectra collected from the zones with low U signals (6–9). All spectra recorded for these U zones are identical and fit well with the spectrum of cuprosklodowskite. The latter is described as one of the uranyl silicate minerals occurring in the Krunkelbach deposit.40 The collected spectra show distinct signatures in the energy position for all spectral features (see Fig. S1†). Feature I, referred to multi-scattering paths at the uranyl moiety, exhibits different shapes and intensities depending on the measured zone and exact spot. Feature I reveals a lower intensity in spectra 1 and 4, it is significantly more intensive in spectra 2, 3 and 5, thus providing the first signature of different U speciations for high-intensity U spots (see inlet box in Fig. 2c).46 Features II, III and IV are more sensitive to close local atomic environment around U atoms, i.e. U–O equatorial bonding, and can be used as a relative measure of uranyl–ligand bonding characteristics in studied species. Thus, features II and IV for spectra 6–9 are shifted ∼4–5 eV to the lower energy compared to spectra 1–5 (see Fig. 2c). The similarity of spectra 1–5 with the reference spectra of metazeunerite and metatorbernite and agreement with the XRF analysis results show that the analysed species are related to these two references. Both metazeunerite and metatorbernite species have been previously described in the Krunkelbach U mine, with metazeunerite being the most common secondary U mineral in the area.43 Metazeunerite and metatorbernite are isostructural minerals with minor differences in crystallographic parameters. This example demonstrates that μ-XANES is a powerful tool, which allows for the fingerprinting analysis in cases when the properly defined references can be used (see theoretically modeled spectra on Fig. S2†).
Additionally, the whole rock sample has been analysed by U L3 edge HERFD-XANES spectroscopy (see ESI† for technique description).47 The technique, in general, provides much better resolved spectral features, allowing for significantly more detailed analysis of U redox state especially for environmentally relevant systems, where U can be stabilized as a mixture of two or even three redox states, namely, U(IV), U(V) and U(VI), and more detailed structural characterization.48–50 The collected spectra were recorded with a relatively large beam size, ∼100 × 400 μm2, and likely HERFD probes simultaneously several U phases (Fig. S4†). However, our data show the capacity of HERFD for future investigations, especially in cases when HERFD can be recorded with an X-ray beam size of 1–5 μm. It is important to mention that the detection limit for U, and As and other heavy metals, depends on the synchrotron source and beamline. While systems with few hundred ppm U can be analysed at most of the synchrotrons, a sub-ppm detection limit can be achieved for some heavy metals at superior beamlines.51
C. Evidence of metazeunerite–metatorbernite occurrence and heterogeneous alteration by Raman and SEM-EDX spectroscopy
