DOI:
10.1039/D0RA03253K
(Paper)
RSC Adv., 2020,
10, 22606-22615
Hierarchical Co(OH)2@NiMoS4 nanocomposite on carbon cloth as electrode for high-performance asymmetric supercapacitors†
Received
11th April 2020
, Accepted 8th June 2020
First published on 12th June 2020
Abstract
Hierarchical Co(OH)2@NiMoS4 nanocomposites were successfully prepared on a carbon cloth by using a simple two-step hydrothermal method coupled with a room-temperature vulcanization method. The resulting nanocomposites were composed of large-scale uniform Co(OH)2 nanowires fully covered with ultrathin vertical NiMoS4 nanoflakes. Because of the synergetic effect between Co(OH)2 and NiMoS4, the nanocomposites exhibited good electrochemical performance as a supercapacitor electrode. In particular, a specific capacity of 2229 F g−1 was achieved at a current density of 1 A g−1. In addition, an asymmetrical supercapacitor fabricated using activated carbon as the negative electrode and the as-synthesised nanocomposite as the positive electrode exhibited a maximum energy density of 59.5 W h kg−1 at a power density of 1 kW h kg−1 and excellent cycling stability (100% capacitance retention after 5000 cycles). These results indicate that the hierarchical Co(OH)2@NiMoS4 nanocomposite has great potential for practical application in high-performance energy storage devices.
Introduction
Supercapacitors, a new type of energy storage device, exhibit higher power and energy densities and better cycling stability compared with traditional energy storage devices and thus have wide applications in portable electronic equipment, electric vehicles, and high-power supplies.1,2 The design and synthesis of novel electrode materials to achieve a higher energy density is a key aspect in extending the application scope of supercapacitors.3–5 Therefore, in recent years, many kinds of electrode materials such as hydroxides, sulphides, and transition metal oxides have been investigated for application as supercapacitor electrodes.6,7 Among these, sulphides (e.g., Co3S4, NiS2, and MoS2)8–10 have shown superior performance in terms of electron transmission capacity, electronegativity, and mechanical and thermal stability, and offer a more flexible structure and better cycling stability and rate capacity compared with conventional energy storage materials.11,12 In particular, ternary transition metal sulphides have attracted widespread attention as they can facilitate rich redox reactions and have high electrical conductivity and are considered promising candidates for energy storage materials.13 Chen et al.14 successfully prepared NiCo2S4 urchin-like nanostructures using nickel sulphide and cobalt bases, which delivered a specific capacitance of 1149 F g−1 at a current density of 1 A g−1. Fan et al.15 synthesised a three-dimensional NiCo2S4-rGO hierarchical porous structure by the hydrothermal method and achieved a high specific capacitance of 1107 F g−1 at a current density of 1 A g−1 that decreased by only 10% after 5000 cycles. Du et al.16 synthesized a NiMoS4-A compound by a chemical coprecipitation method and obtained a high specific capacity of 313 C g−1 at 1 A g−1 and a high energy density of 35 W h kg−1 at a power density of 400 W kg−1.
These studies demonstrate that composite electrode materials can further increase the rate capacity and cycling stability because of their structural advantages. Sulphide-based composite materials17 such as hydroxide and sulphide composites,18 ternary sulphide and metal sulphide composites,19 and a series of other multi-component nanomaterials20 have been explored. Co(OH)2 is a suitable candidate for electrode materials because of its unique layered structure, abundance, environmental benignity, and high theoretical capacitance.21,22 Xu's group successfully prepared a three-dimensional Ni–Co–S/Co(OH)2 nanocomposite using Co(OH)2 as a precursor and achieved a high specific capacitance of 1560.8 F g−1 and an energy density of 48.8 W h Kg−1 at a power density of 800 W kg−1, which indicate its superior performance as a supercapacitor.18 Nevertheless, studies on transition metal sulphides/hydroxides as supercapacitor electrodes are still rare, and further studies are required to improve the performance of supercapacitors. In this study, a new composite electrode material, Co(OH)2 nanowires@NiMoS4 nanoflakes, with a heterogeneous structure was successfully prepared on a carbon cloth by a simple two-step hydrothermal method coupled with a room-temperature vulcanization method. This unique hierarchical structure increased the number of active sites and facilitated the transport of a large number of electrolyte ions.23,24 Moreover, because of the low electronegativity of sulphur ions, the electrical conductivity of the electrode material increased. And, the incorporated Mo element could further decrease the charge transfer resistance of the electrodes, thus leading to an increased power density. An asymmetric supercapacitor (ASC) fabricated using activated carbon (AC) as the negative electrode and the as-synthesised nanocomposite as the positive electrode exhibited a good electrochemical performance, with a high energy density of 59.5 W h Kg−1 at a power density of 1000 W Kg−1 and an excellent cycling stability with 100% capacitance retention after 5000 cycles. The originality and novelty of this work is to introduce a new strategy in structural engineering for developing highly efficient energy storage devices.
Experimental section
Growth of Co(OH)2 nanowires on carbon cloth
All the reagents were of analytical grade and used without further purification. A piece of carbon cloth 3 × 3 cm2 in size was thoroughly ultrasonically washed in absolute ethanol and deionised water for 15 min, and then dried at room temperature. The typical synthesis process was as follows: 0.873 g of Co(NO3)2·6H2O (3 mmol), 0.09 g of CO(NH2)2 (1.5 mmol), and 0.21 g of C6H12N4 (1.5 mmol) were dissolved in 30 mL of deionised water by continuous vigorous stirring to obtain a clear solution. Next, the washed carbon cloth and solution were transferred to a 50 mL Teflon-lined stainless autoclave that was then sealed and maintained at 120 °C for t1 = 4 h. After cooling to room temperature naturally, the product was taken out and washed in ethanol and deionised water several times. Finally, the sample was dried at 60 °C for 12 h to obtain Co(OH)2 nanowires on carbon cloth.
