DOI:
10.1039/D0RA03307C
(Paper)
RSC Adv., 2020,
10, 24079-24094
Treatment of oil refinery effluent using bio-adsorbent developed from activated palm kernel shell and zeolite†
Received
13th April 2020
, Accepted 11th June 2020
First published on 24th June 2020
Abstract
This study investigated the potential of palm kernel shell (PKS) as a biomass feed for adsorbent production. This work aims at synthesizing green adsorbent from activated PKS by integrating iron oxide and zeolite. The newly developed adsorbents, zeolite-Fe/AC and Fe/AC, were analyzed for surface area, chemical composition, magnetic properties, crystallinity, and stability. The adsorbent efficiency in removing effluent from the palm oil mill was evaluated. The influence of operating parameters, including adsorbent dosage, H2O2, reaction time, and initial solution pH for adsorption performance was studied. The Fourier transform infrared analysis revealed that the adsorbents contain functional groups including OH, N–H, C
O and C
C, which are essential for removing pollutants. The SEM-EDX analysis shows holes in the adsorbent surface and that it is smooth. The adsorption study revealed that under optimized conditions, by using 4 g L−1 of adsorbent and 67.7 mM H2O2, zeolite-Fe/AC was able to remove 83.1% colour and 67.2% COD within 30 min. However, Fe/AC requires 5 g L−1 of adsorbent and 87.7 mM to remove 86.8 percent and 65.6 percent, respectively. This study also showed that zeolite-Fe/AC has higher reusability compared to Fe/AC. Among Freundlich and Temkin models, the experimental data were found to be best fitted with the Langmuir isotherm model. The kinetic analysis revealed that for both adsorbents, the adsorption process fitted the pseudo-second-order model (R2 = 0.9724). The finding reflects monolayer adsorption of zeolite-Fe/AC and Fe/AC. This study thus demonstrates the applicability of low-cost green adsorbents produced from PKS to treat oil refinery effluent and other recalcitrant wastewaters.
1 Introduction
Approximately 1500 million tons of agricultural waste is produced and disposed of annually in landfills.1 Researchers have recently devoted much attention to converting agricultural waste into useful products such as green adsorbents, biofuels, enzymes, vitamins, antioxidants, animal feed, antibiotics, and other chemicals.2 The use of agricultural waste adsorbents has several benefits over the use of conventional adsorbents, such as better biodegradability, high abundance and simple methods of collection and preparation. Livestock waste also has a better surface area of active sites, and functional groups such as hydroxyl, amino and carboxylic groups leading to high adsorption efficiency.3 Based on the published results, peanut shells, coconut shell,4,5 banana peel,6 palm kernel shell,7 garlic peel8,9 and rice husks10 can be used as an efficient and environmentally friendly bio-adsorbent for the removal of various heavy metals, anionic and cationic dyes, and persistent organic pollutants. A low-cost, readily available adsorbent should be formulated because adsorption is one of the most efficient treatment technologies for the removal of extremely recalcitrant pollutants especially from chemical and pharmaceutical production. This is because industrial wastewaters often contain substances that need to be treated before being discharged into a biological treatment plant and subsequent water bodies.
In Malaysia, palm oil production is one of the major agricultural industries, providing 37.9% of the agricultural contribution to GDP.11 However, every ton of fresh fruit bunches (FFB) processed produces different types of wastes such as 60 per cent of palm oil mill effluent (POME), 23 per cent of empty fruit bunches (EFB), 12 per cent of mesocarp fibers and 5 per cent palm kernel shell.6 Among them, POME is the largest wastes generated from the palm oil mills production with high BOD, COD, TS, TSS, colour more than 500 ADMI etc.12 POME is mainly generated from crude palm oil (CPO) production line through sterilizer condensate, sludge clarification and hydrocyclone.13 Approximately, 5 to 7.5 tons of POME discharge of every ton of fresh fruit bunches (FFB) processed.12 Since POME is considered as highly recalcitrant, the Department of Environment is started to be more stringent in standard discharge limits to control water pollution. The characteristics of the raw POME and DOE standard discharge limits has been summarized in Table 1.12,14
Table 1 Characteristics of raw POME and DOE standard discharge limits
Parameters |
Average value |
DOE discharge limit (Malaysia) |
Temperature (°C) |
85 |
45 |
pH |
4.2 |
5–9 |
Biochemical oxygen demand, BOD (mg L−1) |
25 000 |
100 |
Chemical oxygen demand, COD (mg L−1) |
51 000 |
— |
Oil & grease, O&G (mg L−1) |
6000 |
50 |
Total solids, TS (mg L−1) |
40 000 |
1500 |
Total suspended solids, TSS (mg L−1) |
18 000 |
400 |
Total volatile solids, TVS (mg L−1) |
34 000 |
— |
Total nitrogen (mg L−1) |
750 |
200 |
Colour (ADMI) |
>500 |
200 |
Presently various technologies including advanced oxidation processes,15 membrane technology16 and chemical coagulation and flocculation,17,18 adsorption,19,20 nanofiltration21 have been successfully used to treat POME. Among them, processes has attracted much researcher due to its simplicity, lower operating cost and higher efficiency compared to physical and chemical techniques.20,22 However, one of the main challenges in the application of adsorption processes, is the selection of suitable material as the cost of operation can be significantly reduced with the usage cheaper and environmentally friendly adsorbents. So, the researchers are finding alternative adsorbents which are easily available and cheaper. Adsorbents derived from agricultural waste such as fruit peels, tea waste, fruit seeds, and bagasse consists of lignin, cellulose, potentially makes them effective adsorbent for the removal of various organic and inorganic pollutants.
On the other hand, palm kernel shell (PKS) is one of the significant waste discharging from the palm oil mill industry, producing approximately 2 million tons annually.6,23 PKS characteristics, such as large cavities and porosity, inexpensive, readily available and adsorption affinity, can be an excellent organic sorbents for pollutant removal.24,25 Past studies have confirmed that PKS could remove heavy metals, dyes, persistent organic pollutants and organic contaminants.26,27 Most raw PKS, however, do not have adequate adsorption efficiency, stability, good separation, and relevant use in real wastewater. Gautam and others (2013) and Zhang and others (2020) reported that various methods such as chemical modification, physical modification, biological modification, mineral impregnation, and magnetic modifications could be used to improve the efficiency of raw biomass.28,29 PKS composite adsorbents can also increase their adsorption performance by combining with other powerful adsorbents, inorganic compounds and organic compounds based on previous studies.29–31 Many materials can be used to hybridize or combined with biomass adsorbent such as iron oxide, titanium dioxide,32 graphene oxide,33 magnesium oxide,34 zeolite, polymer,35 spent shiitake substrate,36 sulfone37 and others to increase its active sites for better adsorbent performance.
Currently, iron species such as zero-valent iron Fe3O4, Fe2O3, FeS, and other iron core are extensively used for adsorbent modification for increasing the surface area and hydraulic conductivity. Magnetically modified biomass adsorbents have attracted much attention with the advantages of cost-effectiveness, environmental friendly, high reactivity, facile availability, and easily recovered due to magnetic property.38–40 Based on the previous studies, various types of low-cost biomass such as wheat straw,41 macroalgae biomass42 and sugarcane bagasse43 have been incorporated with iron oxides to remove both organic and inorganic pollutants. According to the study conducted by Hua and others (2018) incorporation of iron oxide in the biomass adsorbent able to increase the efficiency of separation process and adsorption capacity of adsorbent.44,45 Besides, the presence of oxidant together with iron oxide helps to initiate the Fenton reaction, which produce hydroxyl radicals and aid the adsorption process.46
On the other hand, zeolite is an inorganic material that is known for its ion exchange power, high porosity, large surface area, high regeneration potential, strong acidic stability, readily available and low cost.47 It also has a unique feature that allows other molecular dimensions to move through and shows good cations selectivity.47,48 Many studies also have reported that zeolite is very effective for removal of organic compound and heavy metals from wastewater.49–53 Shavandi and others (2012) has reported that zeolite shows great adsorption capacity particular on POME obtained from aerobic pond on removal of heavy matter.54 Therefore, combining zeolite and iron oxide with activated carbon palm kernel shell could work as a strong capping heterogeneous adsorbent for cationic and anionic ion in adsorption process. The hybrid adsorbent that develop also can offer a great possibility in term of the adsorption efficiency, cycle of regeneration and cost-effectiveness of adsorption process. However, so far in the literature, the application of iron oxide and zeolite for the modification of activated carbon–PKS has not been carried out yet. Therefore, the novelty of this study is the synthesis of hybrid green adsorbent and used it to treat real wastewater, with the aid of oxidant.
Therefore, the aim of this study is to develop green hybrid adsorbent from activated PKS by integrating iron oxide and zeolite. The newly developed adsorbent is used to treat, biologically treated POME through adsorption process. This study would provide a promising choice for a low cost and eco-friendly treatment of POME as well as opens a new renew to utilize by-product of palm oil processing, it is expected that the results in this study may provide some guidance for the step towards zero-discharge and sustainability in the palm oil industry.