To reveal the signatures of possible occurrence of minor uranyl microphases, we used Raman spectroscopy. Raman spectroscopy takes advantage of fast speciation analysis due to the unique positions of the vibrational bands for metals and ligands.20,28 The analysis of microcrystals selected from the surface and the cavity of the rock (see Fig. 4 and S3†) revealed clear signatures in U speciation. Raman spectra exhibited vibrational bands characteristic of uranyl (UO22+), arsenate (AsO43−) and phosphate (PO43−): 326 cm−1 corresponds to ν2(AsO43−), and 404 cm−1 and 458 cm−1 correspond to ν4(AsO43−) and ν4(PO43−) bending modes, respectively (Fig. 4a).20 A double peak was distinguished for ‘r2’ at ∼440 cm−1 and ∼460 cm−1. While the first peak was characteristic of ν2,4(PO43−), the second peak arising at ∼460 cm−1 was likely more sensitive to ν4(AsO43−) bending vibration.20 No information on the peak detected at ∼493 cm−1 was found in the available literature. The most intense and typical bands arising at 817–823 cm−1 originated from the ν1(UO22+) symmetric stretching vibration (Fig. 4b). Two less intense bands at 892 cm−1 corresponded to ν3(UO22+) antisymmetric stretching vibration in metazeunerite (‘r1’) and 992 cm−1 to ν3(PO43−) in metatorbernite, respectively.20,28 Another weak vibrational band normally resolved in metatorbernite 900–905 cm−1 was attributed to ν3(UO22+) stretching vibration. Some broadening of the ν1(UO22+) band in both spectra might be a result of the overlapping with ν1(AsO43−) at 815 cm−1.26 Analysis of the Raman spectra for different natural metazeunerite mineral species summarized in the RRUFF database gives average ν1(UO22+) = 815 cm−1,52 and agrees with our value, 817 cm−1. Some shifts of the frequencies could be attributed to the presence of AsO43− and/or other fractions in each species and settings of the Raman spectrometer. The evidence that PO43− might be present in ‘r2’ is supported by a spectrum feature distinguishable at ∼825–830 cm−1 and by an additional band arising at 992 cm−1, characteristic of PO43−. Deconvolution of ‘r2’ spectrum gives two peaks at 817 cm−1 and 827 cm−1, which agree well with the ν1(UO22+) values of metazeunerite and metatorbernite, respectively (Fig. S5†).28,53
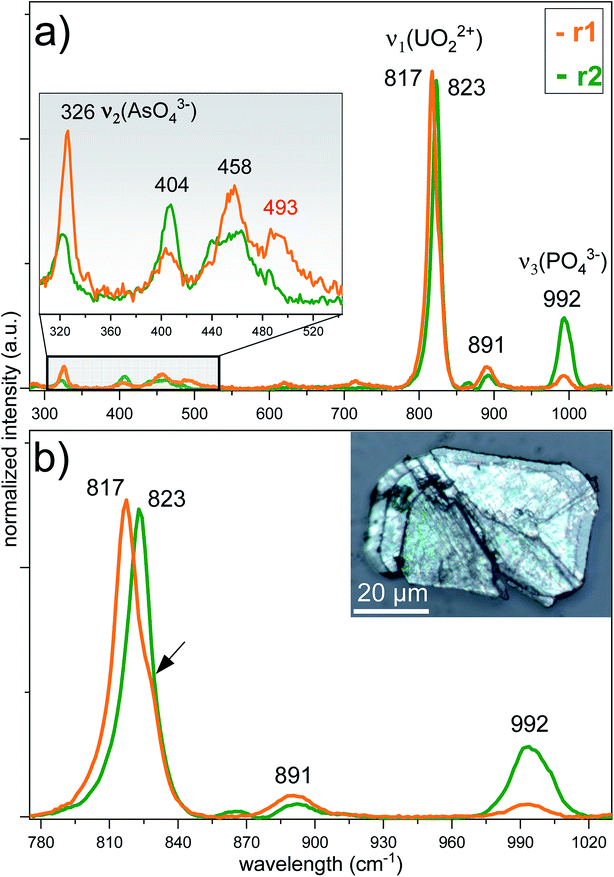 |
| Fig. 4 Raman spectra of two different green crystals selected from the surface (‘r1’, analysed by μ-PXRD corresponds to metazeunerite) and from the cavity (‘r2’) of the rock with zoomed 290–550 cm−1 region (a); Raman spectra for the 780–1020 cm−1 region. Image of the ∼50 μm size flat-shaped microcrystal selected from rock's cavity done with Raman spectrometer for ‘r2’. Arrow indicates on the spectral feature in ‘r1’ presumably referring to U-phosphate phase (b). | |
SEM-EDX analysis performed on ∼200 μm green crystals selected from the surface of the rock showed the presence of major elements: Cu, U, As and P with traces of Si and Fe (Fig. 5, Table 1). SEM reveals two discrete parts, a well-preserved part with a clearly defined pyramidal part with a terminated top plane, and a heavily corroded part without any distinguishable shape. Dark grey parts detected on the SEM image belong to goethite debris as well as lighter parts from quartz. EDX analysis of four different microcrystal parts shows the highest variation in As and P contents around all analysed spots (see Table 1). The part around spot ‘1’ belongs to a well-preserved crystal part, while ‘2–3–4’ are presumably from the overgrown layer, based on O XRF mapping, with heavily corroded part around spots ‘3’ and ‘4’. The most significant variation in elemental composition was found for major elements, Cu, U, As and P, analysed in ‘2’and ‘4’. The latter was associated with a decrease in the U and P contents and a double fold increase in the As content close to that for the theoretical composition of metazeunerite (Table 1).