Growth of NiMoS4 nanoflakes on Co(OH)2 nanowires
First, 0.872 g of Ni(NO3)2·6H2O (3 mmol), 1.936 g of Na2MoO4·2H2O (8 mmol), 0.09 g of CO(NH2)2 (1.5 mmol), and 0.21 g of C6H12N4 (1.5 mmol) were dissolved in 30 mL of deionised water by magnetic stirring to obtain a clear solution. The solution and the carbon cloth with the Co(OH)2 nanowires were transferred to a 50 mL Teflon-lined stainless autoclave and heated at 120 °C for different periods (t2 = 2, 4, and 6 h) to determine the optimum reaction conditions. Afterwards, the carbon cloth was taken out, washed several times with deionised water and ethanol, and dried at 60 °C for 12 h to obtain a Co(OH)2/Ni–Mo oxy-hydroxide (denoted as NiMo–O(OH)) composite precursor on carbon cloth. The precursor was then immersed in a 33 wt% Na2S solution for t3 = 12 h for in situ vulcanization. Finally, the product was taken out, washed in ethanol and deionised water several times, and dried at 60 °C for 12 h to obtain Co(OH)2@NiMoS4 nanocomposite on carbon cloth. The mass loadings of the Co(OH)2@NiMoS4 composite on carbon cloth after 2, 4, and 6 h were 3.1, 3.5, and 4.1 mg cm−2, respectively. For comparison, CoS2 nanowires were prepared on a carbon cloth by the in situ vulcanization of Co(OH)2 nanowires on carbon cloth.
Material characterisation
The microstructures and morphologies of the Co(OH)2@NiMoS4 nanocomposites were analysed by field emission scanning electron microscopy (FESEM) and energy dispersive X-ray spectroscopy (EDS) using a field emission scanning electron microscope equipped with an energy dispersive X-ray spectrometer (JEOL JSM-7100F), and high-resolution transmission electron microscopy (HRTEM, FEI Tecnai G20) equipped with selected area electron diffraction (SAED) patterns. The elemental compositions of the as-synthesised products were determined by X-ray photoelectron spectroscopy (XPS, Thermo Scientific ESCALAB250).
Electrochemical measurements
The electrochemical tests were carried out at room temperature using a CHI 660E three-electrode system electrochemical workstation (CH Instruments, China). The as-synthesized nanocomposite (1 cm × 1 cm), a Pt wire electrode, and an Ag/AgCl electrode were used as the working, counter, and reference electrodes, respectively. A 3.0 M KOH solution was used as the electrolyte. Cyclic voltammetry (CV), galvanostatic charge–discharge (GCD) tests, and electrochemical impedance spectroscopy were performed. The specific capacitance of the sample was calculated by the following equation:25 |
 | (1) |
where Cm, I, Δt, ΔV, and m represent the specific capacitance (F g−1), discharge current (A), discharge time (s), potential window (V), and total active material mass (g), respectively.
Fabrication of asymmetric supercapacitor (ASC)
Asymmetric supercapacitors (ASCs) were fabricated using the Co(OH)2@NiMoS4 nanocomposites on carbon cloth as the positive electrode and AC as the negative electrode. The AC electrode was prepared by mixing AC, conductive carbon black, and polytetrafluoroethylene emulsion in a weight ratio of 80
:
10
:
10 with stirring to form a homogeneous slurry. The slurry was then painted onto a washed Ni foam (1 cm × 1 cm) and dried at 60 °C for 10 h in a vacuum oven. Finally, the resultant electrodes were pressed at 10 MPa to form a sheet electrode. In a two-electrode system, CV and GCD tests were performed with 3 M KOH as the electrolyte. The specific capacitance (CS), energy density (E), and power density (P) of the ASCs were calculated by the following equations:26 |
 | (2) |
|
 | (3) |
|
 | (4) |
|
 | (5) |
Here, m± and C± represent the active material mass and specific capacitance of the positive (or negative) electrode, respectively, ΔV is the potential window, I is the discharge current (A), Δt is the discharge time (s), and M is the total mass (g) of the two electrodes.
Results and discussion
Material characterisation
The synthesis process of the Co(OH)2@NiMoS4 nanocomposites is illustrated in Fig. 1a. In the first step, Co(OH)2 nanowires were grown on a carbon cloth by a hydrothermal method. The SEM image of the Co(OH)2 nanowires grown on a carbon cloth is shown in Fig. 1b. As can be seen, a large number of Co(OH)2 nanowires uniformly cover the entire surface of the carbon fibres forming clusters of a slender interlaced structure. The average length of these nanowires is 10 μm or less. The corresponding high-magnification SEM image (Fig. 1c) shows that each nanowire has a uniform diameter and a smooth surface. The diameter of the nanowires is approximately 40–100 nm. More SEM images of the Co(OH)2 nanowires can be seen in Fig. S1 (ESI†). The chemical composition of the nanowires was determined by EDS. Combining these findings with previous reports leads to the deduction that the strong Co and O peaks in the corresponding EDS spectrum (Fig. 1d) indicate the formation of Co(OH)2. The as-grown Co(OH)2 nanowires on the carbon fibres formed a three-dimensional brush-like structure that increased the specific surface area of the active material. In the second step, Ni–Mo oxy-hydroxide nanoflakes were grown on the Co(OH)2 nanowires. The SEM image of the Ni–Mo oxy-hydroxide nanoflakes synthesized by 4 h of hydrothermal reaction is shown in Fig. 1e. As can be seen, many wire-like structures cover the entire surface of the carbon fibres, as in the case of Co(OH)2 nanowires shown in Fig. 1b. The average length of these wire-like structures is 10 μm. The high-magnification SEM image (Fig. 1f) shows that the wire-like structure is composed of highly dense and uniform nanoflakes distributed on the Co(OH)2 nanowires. Hence, the diameter of the composite wire-like structure is 200–300 nm. The nanoflakes are a few nanometres in thickness and tens of nanometres in lateral size. These small flakes are interlaced and form a three-dimensional structure with open spaces, offering a large specific surface area.19 The corresponding EDS spectrum (Fig. 1g) shows that the product obtained in the second step is composed of O, Mo, Co, and Ni. According to our previous work,5 these nanoflakes should be Ni–Mo oxy-hydroxide.