2 Methodology
2.1 Materials
Biologically treated POME was collected from local palm oil manufacturer in Rawang Selangor Malaysia, and palm kernel shell activated carbon was purchased from Pacific Activated Carbon, Malaysia. Hydrogen peroxide 33% (H2O2), ferrous sulfate (FeSO4·7H2O), ferric chloride (FeCl3·6H2O), sodium hydroxide (NaOH), sulfuric acid (H2SO4), zeolite and ethanol have been purchased from Sigma-Aldrich (M) Sdn Bhd. All the chemicals were reagent grade and were used without further purification. Ultrapure water was used for the preparation of solutions.
2.2 Synthesis of Fe/AC
5 g of ground activated carbon, 3.66 g FeSO4·7H2O and 6.66 g FeCl3·6H2O were placed into a 500 mL beaker containing 100 mL of ultrapure water. The solution was stirred and heated to 65 °C for mixing propose. After 30 minutes of stirring, the solution was cooled down to 40 °C. Then the pH was adjusted to 10–11 to precipitate the iron hydroxides by using 5 M NaOH solution, and the solution was stirred for an hour. After that, the solution was left overnight and covered with cling film. The pipette was used to remove the supernatant, and then precipitates were washed with ultrapure water first then was rinsed with ethanol. After the ethanol drained, the precipitate was moved to the aluminium tray and dried at 80 °C for about 4 hours. Then, the precipitate was washed by using ultrapure water, and the magnetic rod was used to collect the activated carbon particles. The magnetically activated carbon particles dried at 80 °C overnight. After that, the dried Fe/AC was stored in an airtight bottle.
2.3 Synthesis of zeolite-Fe/AC
2.5 g of the zeolite and 5 g Fe/AC (0.5
:
1) were placed into a 500 mL beaker containing 100 mL of ultrapure water and was stirred for 6 hours at 60 °C. Then the mixture was placed in an ultrasonic bath for 45 min, and the mixture was left at room temperature for 24 hours. The mixture was then washed and centrifuged to separate the adsorbent from the solution. Lastly, the synthesis adsorbent (zeolite-Fe/AC) was dried in the oven at 60 °C overnight.55
2.4 Characterization of adsorbents
The surface functional groups of the synthesized adsorbent were studied using Fourier Transformation Infrared (FTIR) with a Perkin Elmer Spectrometer (Frontier) in the absorption range of 500–4000 cm−1. The morphology and chemical composition of the adsorbent were examined with a Scanning Emission Microscopy (SEM) (Phenom ProX)56 and Energy Dispersive X-ray (EDX). The changes in the morphology of the adsorbent were also analyzed using X-ray Diffraction (XRD) analysis.57 The magnetic properties of the adsorbents were evaluated by vibrating sample magnetometer (VSM) (LakeShore 340).
2.5 Adsorption study
The adsorbent capacity (zeolite-Fe/AC) was evaluated using adsorption processes with the aid of oxidants to treat POME. The initial pH of the POME was adjusted to desire condition by using 0.5 M H2SO4 acid and 1 M NaOH alkaline. The desired amount of zeolite-Fe/AC adsorbent and H2O2 were added into the sample POME solution, and the sample solution was stirred continuously at a constant rate of 200 rpm. At the end of each experiment, the sample solution was obtained and filtered to remove the adsorbent. Thus, colour removal was measured immediately. Right after the colour removal reading was taken, the pH of the sample solution was adjusted to the alkaline base by using 1 M NaOH with the ratio of every 1 mL of 200 mM of H2O2 solution with 10 mL of 1 M NaOH. For the reusability study, the used adsorbent was washed with ultrapure water, followed by 0.1 M NaOH for 10 minutes. After that, the used adsorbent was washed with distilled water thrice. Lastly, the adsorbent was dried in an oven for 1.5 hours and stored for the next experiment. Yet, the performance of the Fe/AC also evaluated through the same procedure as evaluating the performance of zeolite-Fe/AC. All the experiments were repeated twice.
2.6 Experimental design and data collection
Design-Expert Software (Version 10) was used to design the experiments. Response Surface Methodology (RSM) – Central Composite Design (CCD) was used in this study to optimize four independent variables: the dosage of adsorbent, dosage of H2O2, reaction time and pH value. Preliminary experiments were carried to determine the ranges for the operating parameters. The adsorption study was conducted to identify the optimum operating conditions used including dosage of adsorbent 1–5 g L−1, the dosage of H2O2 40–200 mM, reaction time 10 min to 60 min and pH value from 3 to 9. Analysis of variance (ANOVA) was used to evaluate the interaction between independent variables and the responses. The quality of the fit regression model was expressed by the determination coefficient, R2. In terms of statistical significance, Fishers F-test was used. It can determine whether the model was accepted or rejected based on the probability (p-value) with a 95% confidence level.
2.7 Data analysis
UV-Vis spectrophotometer was used to determine the COD and colour removal, according to the American Public Health Association (APHA) standard method. The decolourization efficiency and COD removal have been calculated using eqn (1) and (2). |
 | (1) |
|
 | (2) |
3 Results and discussion
3.1 Characterisation of adsorbents
(b) Scanning Emission Microscopy (SEM). The raw AC displayed a rough, irregular, and amorphous structure as can be seen in S1(a).† However, the morphology of modified activated carbon (Fe/AC) in S1(b)† exhibited a crystal surface with smaller pore sizes. Patches of white crystals may indicate the presence of iron oxide nanoparticles.64 The morphology of zeolite-Fe/AC in S1(c)† shows porous, loose texture and presence of crystal surface with reduced, which confirmed the presence of zeolite particles.62 The result obtained in line with the study reported by Cheng and others (2016).65
(c) Energy Dispersive X-ray (EDX). The chemical compositions of AC, Fe/AC and zeolite-Fe/AC were examined, and the results of the EDX analysis is shown in Tables 2 and S2.† PKS–AC, comprised of 60.7% of C, 24.6% of O. While, Fe/AC consists of lesser carbon (40.9%) and higher oxygen (49.9%) and Fe (9.1%). The reduction of C composition most likely due to the introduction of iron oxide in raw AC. On the other hand, the zeolite-Fe/AC shows the decrease of Fe and O compositions due to the presence of zeolite participles. Zeolite is known as carbon material, so there is an increase in the content of C in zeolite-Fe/AC.58,66
Table 2 Chemical composition of AC and Fe/AC
Material |
C (%) |
O (%) |
Fe (%) |
Ca (%) |
AC |
60.7 |
24.6 |
— |
14.6 |
Fe/AC |
40.9 |
49.9 |
9.1 |
— |
Zeolite-Fe/AC |
61.4 |
34.8 |
3.8 |
— |
(d) X-ray Diffraction (XRD) analysis. Fig. 4 shows the X-ray diffraction (XRD) analyses of both Fe/AC and zeolite-Fe/AC. The iron peaks are identified at θ: 30.2, 32.1, 35.5, 43.3, 53.5, 56.8, 62.7 and 73.9. This result further supports the SEM/EDX analysis that the iron magnetic nanoparticles have been successfully coated and incorporated into PKS. Fig. 4(b) also shows that zeolite-Fe/AC contains lesser iron oxide compared to Fe/AC as zeolite particles have replaced it. Similar results have also reported by Jianhua Qu and others (2020).67
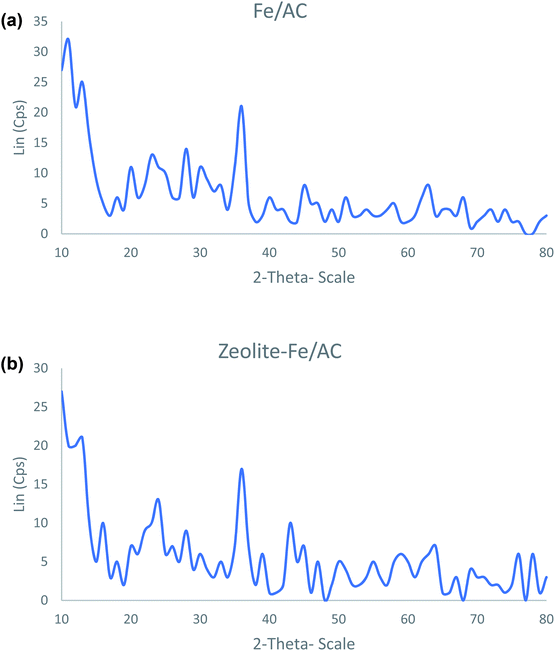 |
| Fig. 4 The XRD spectra of (a) Fe/AC and (b) zeolite-Fe/AC. | |
(e) Vibrating sample magnetometer (VSM). S3† shows that Fe/AC has the saturation magnetization value of about 16.6 emu g−1. Whereas the zeolite-Fe/AC only has a saturation magnetization value of 10.6 emu g−1. Even though zeolite-Fe/AC has lower magnetic properties, it meets the minimum criteria of solid–liquid separation.68 The result obtained in line with the study of Javadian and others (2020).69
3.2 Adsorption study
(a) Experimental design. Tables 3 and 4 summarized the experimental design suggested by RSM. The result clearly shows that both adsorbents managed to remove more than 80% of colour and 70% of COD.