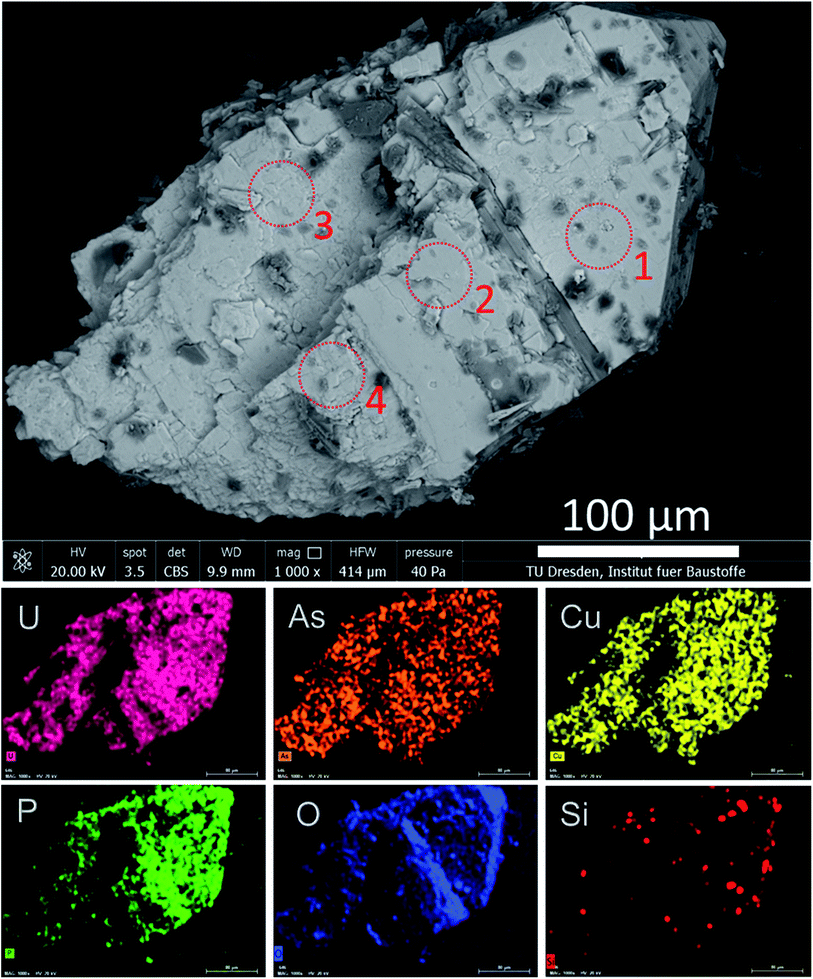 |
| Fig. 5 SEM-EDX analysis for a green microcrystal selected from the surface of the granite rock. Dark grey areas on the SEM image correspond to Fe from the goethite debris. Red dotted circles show the spots at which EDX measurements were performed (Table 1). | |
Table 1 EDX semi-quantitative analysis of a green microcrystal (values normalized to 100% and values given in wt%, EDX sensitivity for U is estimated at 0.1 wt%, deviation ± 1σ, n.d. – value not determined). Theoretical composition for metatorbernite (mt) and metazeunerite (mz)
Analyzed part |
Cu |
U |
As |
P |
O |
H |
1 |
8.9 ± 0.6 |
52.3 ± 1.5 |
11.8 ± 1.0 |
2.8 ± 0.2 |
24.2 ± 4.7 |
n.d. |
2 |
7.5 ± 0.7 |
55.8 ± 2.3 |
7.8 ± 0.6 |
2.1 ± 0.3 |
26.8 ± 7.2 |
n.d. |
3 |
9.3 ± 0.6 |
55.9 ± 1.9 |
10.9 ± 1.1 |
0.9 ± 0.1 |
22.9 ± 5.4 |
n.d. |
4 |
11.5 ± 0.6 |
49.2 ± 1.7 |
14.1 ± 0.8 |
0.8 ± 0.1 |
24.3 ± 4.2 |
n.d. |
mt |
6.78 |
50.77 |
0 |
6.61 |
34.12 |
1.72 |
mz |
6.2 |
46.42 |
14.61 |
0 |
31.20 |
1.57 |
Discussion
The Krunkelbach area represents a potentially rich source of As originating mainly from the zeunerite reported as the main secondary U mineral species occurring at the site with several individual U–As species described elsewhere (see Table S1 and Fig. S6†).22 The formation of metazeunerite and metazeunerite–metatorbernite species is likely to take place after oxidative leaching of uraninite, arsenopyrite, chalcopyrite (CuFeS1–2), enargite (Cu3AsS4), and tennantite (Cu12(Zn,Fe)2As4S13) as potential sources of As and Cu. Phosphorus is released from the host rocks once slightly acidic or close-to-neutral conditions prevail (pH ≤ 7).22,44 Uranyl silicates are precipitated earlier from groundwaters under slightly alkaline conditions, resulting in a more complex U mineralisation.5,34,44 The knowledge of degradation properties for these environmentally relevant compounds is scarce. The correlation between U and As assumes that the complexation would strongly affect the geochemistry of these two elements. The stability of uranyl-arsenates can be understood in terms of paragenesis of U minerals, where U–As together with structurally identical U phosphates form complexes exhibiting extremely low solubility and, thus, high stability to groundwaters.54 Owing to their low solubilities, U–P and U–As are considered important phases controlling U speciation in the near-surface environment as well as U mobility in natural systems, including different types of groundwaters.55,56 Although U–P is one of the most widespread and abundant environmental species, i.e. metatorbernite, the data about U–As phases are limited to some extent. Additional limitations in assessing the geochemical behavior of U–As systems are also due to the lack of reliable thermodynamic data for pH > 6.57
