DOI:
10.1039/D0RA03149F
(Paper)
RSC Adv., 2020,
10, 18526-18532
Electrochemical sensor using poly-(L-cysteine) functionalized CuO nanoneedles/N-doped reduced graphene oxide for detection of lead ions†
Received
8th April 2020
, Accepted 7th May 2020
First published on 14th May 2020
Abstract
A highly sensitive and selective electrochemical sensor modified with poly-(L-cysteine)/CuO nanoneedles/N-doped reduced graphene oxide (L-Cys/NN-CuO/N-rGO) has been prepared for the testing of trace Pb2+. The electrochemical performance of this proposed sensor was investigated using electrochemical impedance spectroscopy (EIS). Based on the excellent electrochemical properties of NN-CuO/N-rGO as well as the specific complexation of natural substance L-cysteine with Pb2+, the L-Cys/NN-CuO/N-rGO was applied as a voltammetric biosensor for the determination of trace Pb2+ at pH 5.0. Under the optimum experimental conditions, the voltammetric peak current was linear with the Pb2+ concentration over the range from 0.001 to 5.0 nM and 5.0 to 1000 nM, respectively, with a low detection limit for Pb2+ concentration on the biosensor of 8.0 × 10−5 nM (S/N = 3). The significant sensitivity, selectivity, and electron conductivity of this L-Cys/NN-CuO/N-rGO modified electrode have also been studied. The specific detection of Pb2+ in water samples was also carried out.
1. Introduction
Transition heavy metal ions, especially lead ions (Pb2+) can accumulate in the human body, which results in sub-toxic exposure levels to toxic levels over a period of accumulation.1,2 The lead ion has been classified as a carcinogenic ion by the WHO International Agency for Research on Cancer. According to the exposure time and the concentration levels of Pb2+, the long-term exposure to low concentrations of Pb2+ in the environment may lead to adverse health problems, which would increase the risk of cancer. Thus, a highly specific and sensitive method for the analysis of Pb2+ in food and the human environment has received more attention. Generally speaking, electrochemical methods have many advantages, such as cheap and portable equipment, simple operation, as well as high specificity and sensitivity. In recent years, electrochemical techniques have been developed for the analysis and detection of Pb2+. Various types of electrochemical sensor have been constructed for the determination of trace metal contamination.
Graphene has a two-dimensional nanostructure and attractive properties, for instance, large surface area, high electron conductivity and excellent mechanical stability,3 which makes it extensive applications, such as using as biosensors,4 and electrochemical determination of heavy metal ions.5 In addition, it has been clearly studied that graphene/metal oxide nanocomposites have showed more attractive properties in their promising applications than using a single graphene or nano-metal oxide,6–9 because the advantages of the two substances can be better displayed. So, it is a significative and attractive strategy that CuO nanomaterials are synthesized and grown on graphene sheets as the electrode material to fabricate electrochemical sensors. The prepared nanocomposite exhibits excellent synergistic effect of CuO nanomaterials and graphene compared with the sole substance.9–11 Majumdar et al.12 have successfully prepared CuO nanoparticles anchored on graphene surface for energy storage applications. Felix et al.13 have fabricated graphene/CuO nanocomposites by electrode position method, and the CuO coated graphene served as a superior electrode material for non-enzymatic glucose detection. Moreover, the CuO nanoparticles doped onto graphene sheets not only can effectively stopping the aggregation of CuO nanoparticles and graphene, but also can maintain the electrochemical active sites of CuO nanoparticles. Therefore, the CuO/graphene nanocomposite is an interesting electrode material with enhanced electronic conductivity.
L-Cysteine, one of many important amino acids, is widely used in many medicines, food and biological tissue. Generally speaking, L-cysteine is one of the effective natural products to treat lead in environment. It has come to be seen as a promising candidate for the poisoning treatment of lead in virtue of the chelating abilities of the chelating groups. As far as we know, Wang et al.14 have presented the ultrathin CdSe nanoplatelets modified with either L or D-cysteine molecules to realize lead ion detection. Cheng et al.15 have fabricated a biosensor by using L-cysteine-modified gold nanoparticles/nitrogen-doped graphene composite for lead determination. Zhou et al.16 have reported an L-cysteine/graphene electrochemical sensors for determining lead ion. These reports have showed that L-cysteine, as a natural product, can form sensitive electrochemical sites on the surface of the sensor. Moreover, L-cysteine may identify Pb2+ in wastewater to produce stable complexes leading to pre-concentration purposes, and realize the detection of Pb2+.