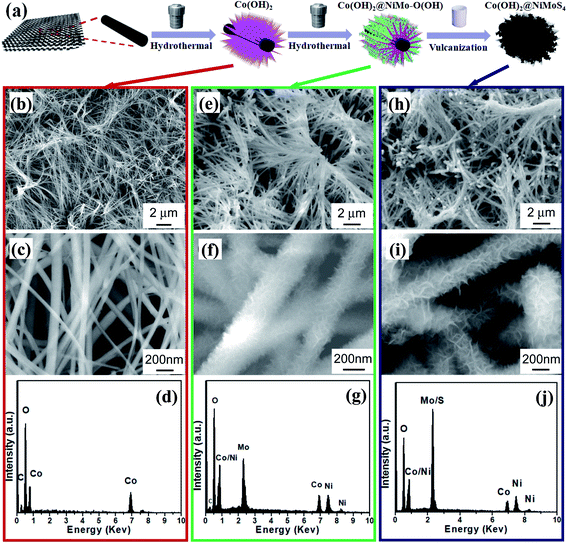 |
| Fig. 1 (a) Schematic of the synthesis process of Co(OH)2@NiMoS4 nanocomposites; (b) low- and (c) high-magnification SEM images of Co(OH)2 nanowires grown on carbon cloth and (d) EDS spectrum of Co(OH)2 nanowires; (e) low- and (f) high-magnification SEM images of Ni–Mo oxy-hydroxide nanoflakes grown on Co(OH)2 nanowires for 4 h and (g) EDS spectrum of Ni–Mo oxy-hydroxide nanoflakes; (h) low- and (i) high-magnification SEM images of Co(OH)2@NiMoS4 nanocomposite and (j) EDS spectrum of Co(OH)2@NiMoS4 nanocomposite. | |
In the third step, the nanoflake precursors were vulcanized in situ at room temperature. After vulcanization, the overall appearance of the final product was similar to that of the sample obtained in the second step, as shown in Fig. 1h and i. The average length of the wire-like structures in the final product remained the same (approximately 10 μm). However, the lateral size and thickness of the nanoflakes grown on the Co(OH)2 nanowires increased slightly, which indicates a tiny increase of surface area after sulfuration. Although the major peaks of S and Mo overlap in the EDS spectrum (shown in Fig. 1j), the increase in peak intensity indicates a change in material composition after vulcanization. The above speculation can be confirmed by the corresponding XRD analysis illustrated in Fig. S2 (ESI†). It indicates the formation of Co(OH)2 in the first step, and the growth of Ni(OH)2 and MoO2 composites in the second step. After vulcanization, the XRD peaks corresponding to Co(OH)2 still remain, showing the preserve of Co(OH)2 nanowires. On the contrary, all of the peaks corresponding to Ni(OH)2 and MoO2 has disappeared to be replaced by some new peaks, which can be attributed to the formation of NiMoS4.27 The weight ratio of NiMoS4 to Co(OH)2 for the final product (t2 = 4 h) is about 4
:
5 by precision weighing method.
The spatial distributions of specific elements in the composite were determined by EDS elemental mapping analysis. As can be seen in Fig. 2, Co and O are distributed primarily in the core region, while Mo, Ni, and S are uniformly distributed in the entire nanocomposite. The elemental composition and valence states of the elements near the surface of the Co(OH)2@NiMoS4 nanocomposites were determined by XPS. The XPS survey spectrum in Fig. 3a shows the presence of Ni, Co, Mo, O, and S in the sample, consistent with the EDS elemental mapping results. The C peak originates from the carbon fibres used as the substrate. The high-resolution spectra were fitted using a Gaussian curve-fitting method. The Co 2p spectrum (Fig. 3b) deconvoluted into two peaks at binding energies of 781.7 (2p3/2) and 797.5 eV (2p1/2) that are attributed to Co2+. In addition, two shake-up satellites peaks (labelled as “Sat.”) appeared at 785.6 and 803.2 eV.28 The O 1s XPS spectrum (Fig. 3c) deconvoluted into two peaks labelled as O1 and O2. The O1 peak at 531.3 eV is generally ascribed to OH− groups, which indicates the formation of hydroxide.29 Thus, the nanowires obtained in the first synthetic step was confirmed to be Co(OH)2. The O2 peak at 532.5 eV is attributed to the multiplicity of adsorbed water at or near the surface.28 The Ni 2p spectrum (Fig. 3d), deconvoluted into two main peaks at binding energies of 856.1 (2p3/2) and 873.9 eV (2p1/2) that are attributed to Ni2+, while the corresponding satellite peaks at 861.8 and 880.0 eV are ascribed to Ni3+ or Ni4+ active species.30 The high-resolution Mo 3d spectrum (Fig. 3e) overlaps with the S 2s region and deconvoluted into two peaks at 232.1 and 235.1 eV corresponding to Mo 3d5/2 and 3d3/2, respectively. The two peaks are separated by a splitting width of approximately 3.1 eV, which is characteristic of the Mo6+ oxidation state.31 The S 2p spectrum (Fig. 3f) deconvoluted into peaks at 161.7 (2p3/2) and 163.3 eV (2p1/2), which confirm the presence of terminal S2− ions. The peaks at 168.6 and 169.7 eV originate from S–Ni and S–Mo, respectively.32 The XPS analysis results demonstrate the presence of Co2+, Ni2+, and Mo6+ in the final products, indicating the successful synthesis of Co(OH)2@NiMoS4 nanocomposites.