The U concentration in groundwaters in some of the Krunkelbach mine's sections has been analysed to range from ppb to a few ppm U level. The sharp increase in the U concentration is attributed to the oxidation of the uraninite after the intrusion of oxygenated groundwater.17 The analysis of available data for U distribution in groundwaters and tap waters shows high median U contents in tap water at 0.76 μg L−1 for the region of Baden-Württemberg in Southern Germany.58 The elevated As content is reported in regions of Bad Herrenalb and Baden-Baden, where thermal and groundwaters mobilize As from sediments and minerals.59 A region of Baden-Baden is known for the occurrence of the rich U–As mineralisation, i.e. metakirchheimerite (Co(UO2)2(AsO4)2·8H2O), as one of the potential sources of both U and As the groundwaters.43
The Krunkelbach deposit has exceptionally rich U mineralogy with more than 40 secondary U mineral species described at a relatively small area of the 240 m deep mine and the surrounding area (see Table S1 and Fig. S6†). The mineral phases include uraninite, uranyl peroxide studtite ([(UO2)(O2)(H2O)2]·H2O), and ianthinite.60,61 The latter is considered as an important intermediate species in the paragenesis of U minerals and proposed as a potential mineral phase capable of hosting IV–V valent An, i.e. Pu(IV) and Np(V).61 The oxidation of uraninite is related to penetration oxygenated water from the water-bearing fractures resulting in the formation of numerous secondary uranyl species. The oxidation processes are estimated to begin 250–350k y ago and continue up to date, causing a loss of up to 10% of initial U inventory.17,22 The location may be therefore considered as a potential natural analogue site for an operational SNF repository for which safety requirements imply safe storage of the SNF material for more than 100k y. The detailed knowledge of the geological history, geochemistry and degradation properties of secondary U phases are therefore crucial for the assessment of the suitability of the geological sites for long-term SNF storage. The mineralogy of SNF will ultimately determine its durability to self-irradiation effects, and chemical corrosion with subsequent release of the radionuclides. Under specific geochemical conditions, uranyl phases might serve as solubility controls restricting U migration even when present in highly mobile U(VI) form.18,34 During the investigation of the Krunkelbach U deposit both unaltered and altered ores analysed for the As content showed minor release from initially estimated ∼1200 ppm As content in unaltered rock.17 However, much more soluble uranyl phosphate species are reported to restrict U removal by the formation of several earth-alkaline uranyl phosphates, i.e. uranocircite (Ba(UO2)2(PO4)2·8H2O) due to higher phosphorus mobility released after oxidative weathering from the related rocks.22 A minor loss of P and As here was associated with the high release of U owing to its oxidative leaching as a geochemically mobile U(VI) species. The retardation of the mobilized U has been identified on clay colloids and Ba-phosphate minerals and through precipitation of individual U(VI) mineral species.