Table 3 Experimental design suggested by RSM, and the result obtained on colour removal
Run |
Dosage of H2O2 (mM) |
Independent variables |
Colour removal efficiency (%) |
Adsorbent (g L−1) |
Reaction time (min) |
pH |
Fe/AC |
Zeolite-Fe/AC |
1 |
120 |
3 |
35 |
6 |
76.5 |
43.9 |
2 |
40 |
1 |
60 |
9 |
69.1 |
24.1 |
3 |
200 |
1 |
60 |
9 |
69.4 |
42.3 |
4 |
40 |
5 |
10 |
3 |
83.7 |
86.1 |
5 |
120 |
3 |
15 |
6 |
78.3 |
43.1 |
6 |
200 |
5 |
60 |
9 |
79.1 |
55.5 |
7 |
40 |
5 |
60 |
9 |
79.9 |
40.8 |
8 |
200 |
1 |
10 |
9 |
70.0 |
41.7 |
9 |
280 |
3 |
35 |
6 |
73.9 |
54.1 |
10 |
120 |
3 |
35 |
6 |
77.9 |
44.9 |
11 |
40 |
1 |
60 |
3 |
76.5 |
62.4 |
12 |
200 |
5 |
10 |
3 |
82.7 |
87.1 |
13 |
40 |
1 |
10 |
9 |
71.4 |
21.7 |
14 |
120 |
3 |
35 |
12 |
74.2 |
67.8 |
15 |
120 |
3 |
35 |
6 |
76.1 |
45.3 |
16 |
120 |
3 |
35 |
6 |
76.1 |
44.5 |
17 |
200 |
1 |
60 |
3 |
73.0 |
68.8 |
18 |
200 |
1 |
10 |
3 |
77.1 |
66.8 |
19 |
120 |
7 |
35 |
6 |
82.7 |
66.6 |
20 |
200 |
5 |
60 |
3 |
80.1 |
93.4 |
21 |
120 |
3 |
35 |
6 |
74.6 |
46.1 |
22 |
40 |
1 |
10 |
3 |
79.5 |
59.6 |
23 |
200 |
5 |
10 |
9 |
80.1 |
55.1 |
24 |
40 |
5 |
10 |
9 |
80.3 |
47.7 |
25 |
120 |
1 |
35 |
6 |
70.0 |
30.4 |
26 |
40 |
5 |
60 |
3 |
76.5 |
93.4 |
27 |
40 |
3 |
35 |
6 |
78.5 |
25.7 |
28 |
120 |
3 |
35 |
0 |
— |
— |
29 |
120 |
3 |
35 |
6 |
76.7 |
46.5 |
30 |
120 |
3 |
85 |
6 |
76.1 |
51.5 |
Table 4 Experimental design suggested by RSM, and the result obtained on COD removal
Run |
Dosage of H2O2 (mM) |
Independent variables |
COD removal efficiency (%) |
Adsorbent (g L−1) |
Reaction time (min) |
pH |
Fe/AC |
Zeolite-Fe/AC |
1 |
120 |
3 |
35 |
6 |
74.7 |
63.7 |
2 |
40 |
1 |
60 |
9 |
65.0 |
55.3 |
3 |
200 |
1 |
60 |
9 |
73.7 |
67.9 |
4 |
40 |
5 |
10 |
3 |
73.0 |
71.1 |
5 |
120 |
3 |
15 |
6 |
73.2 |
67.6 |
6 |
200 |
5 |
60 |
9 |
76.3 |
75.0 |
7 |
40 |
5 |
60 |
9 |
71.0 |
57.1 |
8 |
200 |
1 |
10 |
9 |
72.9 |
66.1 |
9 |
280 |
3 |
35 |
6 |
80.0 |
69.7 |
10 |
120 |
3 |
35 |
6 |
74.7 |
63.7 |
11 |
40 |
1 |
60 |
3 |
71.0 |
58.9 |
12 |
200 |
5 |
10 |
3 |
80.8 |
68.9 |
13 |
40 |
1 |
10 |
9 |
67.0 |
45.8 |
14 |
120 |
3 |
35 |
12 |
70.0 |
70.5 |
15 |
120 |
3 |
35 |
6 |
74.5 |
63.9 |
16 |
120 |
3 |
35 |
6 |
75.0 |
63.4 |
17 |
200 |
1 |
60 |
3 |
75.8 |
69.2 |
18 |
200 |
1 |
10 |
3 |
77.6 |
69.7 |
19 |
120 |
7 |
35 |
6 |
75.5 |
72.4 |
20 |
200 |
5 |
60 |
3 |
79.7 |
75.5 |
21 |
120 |
3 |
35 |
6 |
74.7 |
63.7 |
22 |
40 |
1 |
10 |
3 |
71.0 |
58.4 |
23 |
200 |
5 |
10 |
9 |
75.3 |
70.8 |
24 |
40 |
5 |
10 |
9 |
69.0 |
57.9 |
25 |
120 |
1 |
35 |
6 |
73.4 |
65.3 |
26 |
40 |
5 |
60 |
3 |
75.0 |
71.8 |
27 |
40 |
3 |
35 |
6 |
67.9 |
59.7 |
28 |
120 |
3 |
35 |
0 |
— |
— |
29 |
120 |
3 |
35 |
6 |
74.7 |
63.7 |
30 |
120 |
3 |
85 |
6 |
74.7 |
69.2 |
3.3 Statistical analysis
(a) ANOVA – CCD for colour and COD removal. Tables 5 and 6 shows the analysis of variance (ANOVA) for colour and COD removals for both Fe/AC and zeolite-Fe/AC. Reduced 2FI model and reduced quadratic model were developed for Fe/AC and zeolite-Fe/AC, respectively for the colour removal efficiency. Whereas, the linear model and reduced 2FI were suggested by RSM-CCD for Fe/AC and zeolite-Fe/AC respectively for the COD removal. The final equations of colour and COD removal in terms of coded factors were expressed by eqn (3) and (4), (5) and (6). The positive sign of the terms in the equation implies the synergistic effect while the negative sign suggests antagonistic effect.
Table 5 Analysis of variance (ANOVA) for colour removal efficiencya
Source |
Sum of squares |
df |
Mean square |
F-Value |
p-Value |
A: dosage of H2O2, B: dosage of adsorbent, C: pH value, D: reaction time. |
Fe/AC |
Model |
425.8 |
7 |
60.8 |
45.4 |
<0.0001 |
A – dosage of H2O2 |
8.8 |
1 |
8.8 |
6.5 |
0.0184 |
B – dosage of adsorbent |
283.1 |
1 |
283.1 |
211.5 |
<0.0001 |
C – pH |
60.3 |
1 |
60.3 |
45.0 |
<0.0001 |
D – reaction time |
27.2 |
1 |
27.2 |
20.3 |
0.0002 |
AB |
4.7 |
1 |
4.7 |
3.5 |
0.0763 |
BC |
32.1 |
1 |
32.1 |
24.0 |
<0.0001 |
CD |
10.0 |
1 |
10.0 |
7.4 |
0.0126 |
Lack of fit |
22.4 |
16 |
1.4 |
1.2 |
0.4462 |
R-squared |
0.938 |
Adj R-squared |
0.917 |
Pred R-squared |
0.871 |
Adeq. precision |
24.6 |
Eqn (3) |
76.7–0.6A + 3.4B − 1.7C − 1.2D + 0.5AB + 1.4BC + 0.8CD |
![[thin space (1/6-em)]](https://www.rsc.org/images/entities/char_2009.gif) |
Zeolite-Fe/AC |
Model |
10 098.0 |
9 |
1122.0 |
322.2 |
<0.0001 |
A – dosage of H2O2 |
481.9 |
1 |
481.9 |
138.4 |
<0.0001 |
B – dosage of adsorbent |
2676.5 |
1 |
2676.5 |
768.6 |
<0.0001 |
C – pH |
5557.0 |
1 |
5557.0 |
1595.9 |
<0.0001 |
D – reaction time |
42.0 |
1 |
42.0 |
12.1 |
0.0025 |
AB |
51.7 |
1 |
51.7 |
14.8 |
0.0011 |
AC |
130.8 |
1 |
130.8 |
37.6 |
<0.0001 |
BC |
68.7 |
1 |
68.7 |
19.7 |
0.0003 |
CD |
29.8 |
1 |
29.8 |
8.6 |
0.0086 |
C^2 |
3188.5 |
1 |
3188.5 |
915.7 |
<0.0001 |
Lack of fit |
61.4 |
14 |
4.4 |
4.6 |
0.0511 |
R-squared |
0.993 |
Adj R-squared |
0.990 |
Pred R-Squared |
0.980 |
Adeq. precision |
62.8 |
Eqn (4) |
45.4 + 4.5A + 10.6B − 17.8C + 1.3D − 1.8AB + 2.9AC − 2.1BC − 1.4CD |
Table 6 Analysis of variance (ANOVA) for COD removal efficiencya
Source |
Sum of squares |
df |
Mean square |
F-Value |
p-Value |
A: dosage of H2O2, B: dosage of adsorbent, C: pH value, D: reaction time. |
Fe/AC |
Model |
354.5 |
4 |
88.6 |
77.9 |
<0.0001 |
A – dosage of H2O2 |
230.1 |
1 |
230.1 |
202.3 |
<0.0001 |
B – dosage of adsorbent |
38.4 |
1 |
38.4 |
33.7 |
<0.0001 |
C– pH |
85.4 |
1 |
85.4 |
75.1 |
<0.0001 |
D – reaction time |
0.5 |
1 |
0.5 |
0.5 |
0.5017 |
Lack of fit |
27.2 |
19 |
1.4 |
50.9 |
0.0002 |
R-squared |
0.928 |
Adj R-squared |
0.916 |
Pred R-squared |
0.892 |
Adeq. precision |
29.8 |
Eqn (5) |
73.8 + 3.1A + 1.3B − 2.1C + 0.2D |
![[thin space (1/6-em)]](https://www.rsc.org/images/entities/char_2009.gif) |
Zeolite-Fe/AC |
Model |
896.4 |
5 |
179.3 |
12.5 |
<0.0001 |
A – dosage of H2O2 |
480.4 |
1 |
480.4 |
33.5 |
<0.0001 |
B – dosage of adsorbent |
213.6 |
1 |
213.6 |
14.9 |
0.0008 |
C – pH |
69.4 |
1 |
69.4 |
4.8 |
0.0382 |
D – reaction time |
27.7 |
1 |
27.7 |
1.9 |
0.1776 |
AC |
105.3 |
1 |
105.3 |
7.3 |
0.0125 |
Lack of fit |
329.7 |
18 |
18.3 |
651.8 |
<0.0001 |
R-squared |
0.731 |
Adj R-squared |
0.673 |
Pred R-Squared |
0.542 |
Adeq. precision |
12.9 |
Eqn (6) |
65.5 + 4.5A + 2.9B − 1.9C + 1.1D + 2.6AC |
Tables 5 and 6 present the result of statistical analysis for both adsorbents. The F-values shows that model of both adsorbents was significant at 95% confidence level. For Fe/AC and zeolite-Fe/AC, the F-values were 45.4 and 322.2 respectively for colour removal. While F-values for COD removal for Fe/AC and zeolite-Fe/AC were 77.9 and 12.5. The p-values <0.0500 for A, B, C, D, BC and CD for Fe/AC indicates that the parameters are significant for colour removal. Whereas for zeolite-Fe/AC, A, B, C, D, AB, AC, and CD are significant. On the other hands, for COD removal, A, B and C parameters are significant for Fe/AC, and A, B, C and AC for zeolite-Fe/AC.