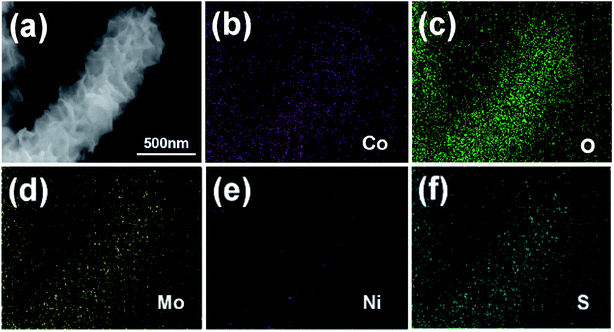 |
| Fig. 2 (a) SEM image of Co(OH)2@NiMoS4 nanocomposite, and (b)–(f) element mapping images of Co, O, Mo, Ni, and S. | |
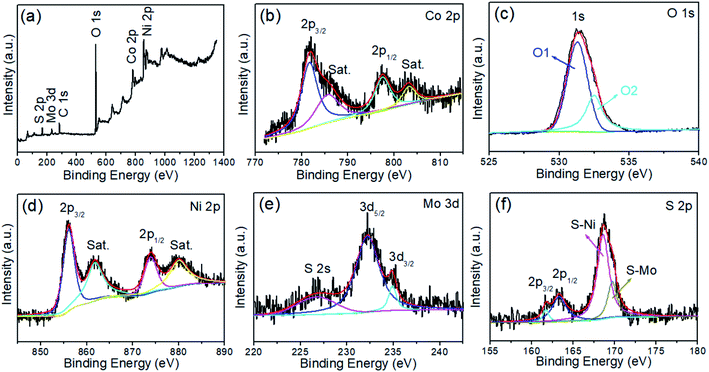 |
| Fig. 3 (a) XPS survey spectrum, and high-resolution XPS spectra of (b) Co 2p, (c) O 1s (d) Ni 2p, (e) Mo 3d, (f) S 2p of Co(OH)2@NiMoS4 nanocomposite. The satellite peaks are denoted as “Sat.”. | |
The TEM image in Fig. 4a shows that the diameter of a single Co(OH)2@NiMoS4 nanocomposite structure is approximately 400 nm. Moreover, many nanoflakes are attached to the central axis, forming a section of wire-like structure; this is consistent with the SEM observations. The magnified TEM image in Fig. 4b shows that these nanoflakes are interconnected and form a highly porous network structure that can considerably shorten the electron and ion transport pathways, resulting in a superior electrochemical reaction activity. Fig. 4c shows that the thickness of these nanoflakes is approximately 4–10 nm. The HRTEM image in Fig. 4d shows lattice fringes with interplanar spacings of 0.21 nm and 0.15 nm corresponding to the (220) and (110) crystal planes of NiS2 and MoS2, respectively. This reveals that NiMoS4 contains NiS2 and MoS2.14 The SAED pattern showing well-defined rings (inset of Fig. 4d) indicates the polycrystalline nature of the CoMoS4 nanocomposites.
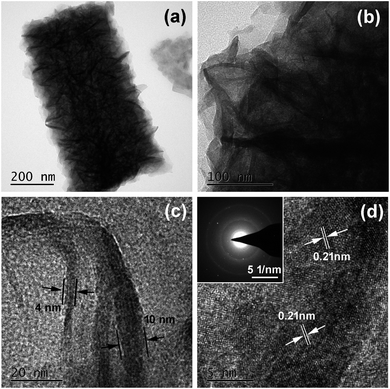 |
| Fig. 4 (a)–(c) TEM images and (d) HRTEM image of Co(OH)2@NiMoS4 nanocomposite. The inset of (d) shows the corresponding SAED pattern. | |
Electrochemical properties of the nanocomposites
The electrochemical performance of Co(OH)2, CoS2, Co(OH)2@NiMo–O(OH), and Co(OH)2@NiMoS4 synthesized for different reaction times in the second stage (t2 = 2, 4, and 6 h) were evaluated by CV performed at a scan rate of 30 mV s−1 using a 3.0 M KOH solution as the electrolyte in a three-electrode system. The appearance of strong redox peaks in the CV curves (Fig. 5a) indicates a good pseudo-capacitive behaviour originating from the faradaic redox reactions.33 Notably, among all the synthesized electrode materials, the Co(OH)2@NiMoS4 electrode sample prepared for t2 = 4 h (Co(OH)2@NiMoS4-4 h) exhibits the largest hysteresis loop area in the CV curve. The higher specific capacitance of the Co(OH)2@NiMoS4 electrodes can be attributed to their high electrical conductivity, large specific surface area, and the synergistic effect of the nanocomposite components.28 The effect of scan rate on the performance of the Co(OH)2@NiMoS4-4 h nanocomposite used as the working electrode is shown in Fig. 5b. With an increase in scan rate from 10 to 50 mV s−1, the cathodic and anodic peaks shifted towards more negative and positive potentials; however, the shapes of the CV curves did not change significantly. This indicates the good rate capability and low polarisation of the nanocomposite.34 Furthermore, as determined by calculations, both the oxidation and reduction peak currents are proportional to the square root of the scanning rate, and diffusion is the dominant electrochemical process.5
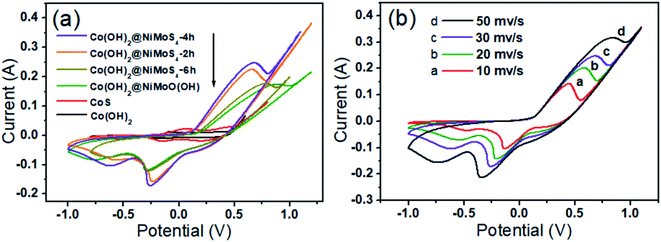 |
| Fig. 5 (a) CV curves of Co(OH)2, CoS, Co(OH)2@NiMo–O(OH), and Co(OH)2@NiMoS4 prepared for different reaction times in the second stage (t2 = 2, 4, and 6 h) measured at a scan rate of 30 mV s−1. (b) CV curves of Co(OH)2@NiMoS4-4 h electrode measured at different scanning rates. | |
Fig. 6a shows the GCD curves of the Co(OH)2@NiMoS4-4 h nanocomposite electrode measured at different current densities (1, 2, 3, 5, and 10 A g−1) in the potential range of −0.1 V to 0.37 V. The appearance of two pronounced potential plateaus during the charge–discharge cycles indicates the pseudo-capacitive behaviour of the reversible faradaic capacitance reaction. In addition, with an increase in current density, the discharge time gradually decreased, which indicates the high utilization rate of the composite electrode under a low current density.35 The specific capacitance of the Co(OH)2@NiMoS4-4 h electrode at different current densities were calculated by eqn (1). The Co(OH)2@NiMoS4-4 h electrode delivered specific capacitances of 2229, 2094, 1989, 1797, and 1436 F g−1 (6.69, 6.28, 5.97, 5.39, and 4.31 F cm−2) at current densities of 1, 2, 3, 5, and 10 A g−1 (corresponding to 3, 6, 9, 15 and 30 mA cm−2), respectively. Moreover, at 5 and 10 A g−1, the electrode retained 81% and 64% of the specific capacitance delivered at 1 A g−1, respectively, which demonstrates its good rate performance. Accordingly, the specific capacitances of Co(OH)2, CoS2, Co(OH)2@NiMo–O(OH), and Co(OH)2@NiMoS4 prepared for different hydrothermal times (t2 = 2 h and 6 h) were measured at different current densities. With an increase in current density from 1 to 10 A g−1, the specific capacitance retentions of were 72, 57, 37, 59, and 47%, respectively (Fig. 6b). The Co(OH)2@NiMoS4-4 h composite electrode retains the highest specific capacitance and has higher capacitance retention throughout. Furthermore, the cycling stability of the electrode materials was evaluated by performing charge–discharge cycling tests for 2000 cycles at a constant current density of 10 A g−1. As shown in Fig. 6c, the total capacitance retention of the Co(OH)2@NiMoS4-4 h electrode (approximately 84%) is higher than that of the Co(OH)2@NiMoS4-2 h electrode (approximately 80%) and Co(OH)2@NiMoS4-6 h electrode (approximately 67%). This indicates the excellent electrochemical performance of the Co(OH)2@NiMoS4-4 h nanocomposite. Moreover, the capacitance of the Co(OH)2@NiMoS4-4 h nanocomposite electrode decreased because of the slight increase in charge-transfer resistance during the charge–discharge process, as observed from the Nyquist plots of the nanocomposite after the 1st and 2000th cycles (Fig. 6d).7 However, the diameter of the semicircle in the high-frequency region that corresponds to the charge-transfer resistance at the electrolyte/electrode interface increased slightly after 2000 cycles (the inset of Fig. 6d), which indicates the good stability of the nanocomposite.13
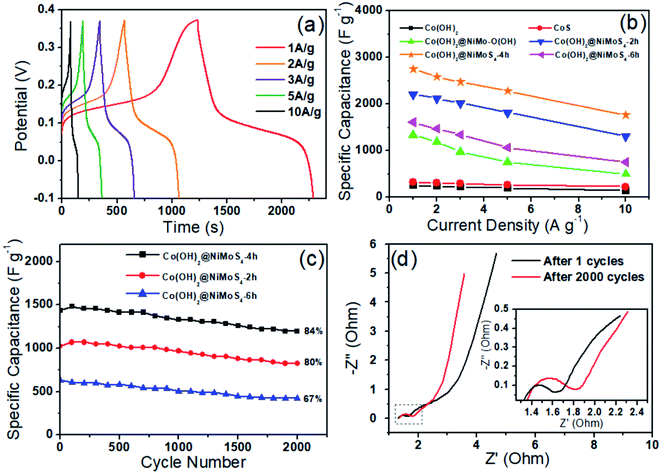 |
| Fig. 6 (a) GCD curves of Co(OH)2@NiMoS4-4 h electrode at different current densities, (b) specific capacitances of Co(OH)2, CoS, Co(OH)2@NiMo–O(OH), and Co(OH)2@NiMoS4 electrodes at different current densities, (c) cycling performance of Co(OH)2@NiMoS4-4 h electrode at a current density of 10 A g−1, and (d) Nyquist plots of Co(OH)2@NiMoS4-4 h after 1st and 2000th cycles. | |
Overall, the Co(OH)2@NiMoS4-4 h composite electrode demonstrated excellent capacitive performance, good cycling stability, and good rate capability, and thus has the potential for application in energy storage devices. The excellent properties of Co(OH)2@NiMoS4 nanocomposites can be attributed to the following factors. First, the interlaced Co(OH)2 nanowires covered with the dense NiMoS4 nanoflakes form as a three-dimensional hierarchical structure that can provide a large surface area and more exposed electroactive sites, thereby shortening the ion diffusion path and increasing the specific capacitance.36 Second, metal sulphides have higher electrochemical activity and electrical conductivity than metal oxides or hydroxides,11,13 while ternary transition metal sulphides offer richer redox reactions and have higher electrical conductivity than binary metal sulphides. Moreover, the interconnected NiMoS4 nanoflakes provide more electron transport pathways and improve the electrical conductivity, thus delivering a higher capacitance. Finally, the Co(OH)2@NiMoS4 composite was grown directly on a carbon cloth, which obviates the necessity of using a polymer binder for electrode preparation and ensures good structural integrity and fast electron transport.28
Properties of the asymmetric supercapacitor (ASC)
To explore the feasibility of using the prepared nanocomposite as a supercapacitor electrode, an ASC was assembled using AC as the negative electrode, Co(OH)2@NiMoS4-4 h as the positive electrode, and 3 M KOH as the electrolyte according to a previously reported method. To determine the optimal cell voltage window for the assembled ASC, CV was performed using a three-electrode system with Co(OH)2@NiMoS4-4 h and AC as the electrodes. As can be seen in Fig. 7a, the CV curve of AC is rectangular in the potential range of −1.0 V to 0.0 V, which indicates that it is an electric double-layer capacitor. The CV of Co(OH)2@NiMoS4-4 h was measured in the potential range of −1.0 V to 1.1 V; thus, the potential window could be extended to 0.0–2.1 V. Fig. 7b shows the CV curves of the assembled ASC at different scanning rates ranging from 10 to 50 mV s−1. The CV curves of the ASC comprising the electric double-layer electrode and the pseudo-capacitive electrode show characteristic shapes. In addition, the shapes of the CV curves changed only slightly, which suggests the good performance of the nanocomposite capacitor.37,38