More generally, depending on specific geochemical conditions, U(VI) minerals are important species controlling mobility of actinides at ore reprocessing and mining sites. Uranyl silicates, uranophane (Ca(UO2)2(SiO3OH)2·5H2O) and cuprosklodowskite and metatorbernite group of minerals are reported to control U speciation at the contaminated Hanford and Oak Ridge sites36,62,63 Similarly, uranophane and haiweeite (Ca(UO2)2Si5O12(OH)2·3H2O) were found to determine U mobility at the Forsmark, a proposed host for radioactive waste repositories in Sweden.64 In another hydrothermal type U deposit in Southern France, U was found in weathered waste rocks to occur as uranyl phosphate comparable to autunite (Ca(UO2)2(PO4)2·10–12H2O) linked with monodentate PO43− and U(VI) species immobilized on clay minerals.29 Different secondary U species, i.e. uranocircite, and metazeunerite, dominate in the area of the Krunkelbach U deposit resulting after high Ba (higher Ba/Ca ratio) and As contents in the groundwaters and their preferential fixation on altered uraninite.17,22 Further oxidative dissolution of uraninite from the microcavities and surface of the rock favors the release of these elements as well as P, W, Pb, Si and Fe into the environment.65
Conclusion
Herein, we demonstrated how a combination of synchrotron and laboratory techniques can be utilized for a rapid elemental and mineralogical analysis of altered granitic rock systems without any complicated sample preparation and treatment procedures. Based on this analytical approach, a multiphase uranium mineralisation with cuprosklodowskite coatings and metazeunerite–metatorbernite microcrystalline species were identified. We showed the evidence for the microscale chemical and morphological heterogeneities of the metazeunerite–metatorbernite phase and their alteration. The ∼200 μm size microcrystalline species collected from the surface of a rock are close to metazeunerite with high As/P ratio and exhibit altered, uneven morphology, while the species collected from the cavities of the rock is a well-preserved phase close to metatorbernite. A high degree of the phase alteration in surface species could be attributed to local geochemical conditions i.e., continuous interaction with the intruding groundwaters. In the recent study, metazeunerite–metatorbernite species has been identified in the soils at another abandoned U mine in the Southern UK, attributed to intensive U mining activities.18 In this context, the stability of the secondary phases and estimation of their long-term behaviour become crucial for predicting the mobilization of radionuclides. Additional research can be focused on the investigation of the thermodynamic and degradation properties of the metazeunerite–metatorbernite phases with varying As and P contents,66 and analysis of U and As speciation in soil systems. The Cu-bearing uranyl arsenate–phosphate mineralization potentially occurs in several hydrothermal type locations including abandoned U mines, geological formations considered for the storage of SNF around hydrothermal U deposits of the orogenic belt in Western and Central Europe.40 A similar experimental methodology described and introduced here can be utilized for the investigation of various environmental systems, i.e., sediments and soils. However, some geological systems with low contents of environmental radionuclides might need additional experimental approach: selective chemical leaching and radiometric analysis.
Statement of contributions
I. P. and K. O. K. designed research; I. P., S. B., I. S., D. D., L. V., D. B., R. v. S. performed μ-XRF and μ-XANES measurements; K. O. K., S. B., R. v. S., I. S. measured HERFD-XANES; I. P. and S. H. performed SEM-EDX analysis; L. A. performed theoretical calculations of XANES spectra for U mineral references; R. J. B. provided U mineral references; J. G. measured μ-PXRD; I. P. measured Raman; I. P., S. B., R. J. B, S. N. K. and K. O. K. wrote the manuscript.
Conflicts of interest
Authors declare no conflict of interests.
Acknowledgements
This work was supported by European Research Council (ERC) Starting Grant No 759696. I. P. is grateful to Steffen Möckel (Alpha Geophysik GmbH) for providing granite sample. K. O. K. and S. N. K. acknowledge support by the Ministry of Education and Science of the Russian Federation under grant no. 075-15-2019-1891.
Notes and references
- R. Hähne and G. Altmann, Principles and Results of Twenty Years of Block-Leaching of Uranium Ores by Wismut GmbH, Germany, IAEA-TECDOC-720, IAEA, Wien, 1993 Search PubMed.
- N. Florea and G. Duliu Octavian, J. Hazard. Toxic Radioact. Waste, 2013, 17, 230–236 CrossRef CAS.
- A. Abdelouas, Elements, 2006, 2, 335–341 CrossRef CAS.
- J.-C. Petit, J. Geochem. Explor., 1992, 46, 1–33 CrossRef CAS.
- R. J. Baker, Coord. Chem. Rev., 2014, 266–267, 123–136 CrossRef CAS.