The F-value should be insignificant to ensure that the model is fit. The result shows that both models for colour removal are insignificant. In terms of R2 for Fe/AC, colour and COD removal reported having 0.938 and 0.928, respectively. Whereas, zeolite-Fe/AC has the R2 of 0.994 and 0.731 for colour and COD removal, respectively. Both adsorbents reported to have R2 close to 1, and the differences between adjusted and predicted R2 were <0.5, which indicated that the high accuracy of the model.70 Adequate precision compares the range of the predicted values at the design points to the average prediction error. As can be seen in Table 5, both the model for colour removal shows the adequate precision ratios of 24.6 (Fe/AC) and 62.8 (zeolite-Fe/AC). And adequate precision ratios for COD removal of Fe/AC and zeolite-Fe/AC were 29.8 and 12.9, respectively. A ratio greater than 4 indicates that models are sufficient to navigate the CCD-model. S4(a), (b), S5(a) and (b)† shows the graph of predicted versus actual for Fe/AC and zeolite-Fe/AC.
3.4 Optimization and model validation
Table 7 shows the predicted and the experimental results obtained for selected optimum conditions. The experimental values are close enough to the predicted values, with a deviation of less than 5%; therefore, the model is considered reliable for this study. As can be seen in Table 7, Fe/AC had the highest colour removal (86.8%) compared to zeolite-Fe/AC (83.1%). However, zeolite-Fe/AC achieved higher COD removal efficiency of 67.2% compared to Fe/AC.
Table 7 Optimized conditions
Adsorbents |
Dosage of adsorbent (g L−1) |
Dosage of H2O2 (mM) |
Reaction time (min) |
pH value |
Fe/AC |
5 |
84.0 |
30 |
3 |
Zeolite-Fe/AC |
4 |
67.7 |
30 |
3 |
Adsorbents |
Colour removal efficiency (%) |
COD removal efficiency (%) |
Predicted |
Experimental |
Predicted |
Experimental |
Fe/AC |
82.7 |
86.8 |
— |
63.6 |
Zeolite-Fe/AC |
81 |
83.1 |
— |
67.2 |
3.5 Kinetic study
Results of kinetic study for Fe/AC and zeolite-Fe/AC under the optimum condition on colour removal has been presented in Table 8. It shows that the adsorption process happened rapidly within 10 to 30 min in experiment.71,72 There is an only slight increase (<1%) after 6 minutes of the kinetic study experiment as recorded. In the present study, the colour removal efficiency was tested on zero-, first- and second-order reaction kinetic. The kinetics study for zeolite-Fe/AC and Fe/AC with the aid of oxidants shows in S6.† It was found that the regression coefficients, R2 of the second-order reaction kinetic S6(c)† of zeolite-Fe/AC and Fe/AC were 0.9331 and 0.9724 respectively, which are obviously much higher than zero-order (zeolite-Fe/AC, R2 = 0.9211 and Fe/AC, R2 = 0.9412) and first-order (zeolite-Fe/AC, R2 = 0.928 and Fe/AC, R2 = 0.9632) reaction kinetic. By comparing the regression coefficients obtained, it can be concluded that the second-order reaction kinetic fit the reaction best. The results from this study were consistent with the work from 73–75
Table 8 Kinetic study of Fe/AC and zeolite-Fe/AC
Time (min) |
Kinetic study under optimum condition on colour removal (%) |
Fe/AC |
Zeolite-Fe/AC |
2 |
78.9 |
80.5 |
4 |
82.6 |
80.6 |
6 |
83.6 |
83.3 |
8 |
84.9 |
83.7 |
10 |
86.7 |
84.9 |
3.6 Adsorption isotherm study
Adsorption isotherms explained about the amount of adsorbate adsorbed by unit mass of adsorbent from the liquid phase. It is essential to carry out the analysis of adsorption equilibrium data for design optimization of an adsorption system. Adsorption isotherm shows the relationship between adsorbate adsorbed onto active site of adsorbent and the concentration of the solution.76 To date, various isotherms model has been used widely to analyze the adsorption data. In this study, Langmuir, Freundlich and Temkin models have been used to study the interaction of concentration of POME with adsorbent, Fe/AC and zeolite-Fe/AC.
(a) Langmuir adsorption isotherm. The Langmuir isotherm is referred to the monolayer adsorption of adsorbate on the adsorbent, Fe/AC and zeolite-Fe/AC. The linearized form of equation of Langmuir isotherm is as follow:77 |
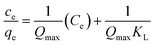 | (7) |
(b) Freundlich adsorption isotherm. Freundlich isotherm suggest multilayer adsorption on the heterogeneous surface; it also explains that the adsorption increase with increase in the concentration. The linear equation of Freundlich adsorption isotherm is shown in eqn (8):78 |
log qe = (1/n)log Ce + log KF
| (8) |
(c) Temkin adsorption isotherm. The Temkin isotherm equation assumes that the heat of adsorption of all the molecules in the layer decreases linearly with coverage due to adsorbent–adsorbate interactions, and that the adsorption is characterized by a uniform distribution of the binding energies, up to some maximum binding energy. The linearized Temkin isotherm is given in eqn (9):where, B = RT/b. KT is the equilibrium binding constant (L mg−1) corresponding to the maximum binding energy and b is a constant related to the heat of adsorption. R is the gas constant (8.314 J mol−1 K−1) and T is the absolute temperature (K). A plot of q versus ln
C enables the determination of the isotherm constants KT and b from the intercept and slope respectively.Temkin isotherm explained the interaction between adsorbent–adsorbate, and it also assumes that the heat of adsorption decreases linearly with coverage. The linearized Temkin isotherm is given in eqn (10)79
|
qe = B1 ln Ce + B1 ln KT
| (10) |
For all isotherm model above, Ce is the concentration of adsorbate adsorbed at equilibrium; qe is the adsorption capacity of adsorbent at equilibrium concentration; Qmax is the maximum adsorption capacity; KL, Kf and KT are the constant in Langmuir, Freundlich Temkin isotherm of adsorption respectively; 1/n is the adsorption intensity; B1 = RT/b; b is a constant related to the heat of adsorption; R is the gas constant (8.314 J mol−1 K−1) and T is the absolute temperature (K).
For Langmuir isotherm, the plot of Ce/qe against Ce will gives straight line. The Langmuir constants will be calculated form the linear plot. For Freundlich isotherm, the value of Kf and 1/n were obtained from the intercept and slope of linear plot in the plot of log
qe against log
Ce respectively. The value of n varies with the heterogeneity of adsorbent. The value of n must be less than 108. When the value 1/n is closer to 0, it means that the adsorbent is becoming more heterogeneous.80 For Temkin isotherm, value of KT and B1 able to obtain through the plot of qe against ln
Ce. All the calculated value of constant was stated in Table 9.