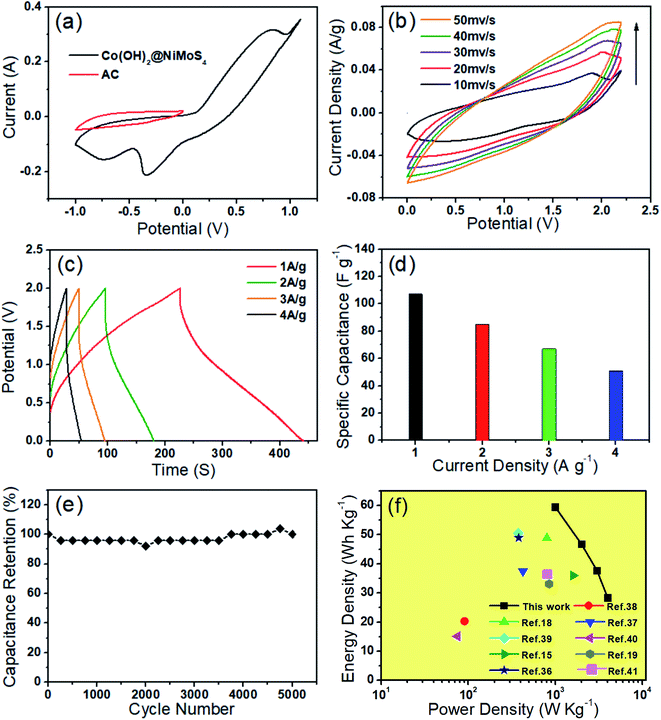 |
| Fig. 7 (a) CV curves of AC and Co(OH)2@NiMoS4-4 h electrode at a scan rate of 30 mV s−1 in a three-electrode system, (b) CV curves of Co(OH)2/NiMoS4-4 h//AC capacitor at different scan rates from 10 to 50 mV s−1, (c) GCD curves of the assembled capacitor at different current densities, (d) mass specific capacitance of the capacitor at different current densities, (e) cycling stability of the capacitor at current density of 4 A g−1 for 5000 cycles, (f) Ragone plots of energy density and power density of our Co(OH)2/NiMoS4-4 h//AC capacitor and comparison with other works. | |
GCD tests were performed for the Co(OH)2@NiMoS4//AC capacitor at different current densities (1, 2, 3, and 4 A g−1) in the potential range of 0.0–2.0 V. The GCD curves are nearly symmetrical and linear, which indicates the excellent capacitive behaviour of the device (Fig. 7c). The specific capacitance of the ASC was calculated by eqn (3). As shown in Fig. 7d, the specific capacitances are 107.4, 84.5, 67.7, and 51.3 F g−1 at current densities of 1, 2, 3, and 4 A g−1, respectively. The cycling stability of the Co(OH)2@NiMoS4//AC capacitor was evaluated by conducting GCD tests for 5000 cycles at a current density of 4 A g−1; the results are shown in Fig. 7e. As can be seen, the capacitance decreased slightly at the initial stage of the GCD test and then increased gradually. The capacitance retention rate was 100% after 5000 cycles; this indicates the excellent cycling stability of the ASC. Fig. 7f shows the Ragone plots of the Co(OH)2@NiMoS4//AC capacitor device calculated by eqn (4) and (5) and those of previously reported capacitors. The Co(OH)2@NiMoS4//AC capacitor device exhibited a maximum energy density of approximately 59.5 W h kg−1 at a power density of 1000 W kg−1 and maintained a substantial energy density of 28.4 W h kg−1 at the power density of 4000 W kg−1. This value is higher than those of previously reported ASCs such as NiCo2S4-rGO (16%)//NCCF-rGO,15 Ni1–Co2–S/Co(OH)2//AC,18 Co3S4/CoMo2S4-rGO//AC,19 AC//Ni–Co–S,36 MoO3/NiMoO4//AC,37 AC/Co(OH)2/Ni foam,38 NF/S–Co3O4@NiCo2S4//AC,39 NiS/MoS2//AC,40 and Mo–CuO-2//AC.41 These exciting results indicate that the hierarchical Co(OH)2@NiMoS4 nanocomposite is a promising material for high-performance electrodes.
Conclusion
Hierarchical Co(OH)2/NiMoS4 nanocomposites were synthesised on a carbon cloth by a two-step hydrothermal method coupled with a vulcanization method. The resulting nanocomposites are composed of large-scale interlaced Co(OH)2 nanowires covered with dense NiMoS4 nanoflakes. As a novel electrode material for pseudo-capacitors, the nanocomposite exhibited excellent electrochemical properties, with a high specific capacitance of 2229 F g−1 at a current density of 1 A g−1. An ASC fabricated using the as-synthesised nanocomposite as the positive electrode and AC as the negative electrode exhibited a maximum energy density of 59.5 W h kg−1 at a power density of 1 kW h kg−1 and excellent cycling stability. These results indicate that the hierarchical Co(OH)2@NiMoS4 nanocomposite has the potential for application in energy storage devices.
Conflicts of interest
There are no conflicts to declare.
Acknowledgements
This work was supported by the Natural Science Foundation Project of CQ CSTC (Grant No. cstc2019jcyj-msxmX0311) and the Fundamental Research Funds for the Central Universities (grant XDJK2018B033 and XDJK2020B055).
References
- J. R. Miller and P. Simon, Materials science–electrochemical capacitors for energy management, Science, 2008, 321, 651–652 CrossRef CAS PubMed.
- P. Simon and Y. Gogotsi, Materials for electrochemical capacitors, Nat. Mater., 2008, 7, 845–854 CrossRef CAS PubMed.
- G. P. Xiong, P. G. He, Z. P. Lyu, T. F. Chen, B. Y. Huang, L. Chen and T. S. Fisher, Bioinspired leaves-on-branchlet hybrid carbon nanostructure for supercapacitors, Nat. Commun., 2018, 9, 790 CrossRef PubMed.