In this study, a nano-needle CuO/N-doped reduced graphene oxide nanocomposite was synthesized and used as electrode sensing material on the electrode. Subsequently, the nano-needle CuO/N-doped reduced graphene oxide modified electrode was covered with L-cysteine by using electropolymerization. The electrode constructing process is displayed in Scheme 1. To the best of our knowledge, it is the first time that nano-needle CuO/N-doped reduced graphene oxide nanocomposite and L-cysteine are bound together through preparing a sensor to research their unique synergistic effect for the electrochemical determination of Pb2+. Electrochemical behaviors of Pb2+ on the prepared sensor were carefully studied and a sensitive square wave voltammetry (SWV) for Pb2+ detection was further established. The proposed method showed excellent electrochemical behaviors and could be employed for the detection of Pb2+ in real water samples.
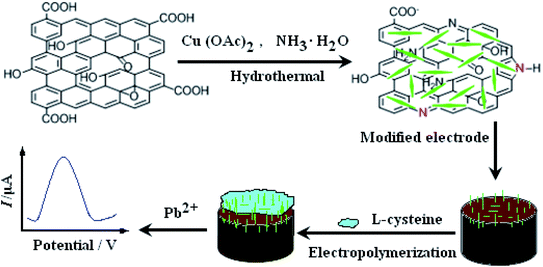 |
| Scheme 1 The constructing process of the L-Cys/NN-CuO/N-rGO modified electrode. | |
2. Experimental
2.1. Reagent and apparatus
Graphene oxide (GO, 99 wt%) was purchased from Sigma-Aldrich. L-Cysteine (analytical reagent, purity ≥ 99.8%) in this study was obtained from Chinese Institutes for Food and Drug Control. All other chemicals, such as PbNO3, Cu(NO3)2·3H2O, Cu(Ac)2·3H2O, KNO3, K3Fe(CN)6, K4Fe(CN)6, NiSO4·7H2O, Cd(NO3)2·4H2O, and Zn(NO3)2·6H2O, were of analytical grade purity (purity ≥ 99.5%) and provided from Sinopharm Chemical Reagent Co., Ltd. Unless otherwise stated, the buffer solution is a 0.1 M KNO3 (pH 5.0). The deionized water was used for all the experiments. And the used all solutions were deoxygenated by nitrogen. CuO nanoneedles/N-doped reduced graphene oxide (NN-CuO/N-rGO) was synthesized according to our former report.17 Total solution volume used in Pb2+ detection tests was 10 mL in electrolytic cell.
All the electrochemical experiments were executed on a CHI660e electrochemical workstation (Shanghai Chenhua Instrument, China) with a conventional three-electrode system. The L-Cys/NN-CuO/N-rGO modified electrode was used as the working electrode. An Ag/AgCl and a platinum wire electrode were used as a reference electrode and auxiliary electrode, respectively. Scanning electron microscopy (SEM) was conducted on a JSM-6700F scanning electron microscope (Japan Electron Company, Japan).
2.2. Fabrication of the modified electrode
According to our former report,17 NN-CuO/N-rGO and N-rGO were synthesized. NN-CuO/N-rGO modified carbon paste electrode (CPE) was prepared as follows: 0.8 g NN-CuO/N-rGO, 1.2 g graphite powder and a modest amount of silicon oil were mixed together and fully ground in an agate mortar. The resulting homogeneous paste was filled into one end of a glass tube (d = 4 mm) and the other end was inserted by a copper wire for electrical contact. So, NN-CuO/N-rGO/CPE was prepared. N-rGO/CPE was obtained by the similar process. CPE was also made by the same procedure without NN-CuO/N-rGO and N-rGO. The fresh prepared electrodes were polished carefully on a weighing paper just before use.
L-Cysteine was electro-polymerized on the prepared NN-CuO/N-rGO/CPE by cyclic voltammetry in the potential range of −0.8 to 2.0 V for 10 cycles at the scan rate of 0.1 mV s−1 in 1.0 × 10−3 M L-cysteine solution. After that, the L-Cys/NN-CuO/N-rGO/CPE was obtained. And then the electrode surface was cleaned with deionized water and N2-dried.