Table 9 Isotherm constant parameter and correlation coefficients calculated for adsorption study
Isotherm |
Parameters |
Adsorbent |
Fe/AC |
Zeolite-Fe/AC |
Langmuir |
Qmax |
19.2 |
24.1 |
KL |
1.2 |
0.36 |
R2 |
0.9987 |
0.9924 |
RL |
0.01 |
0.03 |
Freundlich |
1/n |
0.2 |
0.3 |
KF |
11.8 |
10.4 |
R2 |
0.9508 |
0.9841 |
Temkin |
B1 |
2.7 |
4.4 |
KT |
69.6 |
7.1 |
R2 |
0.9709 |
0.9766 |
Based on the result summarized in the Tables 9 and S7,† the Langmuir isotherm model is fitted the best for both adsorbent, Fe/AC and zeolite-Fe/AC with the highest value of R2 of 0.9987 and 0.9924, respectively. This result proves that the formation of a monolayer adsorption at the surface of the PKS adsorbent.79 Furthermore, it also indicate that the active sites were homogeneously distributed on the adsorbents surface.81 The value of RL obtained in this study are between 0 to 1 for both adsorbents, thus it further explained that Langmuir isotherm is a favorable model to explain the adsorption of POME.82 Besides, the maximum adsorption capacity Qmax of zeolite-Fe/AC (24.1) is higher than Fe/AC (19.2). This shows that the present of zeolite able to increase the surface area of the adsorbent as more amount of an adsorbate loadable on zeolite-Fe/AC. This could be explained as the specific surface area of the adsorbent and affinity of the adsorbent can be expressed by the adsorption isotherm study and maximum adsorption capacity.83,84 Many other published research also reported that Langmuir isotherm is more favorable for COD and color removal by using bio adsorbent6,9,85–88
3.7 Plausible adsorption mechanism
Fig. 5 presents the plausible adsorption mechanisms with the aids of oxidants for zeolite-Fe/AC adsorbent for biological POME by considering initial solution pH, as well as FTIR analysis. In this study, the pH of the POME was found to be acidic conditions (pH 3). The palm oil mill effluent (POME) contains high chemical oxygen demand (COD) and biological oxygen demand (BOD), oil and grease, suspended solids, ammonia–nitrogen, heavy metal concentration and high content of degradable organic matter.89–91 The adsorption behavior of pollutants present in the POME and zeolite-Fe/AC adsorbent is summarized in Fig. 5. Besides, the previously discussed FTIR analysis also clearly explain the deformation and stretching vibrations of the functional group after the adsorption of pollutants. This further describe that the possible mechanism of pollutants adsorption onto zeolite-Fe/AC could be through electrostatic interaction, hydrogen bonding and n–π interactions between the functional group of POME and surface of zeolite-Fe/AC adsorbent.92,93 The possible bond formation mechanism between organic matters and heavy metals with functional group of zeolite-Fe/AC adsorbent has shown in Fig. 5(c)–(e). The proposed mechanism that reported is in line with works reported by previous researchers, where adsorption mechanism could be through three different processes.87,94
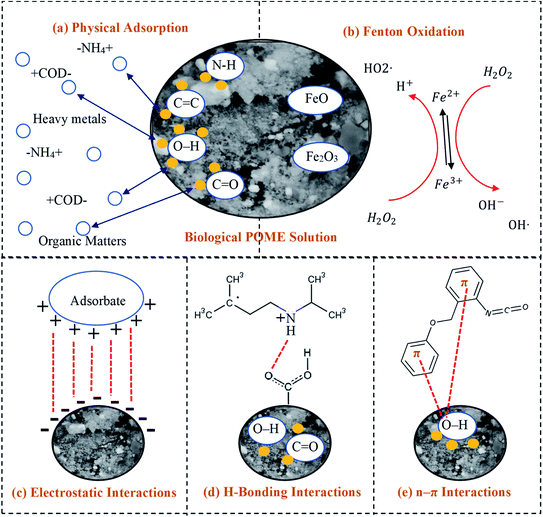 |
| Fig. 5 Proposed adsorption mechanisms of adsorption process with the aids of oxidants: (a) physical adsorption, (b) Fenton oxidation, (c) electrostatic interactions, (d) H-bonding interactions and (e) n–π interactions. | |
Fig. 5(a) and (b) shows that there might be physical adsorption and Fenton oxidation happen simultaneously in this study. The present of iron oxide in the adsorbent will react with the oxidants (H2O2) in the reaction and it will speed up the whole reaction of adsorption process. This could be supported by the removal rate (>80%) within a short reaction time (4 min). Wei and others (2020) also reported that adsorption and oxidation can happened simultaneous with the present of oxidants.95 The present of iron oxide and oxidants can greatly improve the reaction time in the reaction.96,97
3.8 Reusability of adsorbents
The stability study of the developed adsorbent was evaluated over five cycles. The ability of an adsorbent to be used many times without losing its adsorbent capacity is an important consideration in industrial application.98 S8† and Table 10 depicts the colour removal by using the Fe/AC and zeolite-Fe/AC adsorbents over five consecutive cycles. As shown in the results, for Fe/AC, the colour removal efficiency recorded at 80.5% from 87.1% after the 5th cycle. While for zeolite-Fe/AC only shows a slight decrease from 83.9% to 81.0%. Zeolite-Fe/AC was able to retain its adsorbent capability over five cycles with a minimal reduction of 2.9%, but Fe/AC had significantly impaired on its adsorbent capacity of 6.6% decrease which corresponds to the study by Pham, Lee and Kim (2016).99 The reason of zeolite-Fe/AC able to maintain most of its adsorbent capability probably due to the addition of zeolite particles had increased its stability of the adsorbent or due to the characteristic of zeolite that had been reported in few articles.62,63,100,101 Zeolite is made up of aluminium, oxygen, and metals like titanium, Tin, Zinc, etc. Hence it had been proven to have a special characteristic where it is able to permit the passage of molecules that below a certain size.48
Table 10 Reusability of Fe/AC and zeolite-Fe/AC
Adsorbent (%) |
Fe/AC |
Zeolite-Fe/AC |
Regeneration 1st cycle |
87.1 |
83.9 |
Regeneration 5th cycle |
80.5 |
81.0 |
Total amount lost |
6.6 |
2.9 |
3.9 Comparison between Fe/AC and zeolite-Fe/AC adsorbents
Table 11 shows the comparison of Fe/AC and zeolite-Fe/AC adsorbents. The result shows that both adsorbents able to achieve >80% colour removal and >65% COD removal within 30 minutes. As can be seen in Table 11, Fe/AC managed to achieve higher colour removal (86.8%) compared to zeolite-Fe/AC (83.1%). However, Fe/AC reported having lower COD removal (65.6%) in comparison to zeolite-Fe/AC (67.2%). On the other hand, zeolite-Fe/AC used lesser adsorbent and H2O2 (4 g L−1; 67.7 mM) compared to Fe/AC (5 g L−1; 84.0 mM). As can be seen in Table 10, zeolite-Fe/AC was able to retain its adsorbent capability over five cycles, with only 2.9% loss compared to Fe/AC. One of the biggest challenges of adsorption is the cost of the adsorbent and regeneration ability of conventional activated carbon.62,98 Therefore the minimal consumption of adsorbent and oxidants, a shorter reaction time of 30 min and reusability capacity show that both zeolite-Fe/AC and Fe/AC developed from palm kernel shell (PKS) is a great alternative of an adsorbent for adsorption process compared to conventional activated carbon. In addition, the presence of oxidant in the process usually creates a lower hydroxyl radical requirement, as a reaction such as Fenton oxidation can occur with the adsorption cycle. As explained by eqn (11) and (12), H2O2 will react with Fe2+ that present in the adsorbent to form OH˙ radical and Fe3+ will react with H2O2 to regenerate Fe2+ with HO2 and H+.102 Based on the studies of Huling (2017) and Y.-T. Chung (2017), it had been proven that oxidants were able to improve adsorption process.103,104 |
Fe2+ + H2O2 → OH˙ + Fe3+ + OH−
| (11) |
|
Fe3+ + H2O2 → HO2˙ + Fe2+ + H+
| (12) |
Table 11 Comparison of Fe/AC and zeolite-Fe/AC adsorbent
Adsorbent |
pH |
Dosage adsorbent (g L−1) |
Dosage H2O2 (mM) |
Reaction time (min) |
Colour removal (%) |
COD removal (%) |
Fe/AC |
3 |
5 |
84.0 |
30 |
86.8 |
65.6 |
Zeolite-Fe/AC |
3 |
4 |
67.7 |
30 |
83.1 |
67.2 |
Ultimately, zeolite-Fe/AC is preferred economically over Fe/AC as less adsorbent and oxidants are needed and the better adsorption capacity. The effect of the operating parameter on the adsorption of zeolite-Fe/AC cycle is discussed in the following section.
3.10 Effect of operational parameters
(a) Effect of pH. The solution pH is an essential parameter in the adsorption process.72 In this experiment, the effect of pH was investigated by varying the pH between 3 to 9. Fig. 6(a) and (b) shows the effect of pH and dosage of adsorbent on the colour and COD removal, respectively. As, when the pH of the solution increase, the surface of adsorbent become more negatively charged and thus increase in the repulsion between the adsorbate and adsorbent. Therefore, this cause the removal efficiency decreases with an increase in pH.105 Hence, it is proven that the adsorption process for POME favors acidic condition in this study.