- W. Wen, J. C. Yao, H. Tan and J. M. Wu, Hollow TiN nanotrees derived from a surface induced Kirkendall effect and their application in high-power supercapacitors, J. Mater. Chem. A, 2019, 7, 21378 RSC.
- Y. J. Gu, W. Wen and J. M. Wu, Simple air calcination affords commercial carbon cloth with high areal specific capacitance for symmetrical supercapacitors, J. Mater. Chem. A, 2018, 6, 21078 RSC.
- Z. Yu, L. Tetard, L. Zhai and J. Thomas, Supercapacitor electrode materials: nanostructures from 0 to 3 dimensions, Energy Environ. Sci., 2015, 8, 702–730 RSC.
- C. B. Duan, J. W. Zhao, L. R. Qin, L. J. Yang and Y. C. Zhou, Ternary Ni-Co-Mo oxy-hydroxide nanoflakes grown on carbon cloth for excellent supercapacitor electrodes, Mater. Lett., 2017, 208, 65–68 CrossRef CAS.
- G. Liu, B. Wang, L. Wang, T. Liu, T. Gao and D. Wang, Facile controlled synthesis of a hierarchical porous nanocoral-like Co3S4 electrode for high-performance supercapacitors, RSC Adv., 2016, 6, 54076–54086 RSC.
- D. Kong, C. Cheng, Y. Wang, J. I. Wong, Y. Yang and H. Y. Yang, Three-dimensional Co3O4@C@Ni3S2 sandwich-structured nanoneedle arrays: towards high-performance flexible all-solid-state asymmetric supercapacitors, J. Mater. Chem. A, 2015, 3, 16150–16161 RSC.
- Y. Yang, H. Fei, G. Ruan, C. Xiang and J. M. Tour, Edge-oriented MoS2 nanoporous films as flexible electrodes for hydrogen evolution reactions and supercapacitor devices, Adv. Mater., 2014, 26, 8163–8168 CrossRef CAS PubMed.
- X. Y. Yu and X. W. Lou, Mixed metal sulfides for electrochemical energy storage and conversion, Adv. Energy Mater., 2018, 8, 1701592 CrossRef.
- X. M. Geng, Y. L. Zhang, Y. Han, J. Li, L. Yang, M. Benamara, L. Chen and H. L. Zhu, Two-dimensional water-coupled metallic MoS2 with nanochannels for ultrafast supercapacitors, Nano Lett., 2017, 17, 1825–1832 CrossRef CAS PubMed.
- L. Li, H. Hu, S. Ding, X. Yan and C. Wang, CoNi2S4 nanosheets on nitrogen-doped carbon foam as binder-free and flexible electrodes for high-performance asymmetric supercapacitors, Nanotechnology, 2019, 30, 495404 CrossRef CAS PubMed.
- H. C. Chen, J. J. Jiang, L. Zhang, H. Z. Wan, T. Qi and D. D. Xia, Highly conductive NiCo2S4 urchin-like nanostructures for high-rate pseudocapacitors, Nanoscale, 2013, 5, 8879–8883 RSC.
- Y. M. Fan, Y. C. Liu, X. B. Liu, Y. N. Liu and L. Z. Fan, Hierarchical porous NiCo2S4-rGO composites for high-performance supercapacitors, Electrochim. Acta, 2017, 249, 1–8 CrossRef CAS.
- D. Du, R. Lan, J. Humphreys, W. Xu, K. Xie, H. Wang and S. Tao, Synthesis of NiMoS4 for high-performance hybrid supercapacitors, J. Electrochem. Soc., 2017, 164, A2881–A2888 CrossRef CAS.
- X. F. Wang, Y. F. Zhang, J. Q. Zheng, X. Liu and C. G. Meng, Hydrothermal synthesis of VS4/CNTs composite with petal-shape structures performing a high specific capacity in a large potential range for high-performance symmetric supercapacitors, J. Colloid Interface
Sci., 2019, 554, 191–201 CrossRef CAS PubMed.
- T. H. Xu, G. Y. Li and L. J. Zhao, Ni-Co-S/Co(OH)2 nanocomposite for high energy density all-solid-state asymmetric supercapacitors, Chem. Eng. J., 2018, 336, 602–611 CrossRef CAS.
- X. Yang, H. Sun, P. Zan, L. Zhao and J. Lian, Growth of vertically aligned Co3S4/CoMo2S4 ultrathin nanosheets on reduced graphene oxide as a high-performance supercapacitor electrode, J. Mater. Chem. A, 2016, 4, 18857–18867 RSC.
- F. S. Chen, H. Wang, S. Ji, B. G. Pollet and R. F. Wang, Hierarchical core-shell structured CoNi2S4/Ni3S2@Ni(OH)2 nanosheet arrays as electrode for electrochemical energy storage, J. Alloys Compd., 2019, 785, 684–691 CrossRef CAS.
- T. Zhao, H. Jiang and J. Ma, Surfactant-assisted electrochemical deposition of α-cobalt hydroxide for supercapacitors, J. Power Sources, 2011, 196, 860–864 CrossRef CAS.
- Z. Yu, Z. Cheng, S. R. Majid, Z. Tai, X. Wang and S. Dou, Smart design of free-standing ultrathin Co-Co(OH)2 composite nanoflakes on 3D nickel foam for high-performance electrochemical capacitors, Chem. Commun., 2015, 51, 1689–1692 RSC.
- S. M. Li, H. Jiang, K. Yang, Z. Zhang, S. J. Li, N. H. Luo, Q. H. Liu and R. Wei, Three-dimensional hierarchical graphene/TiO2 composite as highperformance electrode for supercapacitor, J. Alloys Compd., 2018, 746, 670–676 CrossRef CAS.
- S. M. Li, K. Yang, P. W. Ye, H. Jiang, Z. Zhang, Q. Huang and L. Y. Wang, Hierarchical interpenetrating rHGO-decorated NiCo2O4 nanowires architectures for high-performance supercapacitors, Appl. Surf. Sci., 2019, 473, 326–333 CrossRef CAS.