- P. C. Burns, R. C. Ewing and A. Navrotsky, Science, 2012, 335, 1184 CrossRef CAS PubMed.
- R. C. Ewing, Nat. Mater., 2015, 14, 252 CrossRef CAS PubMed.
- B. E. Burakov, M. I. Ojovan and W. E. Lee, Crystalline Materials for Actinide Immobilisation, Imperial College Press, 2010 Search PubMed.
- M. Laraia, in Radioactive Waste Management and Contaminated Site Clean-Up, ed. W. E. Lee, M. I. Ojovan and C. M. Jantzen, Woodhead Publishing, 2013, pp. 301–326, DOI:10.1533/9780857097446.1.301.
- W. Miller, R. Alexander, N. Chapman, J. C. McKinley and J. A. T. Smellie, Geological Disposal of Radioactive Wastes and Natural Analogues, Elsevier Science, 2000 Search PubMed.
- P. C. Burns, Can. Mineral., 2005, 43, 1839–1894 CrossRef CAS.
- P. Gamaletsos, The Handbook of Environmental Chemistry, 2016, vol. 40, pp. 77–113 Search PubMed.
- I. A. Katsoyiannis, S. J. Hug, A. Ammann, A. Zikoudi and C. Hatziliontos, Sci. Total Environ., 2007, 383, 128–140 CrossRef CAS PubMed.
- Y. Wang, P. Le Pape, G. Morin, M. P. Asta, G. King, B. Bártová, E. Suvorova, M. Frutschi, M. Ikogou, V. H. C. Pham, P. L. Vo, F. Herman, L. Charlet and R. Bernier-Latmani, Environ. Sci. Technol., 2018, 52, 3431–3439 CrossRef CAS PubMed.
- E. Curti, A. Puranen, D. Grolimund, D. Jädernas, D. Sheptyakov and A. Mesbah, Environ. Sci.: Processes Impacts, 2015, 17, 1760–1768 RSC.
- Y. He, Y. Xiang, Y. Zhou, Y. Yang, J. Zhang, H. Huang, C. Shang, L. Luo, J. Gao and L. Tang, Environ. Res., 2018, 164, 288–301 CrossRef CAS PubMed.
- B. A. Hofmann, MRS Proceedings, 2011, 127, 921 CrossRef.
- C. L. Corkhill, D. E. Crean, D. J. Bailey, C. Makepeace, M. C. Stennett, R. Tappero, D. Grolimund and N. C. Hyatt, npj Mater. Degrad., 2017, 1, 19 CrossRef.
- M. A. Denecke, A. Somogyi, K. Janssens, R. Simon, K. Dardenne and U. Noseck, Microsc. Microanal., 2007, 13, 165–172 CrossRef CAS PubMed.
- R. J. P. Driscoll, D. Wolverson, J. M. Mitchels, J. M. Skelton, S. C. Parker, M. Molinari, I. Khan, D. Geeson and G. C. Allen, RSC Adv., 2014, 4, 59137–59149 RSC.
- J. Essilfie-Dughan, M. J. Hendry, J. Warner and T. Kotzer, in The New Uranium Mining Boom: Challenge and Lessons learned, ed. B. Merkel and M. Schipek, Springer Berlin Heidelberg, Berlin, Heidelberg, 2012, pp. 325–334, DOI:10.1007/978-3-642-22122-4_38.
- B. Hofmann, Genese, Alteration und rezentes Fliess-System der Uranlagerstätte Krunkelbach (Menzenschwand, Südschwarzwald), NAGRA Technischer
Bericht 88-30, 1989 Search PubMed.
- U. Noseck, T. Brasser, J. Suksi, V. Havlová, M. Hercik, M. A. Denecke and H.-J. Förster, Phys. Chem. Earth, 2008, 33, 969–977 CrossRef.
- M. Paul, T. Metschies, M. Frenzel and J. Meyer, in The New Uranium Mining Boom: Challenge and Lessons learned, ed. B. Merkel and M. Schipek, Springer Berlin Heidelberg, Berlin, Heidelberg, 2012, pp. 689–699, DOI:10.1007/978-3-642-22122-4_79.
- U. Schwertmann, Plant Soil, 1991, 130, 1–25 CrossRef CAS.