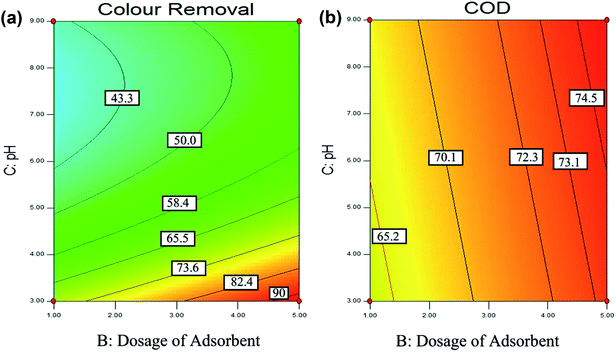 |
| Fig. 6 Contour (2D) plot for (a) colour (b) COD removal of pH and dosage of adsorbent. | |
Fig. 6(a) shows that higher colour removal efficiency (>90%) is achieved at acidic condition, around pH 3. However, when the pH value exceeded 4, it clearly shows that there is a significant decrease in the colour removal efficiency (<65%). Besides, Fig. 6 also shows that the interaction between solution pH and dosage of the adsorbent. It shows that higher colour removal rate (>90%) and COD removal rate (>73%) achieved when the adsorbent is 5 g L−1 and pH 3, however lower colour removal rate (<40%), and COD removal rate (<65%) were observed at the dosage of adsorbent is 1 g L−1 with pH 9.
(b) Effect of adsorbent dosage. By varying the adsorbent dosage between 1 to 5 g L−1, the effect of adsorbent dosage on the colour removal was studied. Fig. 7(a) and (b) show the effective dosage of adsorbent and H2O2 dosage on the colour removal and COD removal of POME. At lower adsorbent dosage, the colour and COD removal efficiency was small as there was insufficient surface area of the adsorbent for the effective decomposition of H2O2. The dosage of adsorbent able to affect the adsorption in terms of availability of surface area for adsorption process as it allows adsorbate to penetrate the adsorption sites easily.6 In conclusion, the dosage of adsorbent will affect the colour removal rate significantly, and it also acts as one of the main characteristics of industrial application.98 A similar result was reported by Mohammed and Chong (2014).6
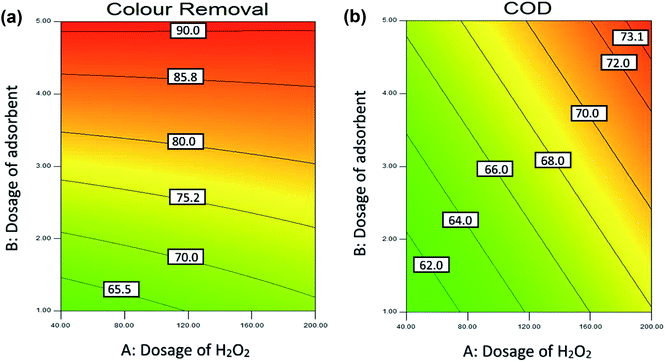 |
| Fig. 7 Contour (2D) plot for (a) colour (b) COD removal of adsorbent dosage and H2O2 dosage. | |
(c) Effect of dosage of H2O2. Fig. 7(a) and (b) shows the effect of adsorbent dosage and H2O2 dosage on the colour removal and COD removal of POME. The dosage of H2O2 act as an essential parameter in COD removal as the production of the hydroxyl radical depends on the oxidants. The previous studies shows that the presence of oxidants such as H2O2 able to increase the rate of adsorption in reaction.103,104 However, the excessive dosage of H2O2 will exert an inhibitory effect as H2O2 molecules will consume OH˙ and form other radicals such as HO2˙ which slow down the adsorption process.106Fig. 7 shows that increase in adsorbent dosage from 1 g L−1 to 5 g L−1 at the acidic condition and 30 minutes reaction time lead to an increase in the colour removal efficiency from 60% to 90% and COD removal efficiency from 60% to 73%. As seen in Fig. 7(a) the best colour removal (>90%) was achieved at the dosage of adsorbent between 4–5 g L−1 and H2O2 between 40 mM to 200 mM.
(d) Effect of reaction time. Fig. 8(a) and (b) shows the effect of reaction time and pH on the colour removal and COD removal of POME. As discussed earlier, the statistical analysis shows that the reaction time is not a significant parameter, as adsorption process often occurs in the beginning of the reaction. This can be explained as in the beginning stage; the adsorption was rapid as there are many active sites on the adsorbent surface. And, with the passage of contact time, the number of actives sites decreased and more difficult to be penetrated by the pollutants as this is due to repulsive forces between the solute molecules in the solid and the bulk liquid phase.107 Besides, adsorbent with a larger surface area required shorter reaction time to achieve the desire adsorption.
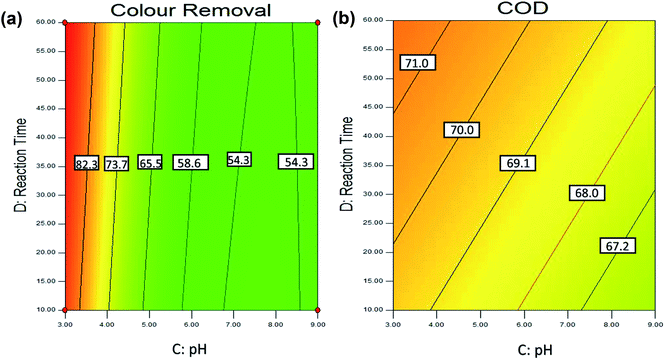 |
| Fig. 8 Contour (2D) plot for (a) colour (b) COD removal of reaction time and pH. | |
Fig. 8(a) and (b) shows that the highest colour removal (>80%) and COD removal (>70%) were obtained within 10 min and 60 min at the acidic condition with 5 g L−1 of adsorbent dosage. Previous studies also reported that the adsorption process usually occurs within 15–30 min reaction time71,72,74
4 Conclusions
This study investigated the possible utilization of palm kernel shell (PKS) for the development of biomass adsorbent. Two types of bio-adsorbent were developed, incorporating PKS with iron oxide and zeolite. The surfaces area, chemical composition, magnetic properties, crystallinity, and stability of the adsorbents were analysed. The adsorption efficiency of newly develop adsorbents was then investigated by using biologically treated POME with the aids of oxidants. Among all the parameters, solution pH was observed to be the most significant parameter affecting the performance of the adsorbent compared to adsorbent dosage, the dosage of H2O2 and reaction time. Both adsorbents show an excellent efficiency on colour removal (>80%) and COD removal (>60%) under the optimum condition which able to meet the standard set by Department of Environment Malaysia (DOE). The experimental data were found to best fit with Langmuir isotherm model compared to Freundlich and Temkin models. Besides, the adsorption kinetic data follow the pseudo-second-order equation for both adsorbents, which reveals that the adsorption of zeolite-Fe/AC and Fe/AC is monolayer adsorption. This study has proven that palm kernel shell able to convert into a low-cost adsorbent for the adsorption of POME in tertiary treatment. This study could provide a dual benefit to the palm oil industry, as its solid waste can be converted into a useful adsorbent and cost savings in wastewater treatment.
List of nomenclature and symbol
HO˙ | Hydroxyl radical |
Fe2+ | Ferrous ion |
k | Reaction rate constant zero order (M/s), first order (1/s), second order (1/Ms) |
mM | Molar (mol L−1) |
min | Minute |
s | Seconds |
T | Temperature (°C) |
t | Time (min) |
g L−1 | Gram per liter |
+COD− | Chemical oxygen demand |
–NH4+ | Ammonium ions |
KL | Constant of Langmuir isotherm |
Kf | Constant of Freundlich isotherm |
KT | Constant of Temkin isotherm |
Conflicts of interest
There are no conflicts to declare.
Acknowledgements
The authors are grateful to University Malaya Research University Grant (RU Grant), GPF060A-2018 and University Malaya Research Grant (UMRG) – Frontier Science (AFR) RG384-17AFR Research Fund for financially supporting this research.
References
- S. Li and G. Chen, J. Cleaner Prod., 2020, 251, 119669 CrossRef.
- M. T. Munir, S. S. Mansouri, I. A. Udugama, S. Baroutian, K. V. Gernaey and B. R. Young, Renewable Sustainable Energy Rev., 2018, 96, 64–75 CrossRef CAS.
- I. Anastopoulos, I. Pashalidis, A. Hosseini-Bandegharaei, D. A. Giannakoudakis, A. Robalds, M. Usman, L. B. Escudero, Y. Zhou, J. C. Colmenares, A. Núñez-Delgado and É. C. Lima, J. Mol. Liq., 2019, 295, 111684 CrossRef CAS.
- S. P. D. Kaman, I. A. W. Tan and L. L. P. Lim, MATEC Web Conf., 2016, 03009 Search PubMed.
- A. A. Indera Luthfi, J. M. Jahim, S. Harun, J. P. Tan and A. W. Mohammad, RSC Adv., 2017, 7, 49480–49489 RSC.
- R. R. Mohammed and M. F. Chong, J. Environ. Manage., 2014, 132, 237–249 CrossRef CAS PubMed.