3. Results and discussion
3.1. Characterization of the nanocomposites
The shape of the prepared materials was carried out by SEM (seen Fig. 1). Fig. 1a obviously revealed a wrinkled texture of N-rGO planar sheets, showing that the essential structure of graphene was splendid existed. As can be seen from Fig. 1b, a great deal of nanoneedle-like CuO permeate the N-rGO planar sheets, and equally distributed on it with the wide size of about 20–100 nM and long size of about 0.5 μm. Fig. 1c shows finally electrode of L-Cys/NN-CuO/N-rGO/CPE. A thin film was covered on the electrode surface, showing the formation of electropolymerization of L-cysteine. Moreover, the electropolymerization process has not altered the CuO needle morphology. The formations of nanoneedle-like CuO maybe intensively increase the specific surface area of the as-prepared materials. This experimental result was consistent with our former report.17 From the XPS (Fig. S1†) and EDS examinations (Fig. S2†), the elemental composition was confirmed the presence of C, O, N and Cu elements which have an uniform distribution within nanocomposite.
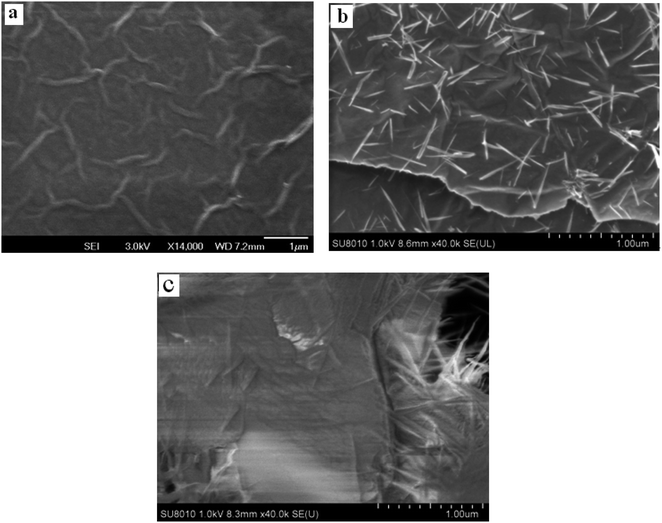 |
| Fig. 1 SEM images of N-rGO (a), NN-CuO/N-rGO nanocomposite (b) and L-Cys/NN-CuO/N-rGO/CPE (c). | |
3.2. Electropolymerization of L-cysteine
As shown in Fig. 2, L-cysteine was electro-polymerized on the prepared NN-CuO/N-rGO/CPE by cyclic voltammetry in the potential range of −0.8 to 2.0 V at a scan rate of 0.1 mV s−1 in 1.0 × 10−3 M L-cysteine solution (0.1 M phosphate buffer solution, pH 6.86). During the first scan, there were two oxidation peaks, and the oxidation–reduction peak current increased with the increase of scanning times, which indicated that L-cysteine had been fixed on the electrode surface.18 After the electropolymerization was completed, the electrode was washed with deoxygenated water. Compared with the NN-CuO/N-rGO/CPE, there was a layer of material with special luster on the electrode surface, which explained that the L-Cys/NN-CuO/N-rGO/CPE had been formed.
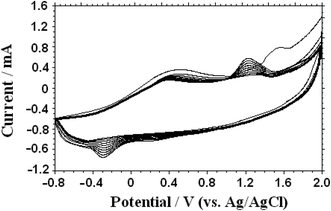 |
| Fig. 2 Electro-polymerized process of L-cysteine on the prepared NN-CuO/N-rGO/CPE by cyclic voltammetry for 10 cycles at the scan rate of 0.1 mV s−1. | |
The effect of the thickness of the electro-polymerized film on the response current of Pb2+ was studied. Typically speaking, the film thickness can be easily adjusted by the number of cyclic scans. Initially, the current value of Pb2+ increased with increasing number of scan cycles. A maximum current value was obtained at 10 scan cycles, and then decreased with further increase of the scan cycle number. This reason can be possibly explain that very thin films do not have enough active sites, whereas too thick films would block the electron transfer. Therefore, 10 cyclic scans were selected as the optimal condition to fabricate the electro-polymerized film on the electrode surface.