- R. Vochten and A. Goeminne, Phys. Chem. Miner., 1984, 11, 95–100 CrossRef CAS.
- C. Frondel, Systematic mineralogy of uranium and thorium, Report 1064, 1958 Search PubMed.
- E. Faulques, N. Kalashnyk, F. Massuyeau and D. L. Perry, RSC Adv., 2015, 5, 71219–71227 RSC.
- A. Tayal, S. D. Conradson, A. Kanzari, F. Lahrouch, M. Descostes and M. Gerard, RSC Adv., 2019, 9, 11762–11773 RSC.
- H. A. Thompson, G. E. Brown, Jr and G. A. Parks, Am. Mineral., 1997, 82, 483–496 CAS.
- N. P. Edwards, S. M. Webb, C. M. Krest, D. van Campen, P. L. Manning, R. A. Wogelius and U. Bergmann, J. Synchrotron Radiat., 2018, 25, 1565–1573 CrossRef CAS PubMed.
- C. Hall, P. Barnes, J. K. Cockcroft, S. D. M. Jacques, A. C. Jupe, X. Turrillas, M. Hanfland and D. Häusermann, Anal. Commun., 1996, 33, 245–248 RSC.
- E. A. Stefaniak, A. Alsecz, R. Frost, Z. Máthé, I. E. Sajó, S. Török, A. Worobiec and R. Van Grieken, J. Hazard. Mater., 2009, 168, 416–423 CrossRef CAS PubMed.
- K. Maher, J. R. Bargar and G. E. Brown, Inorg. Chem., 2013, 52, 3510–3532 CrossRef CAS PubMed.
- L. Newsome, K. Morris and J. R. Lloyd, Chem. Geol., 2014, 363, 164–184 CrossRef CAS.
- J. P. McKinley, J. M. Zachara, C. Liu, S. C. Heald, B. I. Prenitzer and B. W. Kempshall, Geochim. Cosmochim. Acta, 2006, 70, 1873–1887 CrossRef CAS.
- C. A. Cravotta and G. R. Watzlaf, in Handbook of Groundwater Remediation using Permeable Reactive Barriers, ed. D. L. Naftz, S. J. Morrison, C. C.Fuller and J. A.Davis, Academic Press, San Diego, 2003, pp. 19–66, DOI:10.1016/B978-012513563-4/50006-2.
- C. C. Fuller, J. R. Bargar, J. A. Davis and M. J. Piana, Environ. Sci. Technol., 2002, 36, 158–165 CrossRef CAS PubMed.
- B. Hofmann and J. Eikenberg, Econ. Geol., 1991, 86, 1031–1049 CrossRef CAS.
- H. W. Bültemann and W. D. Bültemann, The Uranium Deposit Krunkelbach in the Southern Black Forest, IAEA-TECDC-361, Federal Republic of Germany, 1986 Search PubMed.
- S. Bauters, P. Tack, J. H. Rudloff-Grund, D. Banerjee, A. Longo, B. Vekemans, W. Bras, F. E. Brenker, R. van Silfhout and L. Vincze, Anal. Chem., 2018, 90, 2389–2394 CrossRef CAS PubMed.
- A. Hammersley, J. Appl. Crystallogr., 2016, 49, 646–652 CrossRef CAS.
- K. Walenta, Tschermaks mineralogische und petrographische Mitteilungen, 1964, vol. 9, pp. 111–174 Search PubMed.
- S. V. Krivovichev and J. Plášil, in Uranium - Cradle to Grave, ed. P. C. Burns and G. E. Sigmon, The Mineralogical Association of Canada, Winnipeg, MB, 2013, pp. 43–49 Search PubMed.
- K. Walenta, F. Hatert, T. Theye, F. Lissner and K. Röller, Eur. J. Mineral., 2009, 21, 515–520 CrossRef CAS.
- C. Den Auwer, E. Simoni, S. Conradson and C. Madic, Eur. J. Inorg. Chem., 2003, 3843–3859, DOI:10.1002/ejic.200300093.
- K. O. Kvashnina and A. C. Scheinost, J. Synchrotron Radiat., 2016, 23, 836–841 CrossRef CAS PubMed.
- I. Pidchenko, K. O. Kvashnina, T. Yokosawa, N. Finck, S. Bahl, D. Schild, R. Polly, E. Bohnert, A. Rossberg, J. Göttlicher, K. Dardenne, J. Rothe, T. Schäfer, H. Geckeis and T. Vitova, Environ. Sci. Technol., 2017, 51, 2217–2225 CrossRef CAS PubMed.