- N. H. Zainal, A. A. Aziz, J. Idris, N. F. Jalani, R. Mamat, M. F. Ibrahim, M. A. Hassan and S. Abd-Aziz, J. Cleaner Prod., 2018, 182, 830–837 CrossRef CAS.
- A. Asfaram, M. R. Fathi, S. Khodadoust and M. Naraki, Spectrochim. Acta, Part A, 2014, 127, 415–421 CrossRef CAS PubMed.
- B. H. Hameed and A. A. Ahmad, J. Hazard. Mater., 2009, 164, 870–875 CrossRef CAS PubMed.
- J. Qu, X. Tian, Z. Jiang, B. Cao, M. S. Akindolie, Q. Hu, C. Feng, Y. Feng, X. Meng and Y. Zhang, J. Hazard. Mater., 2020, 387, 121718 CrossRef CAS PubMed.
- D. S. D. M. U. Mahidin, Selected Agricultural Indicators, Malaysia, 2019, https://www.dosm.gov.my/v1/index.php?r=column/ctwoByCat%26parent_id=45%26menu_id=Z0VTZGU1UHBUT1VJMFlpaXRRR0xpdz09 Search PubMed.
- M. M. Bello and A. A. Abdul Raman, J. Environ. Manage., 2017, 198, 170–182 CrossRef CAS PubMed.
- C. H. Chung, J. Janaun, V. Semilin and W. S. Balan, MATTER: International Journal of Science and Technology, 2017, 3(2), 276–294 Search PubMed.
- M. O. Saeed, K. A. M. Azizli, M. H. Isa and E. H. Ezechi, Desalin. Water Treat., 2016, 57, 23750–23759 CrossRef CAS.
- M. O. Saeed, K. Azizli, M. H. Isa and M. J. K. Bashir, Journal of Water Process Engineering, 2015, 8, e7–e16 CrossRef.
- A. Fakhru'l-Razi and M. J. M. M. Noor, Water Sci. Technol., 1999, 39, 159–163 CrossRef.
- A. L. Ahmad, S. Sumathi and B. H. Hameed, Chem. Eng. J., 2006, 118, 99–105 CrossRef CAS.
- A. A. L. Zinatizadeh, A. R. Mohamed, M. D. Mashitah, A. Z. Abdullah and M. H. Isa, Biochem. Eng. J., 2007, 35, 226–237 CrossRef CAS.
- N. Razali and N. Z. Kamarulzaman, Mater. Today: Proc., 2020 DOI:10.1016/j.matpr.2020.02.219.
- R. R. Mohammed, M. R. Ketabchi and G. McKay, Chem. Eng. J., 2014, 243, 31–42 CrossRef CAS.
- H. Zangeneh, A. A. Zinatizadeh, S. Zinadini, M. Feyzi and D. W. Bahnemann, React. Funct. Polym., 2018, 127, 139–152 CrossRef CAS.
- A. O. Adeleke, A. A. A. Latiff, A. A. Al-Gheethi and Z. Daud, Chemosphere, 2017, 174, 232–242 CrossRef CAS.
- M. A. Mohammad Razi, A. Al-Gheethi, M. Al-Qaini and A. Yousef, Arab Journal of Basic and Applied Sciences, 2018, 25, 103–110 CrossRef.
- S. S. M. Ezzuldin, S. B. A. Rahim, H. Wan Yussof, O. A. Olalere and O. A. Habeeb, Chem. Data Collect., 2019, 21, 100235 CrossRef.
- F. Zhao, D. Li, B. Cao, M. Liu, K. Chen and Y. Chen, New J. Chem., 2017, 41, 4110–4115 RSC.
- A. C. Lua and Q. Jia, Chem. Eng. J., 2009, 150, 455–461 CrossRef CAS.
- G. Issabayeva, M. K. Aroua and N. M. Sulaiman, Desalination, 2010, 262, 94–98 CrossRef CAS.
- R. Gautam, A. Mudhoo, G. Lofrano and M. Chattopadhyaya, J. Environ. Chem. Eng., 2014, 2, 239–259 CrossRef CAS.
- P. Zhang, D. O'Connor, Y. Wang, L. Jiang, T. Xia, L. Wang, D. C. W. Tsang, Y. S. Ok and D. Hou, J. Hazard. Mater., 2020, 384, 121286 CrossRef CAS.
- Q. Xie, Y. Lin, D. Wu and H. Kong, Fuel, 2017, 203, 411–418 CrossRef CAS.
- I. A. W. Tan, A. L. Ahmad and B. H. Hameed, Colloids Surf., A, 2008, 318, 88–96 CrossRef CAS.
- T. Fazal, A. Razzaq, F. Javed, A. Hafeez, N. Rashid, U. S. Amjad, M. S. Ur Rehman, A. Faisal and F. Rehman, J. Hazard. Mater., 2020, 390, 121623 CrossRef CAS PubMed.
- Z. Wang, M. Gao, X. Li, J. Ning, Z. Zhou and G. Li, Mater. Sci. Eng. Carbon, 2020, 108, 110196 CrossRef CAS PubMed.
- O. Oginni, G. A. Yakaboylu, K. Singh, E. M. Sabolsky, G. Unal-Tosun, D. Jaisi, S. Khanal and A. Shah, J. Environ. Chem. Eng., 2020, 8, 103723 CrossRef CAS.
- J.-X. Yu, B.-H. Li, X.-M. Sun, J. Yuan and R.-a. Chi, J. Hazard. Mater., 2009, 168, 1147–1154 CrossRef CAS PubMed.
- Y.-b. Dong and H. Lin, Trans. Nonferrous Met. Soc. China, 2015, 25, 991–996 CrossRef CAS.
- V. Nejadshafiee and M. R. Islami, Mater. Sci. Eng. Carbon, 2019, 101, 42–52 CrossRef CAS PubMed.
- P. Suresh Kumar, T. Prot, L. Korving, K. J. Keesman, I. Dugulan, M. C. M. van Loosdrecht and G.-J. Witkamp, Chem. Eng. J., 2017, 326, 231–239 CrossRef CAS.
- L. C. A. Oliveira, R. V. R. A. Rios, J. D. Fabris, V. Garg, K. Sapag and R. M. Lago, Carbon, 2002, 40, 2177–2183 CrossRef CAS.
- M. Jain, M. Yadav, T. Kohout, M. Lahtinen, V. K. Garg and M. Sillanpää, Water Resources and Industry, 2018, 20, 54–74 CrossRef.
- W. Yang, Y. Li, S. Shi, H. Chen, Y. Shan and Y. Liu, Fuel, 2019, 256, 115977 CrossRef CAS.
- R. Angelova, E. Baldikova, K. Pospiskova, Z. Maderova, M. Safarikova and I. Safarik, J. Cleaner Prod., 2016, 137, 189–194 CrossRef CAS.
- N. M. Noor, R. Othman, N. M. Mubarak and E. C. Abdullah, J. Taiwan Inst. Chem. Eng., 2017, 78, 168–177 CrossRef CAS.
- O. V. Kharissova, H. V. R. Dias and B. I. Kharisov, RSC Adv., 2015, 5, 6695–6719 RSC.
- J. Hua, J. Environ. Chem. Eng., 2018, 6, 156–168 CrossRef CAS.
- O. B. S. Azmi Aris, K. Suh Kee and Z. Ujang, Malays. J. Civ. Eng., 2008, 20, 12–25 Search PubMed.
- S. M. Al-Jubouri and S. M. Holmes, Journal of Water Process Engineering, 2020, 33, 101059 CrossRef.
- S. Montalvo, C. Huiliñir, R. Borja, E. Sánchez and C. Herrmann, Bioresour. Technol., 2020, 301, 122808 CrossRef CAS PubMed.
- H. Zhang, A. Li, W. Zhang and C. Shuang, J. Colloid Interface Sci., 2016, 468, 128–135 CrossRef CAS PubMed.
- J. Hun Kwak, H. Zhu, J. H. Lee, C. H. Peden and J. Szanyi, Chem. Commun., 2012, 48, 4758–4760 RSC.
- T. Motsi, N. A. Rowson and M. J. H. Simmons, Int. J. Miner. Process., 2009, 92, 42–48 CrossRef CAS.
- S. K. Pitcher, R. C. T. Slade and N. I. Ward, Sci. Total Environ., 2004, 334–335, 161–166 CrossRef CAS.
- E. Erdem, N. Karapinar and R. Donat, J. Colloid Interface Sci., 2004, 280, 309–314 CrossRef CAS PubMed.
- M. A. Shavandi, Z. Haddadian, M. H. S. Ismail, N. Abdullah and Z. Z. Abidin, J. Taiwan Inst. Chem. Eng., 2012, 43, 750–759 CrossRef CAS.
- T. Liu, H. Wang, Z. Zhang and D. Zhao, Chemosphere, 2017, 180, 160–168 CrossRef CAS.
- Ö. Şahin and C. Saka, Bioresour. Technol., 2013, 136, 163–168 CrossRef PubMed.
- C. Tedesco and M. Brunelli, in Comprehensive Supramolecular Chemistry II, ed. J. L. Atwood, Elsevier, Oxford, 2017, pp. 45–73, DOI:10.1016/b978-0-12-409547-2.12489-8.