- Z. J. Fan, J. Yan, T. Wei, L. J. Zhi, G. Q. Ning, T. Y. Li and F. Wei, Asymmetric supercapacitors based on graphene/MnO2 and activated carbon nanofifiber electrodes with high power and energy density, Adv. Funct. Mater., 2011, 21, 2366–2375 CrossRef CAS.
- X. Wu, L. Jiang, C. Long, T. Wei and Z. Fan, Dual support system ensuring porous Co-Al hydroxide nanosheets with ultrahigh rate performance and high energy density for supercapacitors, Adv. Funct. Mater., 2015, 25, 1648–1655 CrossRef CAS.
- M. Gao, K. Le, D. Xu, Z. Wang, F. Wang, W. Liu, H. Yu, J. Liu and C. Chen, Controlled sulfidation towards achieving core-shell 1D-NiMoO4@2DNiMoS4 architecture for high-performance asymmetric supercapacitor, J. Alloys Compd., 2019, 804, 27–34 CrossRef CAS.
- Y. Zhao, X. He, R. Chen, Q. Liu, J. Liu, J. Yu, J. Li, H. Zhang, H. Dong, M. Zhang and J. Wang, A flexible all-solid-state asymmetric supercapacitors based on hierarchical carbon cloth@CoMoO4@NiCo layered double hydroxide core-shell heterostructures, Chem. Eng. J., 2018, 352, 29–38 CrossRef CAS.
- S. Kumar, G. Saeed, N. H. Kim and J. H. Lee, Hierarchical nanohoneycomb-like CoMoO4-MnO2 core-shell and Fe2O3 nanosheet arrays on 3D graphene foam with excellent supercapacitive performance, J. Mater. Chem. A, 2018, 6, 7182–7193 RSC.
- F. Lai, Y. Huang, Y. E. Miao and T. Liu, Controllable preparation of multi-dimensional hybrid materials of nickel-cobalt layered double hydroxide nanorods/nanosheets on electrospun carbon nanofibers for high-performance supercapacitors, Electrochim. Acta, 2015, 174, 456–463 CrossRef CAS.
- Y. Cao, L. An, L. Liao, X. Liu, T. Ji, R. Zou, J. Yang, Z. Qin and J. Hu, Hierarchical core/shell structures of ZnO nanorod@CoMoO4 nanoplates used as a high-performance electrode for supercapacitors, RSC Adv., 2016, 6, 3020–3024 RSC.
- W. Wang, L. Yang, F. Qu, Z. Liu, G. Du, A. M. Asiri, Y. Yao, L. Chen and X. Sun, A self-supported NiMoS4 nanoarray as an efficient 3D cathode for the alkaline hydrogen evolution reaction, J. Mater. Chem. A, 2017, 5, 16585–16589 RSC.
- J. X. Wang, J. W. Zhao, L. R. Qin, B. L. Zhao and Z. Y. Jiang, Synthesis of ordered Ni/NiO nanocables for electrochemical capacitor application, J. Nanopart. Res., 2018, 20, 90 CrossRef.
- X. J. Ma, L. B. Kong, W. B. Zhang, M. C. Liu, Y. C. Luo and L. Kang, Design and synthesis of 3D Co3O4@MMoO4 (M=Ni, Co) nanocomposites as high-performance supercapacitor electrodes, Electrochim. Acta, 2014, 130, 660–669 CrossRef CAS.
- X. He, R. Li, J. Liu, Q. Liu, R. Chen, D. Song and J. Wang, Hierarchical FeCo2O4@NiCo layered double hydroxide core/shell nanowires for high performance flexible all-solid-state asymmetric supercapacitors, Chem. Eng. J., 2018, 334, 1573–1583 CrossRef CAS.
- Z. Wang, H. Wang, S. Ji, H. Wang, D. J. L. Brett and R. Wang, Design and synthesis of tremella-like Ni-Co-S flakes on co-coated cotton textile as high-performance electrode for flexible supercapacitor, J. Alloys Compd., 2020, 814, 151789 CrossRef CAS.
- X. Y. Zhang, L. Wei and X. Guo, Ultrathin mesoporous NiMoO4-modifified MoO3 core/shell nanostructures: enhanced capacitive storage and cycling performance for supercapacitors, Chem. Eng. J., 2018, 353, 615–625 CrossRef CAS.
- M. Li, S. Xu, C. Cherry, Y. Zhu, R. Huang, R. Qi, P. Yang, L. Wang and P. K. Chu, Asymmetrical Supercapacitor Composed of Thin Co(OH)2 Nanoflakes on Three-Dimensional Ni/Si Microchannel Plates with Superior Electrochemical Performance, Electrochim. Acta, 2014, 149, 18–27 CrossRef CAS.
- Y. Ouyang, H. T. Ye, X. F. Xia, X. Y. Jiao, G. M. Li, S. Mutahir, L. Wang, D. Mandler, W. Lei and Q. L. Hao, Hierarchical electrodes of NiCo2S4 nanosheets anchored sulfur-doped Co3O4 nanoneedles with advanced performance for battery-supercapacitor hybrid devices, J. Mater. Chem. A, 2019, 7, 3228–3237 RSC.
- J. Yan, S. C. Wang and Y. Chen, Smart in situ construction of NiS/MoS2 composite nanosheets with ultrahigh specifific capacity for high-performance asymmetric supercapacitor, J. Alloys Compd., 2019, 811, 151915 CrossRef CAS.
- W. B. Lv, L. Li, Q. H. Meng and X. T. Zhang, Molybdenum-doped CuO nanosheets on Ni foams with extraordinary specific capacitance for advanced hybrid supercapacitors, J. Mater. Sci., 2020, 55, 2492–2502 CrossRef CAS.
Footnote |
† Electronic supplementary information (ESI) available. See DOI: 10.1039/d0ra03253k |
|
This journal is © The Royal Society of Chemistry 2020 |
Click here to see how this site uses Cookies. View our privacy policy here.