- J. Rothe, M. Altmaier, R. Dagan, K. Dardenne, D. Fellhauer, X. Gaona, E. González-Robles Corrales, M. Herm, O. K. Kvashnina, V. Metz, I. Pidchenko, D. Schild, T. Vitova and H. Geckeis, Geosciences, 2019, 9, 91 CrossRef CAS.
- I. Pidchenko, F. Heberling, K. O. Kvashnina, N. Finck, D. Schild, E. Bohnert, T. Schäfer, J. Rothe, H. Geckeis and T. Vitova, J. Phys. Conf. Ser., 2016, 712, 012086 CrossRef.
- A. Manceau, M. Enescu, A. Simionovici, M. Lanson, M. Gonzalez-Rey, M. Rovezzi, R. Tucoulou, P. Glatzel, K. L. Nagy and J.-P. Bourdineaud, Environ. Sci. Technol., 2016, 50, 10721–10729 CrossRef CAS PubMed.
- B. Lafuente, R. T. Downs, H. Yang and N. Stone, Highlights in Mineralogical Crystallography, 2015, DOI:10.1515/9783110417104-003.
- R. L. Frost, M. L. Weier and M. O. Adebajo, Thermochim. Acta, 2004, 419, 119–129 CrossRef CAS.
- S. L. Korzeb, E. E. Foord and F. E. Lichte, Can. Mineral., 1997, 35, 135–144 CAS.
- R. Finch and T. Murakami, in Uranium: Mineralogy, Geochemistry, and the Environment, 1999, vol. 38, ch. 91–180 Search PubMed.
- T. Murakami, T. Ohnuki, H. Isobe and T. Sato, Am. Mineral., 1997, 82, 888–899 CAS.
- B. J. Merkel, in The New Uranium Mining Boom: Challenge and Lessons learned, ed. B. Merkel and M. Schipek, Springer Berlin Heidelberg, Berlin, Heidelberg, 2012, pp. 627–633, DOI:10.1007/978-3-642-22122-4_72.
- M. Birke, U. Rauch, H. Lorenz and R. Kringel, J. Geochem. Explor., 2010, 107, 272–282 CrossRef CAS.
- K. Bender, Herkunft und Entstehung der Mineral- und Thermalwässer im nördlichen Schwarzwald, PhD thesis, University of Heidelberg, 1995.
- N. Belai, M. Frisch, E. S. Ilton, B. Ravel and C. L. Cahill, Inorg. Chem., 2008, 47, 10135–10140 CrossRef CAS PubMed.
- P. C. Burns, R. J. Finch, F. C. Hawthorne, M. L. Miller and R. C. Ewing, J. Nucl. Mater., 1997, 249, 199–206 CrossRef CAS.
- D. H. Phillips, D. B. Watson and Y. Roh, Environ. Sci. Technol., 2007, 41, 7653–7660 CrossRef CAS PubMed.
- J. E. Stubbs, D. C. Elbert, D. R. Veblen and C. Zhu, Environ. Sci. Technol., 2006, 40, 2108–2113 CrossRef CAS PubMed.
- L. Krall, B. Sandström, E.-L. Tullborg and L. Z. Evins, Appl. Geochem., 2015, 59, 178–188 CrossRef CAS.
- J. Finch Robert, L. Miller Mark and C. Ewing Rodney, Radiochim. Acta, 1992, 58–59, 433 Search PubMed.
- J. Kulaszewska, S. Dann, P. Warwick and C. Kirk, Philos. Trans. R. Soc. A, 2019, 377, 20180242 CrossRef CAS PubMed.
Footnote |
† Electronic supplementary information (ESI) available: Selected U L3 edge μ-XANES spectra plotted without Y axis offset, theoretical calculations for U L3 edge HERFD-XANES spectra, microphotograph of the rock cavities revealing flat shaped green microcrystals, details for U L3 edge HERFD-XANES measurements, U L3 edge HERFD-XANES spectra collected from a whole rock, deconvolution of Raman spectrum, list of U minerals identified in the Krunkelbach mine. See DOI: 10.1039/d0ra03375h |
|
This journal is © The Royal Society of Chemistry 2020 |