- M. M. Bello, A. A. A. Raman and A. Asghar, Journal of Water Process Engineering, 2020, 33, 101001 CrossRef.
- C. Saka, J. Anal. Appl. Pyrolysis, 2012, 95, 21–24 CrossRef CAS.
- I. I. Misnon, N. K. M. Zain, R. A. Aziz, B. Vidyadharan and R. Jose, Electrochim. Acta, 2015, 174, 78–86 CrossRef CAS.
- B. D'Cruz, M. Madkour, M. O. Amin and E. Al-Hetlani, Mater. Chem. Phys., 2020, 240, 122109 CrossRef.
- W. A. Khanday, F. Marrakchi, M. Asif and B. H. Hameed, J. Taiwan Inst. Chem. Eng., 2017, 70, 32–41 CrossRef CAS.
- W. A. Khanday, M. Asif and B. H. Hameed, Int. J. Biol. Macromol., 2017, 95, 895–902 CrossRef CAS PubMed.
- Y.-C. Lee, Y.-f. Li, M.-J. Chen, Y.-C. Chen, J. Kuo and S.-L. Lo, Water Res., 2020, 174, 115618 CrossRef CAS PubMed.
- W. P. Cheng, W. Gao, X. Cui, J. H. Ma and R. F. Li, J. Taiwan Inst. Chem. Eng., 2016, 62, 192–198 CrossRef CAS.
- S. Das and S. Mishra, Mater. Chem. Phys., 2020, 245, 122751 CrossRef CAS.
- J. Qu, M. S. Akindolie, Y. Feng, Z. Jiang, G. Zhang, Q. Jiang, F. Deng, B. Cao and Y. Zhang, Chem. Eng. J., 2020, 394, 124915 CrossRef CAS.
- S. Wang, Y. Lu, X.-k. Ouyang, X. X. Liang, D. Yu, L.-Y. Yang and F. Huang, Int. J. Biol. Macromol., 2019, 139, 886–895 CrossRef CAS PubMed.
- H. Javadian, M. Ruiz, T. A. Saleh and A. M. Sastre, J. Mol. Liq., 2020, 112760, DOI:10.1016/j.molliq.2020.112760.
- J. Qu, X. Meng, H. You, X. Ye and Z. Du, Bioresour. Technol., 2017, 241, 1036–1042 CrossRef CAS PubMed.
- J. P. Kushwaha, V. C. Srivastava and I. D. Mall, Bioresour. Technol., 2010, 101, 3474–3483 CrossRef CAS PubMed.
- B. Nandi, A. Goswami and M. Purkait, Appl. Clay Sci., 2009, 42, 583–590 CrossRef CAS.
- J. Qu, X. Meng, Y. Zhang, Q. Meng, Y. Tao, Q. Hu, X. Jiang, H. You and C. A. Shoemaker, J. Hazard. Mater., 2019, 379, 120804 CrossRef CAS PubMed.
- K. E. Chingono, E. Sanganyado, E. Bere and B. Yalala, J. Environ. Manage., 2018, 224, 182–190 CrossRef CAS.
- T. Depci, Chem. Eng. J., 2012, 181–182, 467–478 CrossRef CAS.
- A. Ali, Environmental Nanotechnology, Monitoring & Management, 2017, 7, 57–63 Search PubMed.
- I. Langmuir, J. Am. Chem. Soc., 1918, 40, 1361–1403 CrossRef CAS.
- L. E. Swearingen and B. N. Dickinson, J. Phys. Chem., 1931, 36, 534–545 CrossRef.
- G. D. Akpen, M. I. Aho and M. H. Mamwan, Global J. Pure Appl. Sci., 2018, 24, 61–67 CrossRef.
- A. Özhan, Ö. Şahin, M. M. Küçük and C. Saka, Cellulose, 2014, 21, 2457–2467 CrossRef.
- R. R. J. I. Mohammed, International Refereed Journal of Engineering and Science, 2013, 2, 1–11 Search PubMed.
- K. R. Hall, L. C. Eagleton, A. Acrivos and T. Vermeulen, Ind. Eng. Chem. Fundam., 1966, 5, 212–223 CrossRef CAS.
- O. F. Okeola, E. O. Odebunmi and O. M. Ameen, Bull. Chem. Soc. Ethiop., 2012, 26, 171–180 CrossRef CAS.
- S. Wongcharee, V. Aravinthan, L. Erdei and W. Sanongraj, Int. Biodeterior. Biodegrad., 2017, 124, 276–287 CrossRef CAS.
- A. Hasan, S. P. D. Kaman, I. A. W. Tan, L. L. P. Lim, A. A. Khan, M. A. Mannan, C. N. Hipolito, N. Mohamed Sutan, A.-K. H. Othman, M. R. Kabit and N. Abdul Wahab, MATEC Web of Conferences, 2016, vol. 87 Search PubMed.
- M. B. Desta, J. Thermodyn., 2013, 2013, 375830 Search PubMed.
- H. N. Tran, S.-J. You, T. V. Nguyen and H.-P. Chao, Chem. Eng. Commun., 2017, 204, 1020–1036 CrossRef CAS.
- P. Naderi, M. Shirani, A. Semnani and A. Goli, Ecotoxicol. Environ. Saf., 2018, 163, 372–381 CrossRef CAS PubMed.
- M. Khemkhao, S. Techkarnjanaruk and C. Phalakornkule, Bioresour. Technol., 2015, 177, 17–27 CrossRef CAS PubMed.
- A. Adeleke, A. Latiff, R. M. S. Radin Mohamed, Z. Daud, N. Ismail, A. Ahsan, N. A. Ab Aziz, A. Al-Gheethi, V. Kumar, A. Fadilat and N. Apandi, 2019, DOI:10.1016/b978-0-12-813902-8.00001-0, 1–33.
- L. Okwute and I. Nnennaya, Afr. J. Agric. Res., 2007, 2, 656–662 Search PubMed.
- V.-P. Dinh, T.-D.-T. Huynh, H. M. Le, V.-D. Nguyen, V.-A. Dao, N. Q. Hung, L. A. Tuyen, S. Lee, J. Yi, T. D. Nguyen and L. V. Tan, RSC Adv., 2019, 9, 25847–25860 RSC.
- W. Luo, Z. Bai and Y. Zhu, RSC Adv., 2018, 8, 13370–13387 RSC.
- D. Allouss, Y. Essamlali, O. Amadine, A. Chakir and M. Zahouily, RSC Adv., 2019, 9, 37858–37869 RSC.
- D. Wei, B. Li, L. Luo, Y. Zheng, L. Huang, J. Zhang, Y. Yang and H. Huang, J. Hazard. Mater., 2020, 391, 122057 CrossRef CAS PubMed.
- J. Liang, S. Zhang, M. Ye, J. Huang, X. Yang, S. Li, S. Huang and S. Sun, Chem. Eng. J., 2020, 380, 122499 CrossRef CAS.
- A. Adeleke, A. Latiff, R. M. S. Radin Mohamed, Z. Daud, N. Ismail, A. Ahsan, N. A. Ab Aziz, A. Al-Gheethi, V. Kumar, A. Fadilat and N. Apandi, 2019, pp. 1–33, DOI:10.1016/b978-0-12-813902-8.00001-0.
- N. L. M. Tri, P. Q. Thang, L. Van Tan, P. T. Huong, J. Kim, N. M. Viet, N. M. Phuong and T. M. Al Tahtamouni, Journal of Water Process Engineering, 2020, 33, 101070 CrossRef.
- T.-H. Pham, B.-K. Lee and J. Kim, Process Saf. Environ. Prot., 2016, 104, 314–322 CrossRef CAS.
- M. Wang, R. Xie, Y. Chen, X. Pu, W. Jiang and L. Yao, Bioresour. Technol., 2018, 268, 726–732 CrossRef CAS.
- I. Khalil, K. Thomas, H. Jabraoui, P. Bazin and F. Maugé, J. Hazard. Mater., 2020, 384, 121397 CrossRef PubMed.
- D. Gamaralalage, O. Sawai and T. Nunoura, J. Hazard. Mater., 2019, 364, 791–799 CrossRef CAS PubMed.
- J. R. Huling, S. G. Huling and R. Ludwig, Water Res., 2017, 123, 183–191 CrossRef CAS PubMed.
- Y.-T. Chung, C.-K. Wang, K.-S. Wang, S.-Y. Huang and S.-H. Chang, J. Taiwan Inst. Chem. Eng., 2017, 80, 747–753 CrossRef CAS.
- K. S. Padmavathy, G. Madhu and P. V. Haseena, Procedia Technology, 2016, 24, 585–594 CrossRef.
- S. P. V. Kumar, C. Thakur and P. Ghosh, J. Environ. Chem. Eng., 2019, 7, 102859 CrossRef.
- V. C. Srivastava, I. D. Mall and I. M. Mishra, Colloids Surf., A, 2005, 260, 17–28 CrossRef CAS.
Footnote |
† Electronic supplementary information (ESI) available. See DOI: 10.1039/d0ra03307c |
|
This journal is © The Royal Society of Chemistry 2020 |