3.3. Electrochemical impedance studies
Electrochemical impedance spectroscopy (EIS) is an effective measure for proving the change of electrode interface. Generally speaking, the semicircle diameter equals to the electron transfer resistance (Ret). Fig. 3 showed the EIS of the NN-CuO/N-rGO/CPE (a), N-rGO/CPE (b), L-Cys/NN-CuO/N-rGO/CPE (c) and CPE (d) in 5.0 mM Fe(CN)63−/4− and 0.1 M KCl solution in the frequency range swept 105 to 0.1 Hz. The AC voltage amplitude was 5 mV, and the applied potential was 0.250 V. The Randles equivalence circuit model used to fit the experimental data was shown in the inset of Fig. 3, which included the ohmic resistance of the electrolyte (Rs), the electron transfer resistance (Ret), the double layer capacitance (Cdl), and Warburg impedance (Zw). The bare CPE had a big semicircle diameter with a Ret value of 395.0 Ω. While the rGO/CPE and NN-CuO/N-rGO/CPE showed low Ret of 140.5 and 74.2 Ω, respectively, However, L-Cys/NN-CuO/N-rGO/CPE showed a Ret of 172.3 Ω, higher than the values of the rGO/CPE or NN-CuO/N-rGO/CPE. This phenomenon can be ascribed to the rGO nanosheets or NN-CuO/N-rGO in the electrode, but the L-cysteine layer obstructed the electron transport. The EIS demonstrated that the construction of each step had been successfully conformed.
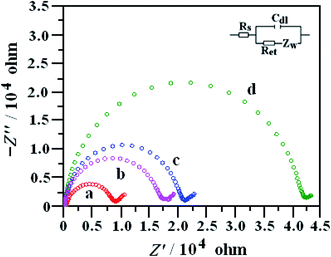 |
| Fig. 3 Nyquist plots of the EIS the NN-CuO/N-rGO/CPE (a), N-rGO/CPE (b), L-Cys/NN-CuO/N-rGO/CPE (c) and CPE (d) in 5.0 mM Fe(CN)63−/4− and 0.1 M KCl solution in the frequency range swept 105 to 0.1 Hz at 0.25 V. | |
The microscopic areas of the prepared modified-electrodes were obtained by cyclic voltammetry employing 1.0 mmol L−1 K3Fe(CN)6 as a redox probe in the 0.1 mol L−1 KCl electrolyte at different scan rates.19,20 For a reversible process, the anodic peak current ip is linear to ν1/2 as follows:
ip = (2.69 × 105)n3/2AC0DR1/2ν1/2 |
where
ip refers to the anodic peak current,
A the surface area of the electrode,
v the scan rate and
C0 the concentration of K
3Fe(CN)
6. For 1.0 mmol L
−1 K
3Fe(CN)
6, the electron transfer
n = 1, the diffusion coefficient
DR = 7.60 × 10
−6 cm
2 s
−1. Thus, from the slope of the
ip vs. ν1/2 relation, the microscopic areas of the N-rGO/CPE, NN-CuO/N-rGO/CPE and
L-Cys/NN-CuO/N-rGO/CPE were calculated to be 0.9729 cm
2, 1.0738 cm
2, 1.0937 cm
2, respectively, which were great higher than the bare CPE (0.1256 cm
2). In combination with the EIS analysis, the electrochemically active surface area of the CPE, N-rGO/CPE, NN-CuO/N-rGO/CPE and
L-Cys/NN-CuO/N-rGO/CPE were calculated to be 49.6, 136.7, 79.7 and 188.4 ohm cm
2.
3.4. Electrochemical behaviors of Pb2+ on different electrodes
The electrochemical features of 1.0 μM Pb2+ on different electrodes was studied through the square wave voltammetry (SWV) in 0.1 M KNO3 (pH 5.0), which has been shown in Fig. 4. After electrochemical accumulation time for 250 s at an accumulation potential −0.6 V, frequency 15 Hz, and amplitude 25 mV, a well-shaped electrochemical stripping peak appears in the potential range from−0.48 to −0.60 V with different stripping peak current on different electrodes. An unobvious stripping peak current of Pb2+ was seen on the bare CPE at −0.52 V. In contrast, the peak current can be obviously enhanced on the NN-CuO/N-rGO and N-rGO modified electrodes because of the larger surface area and fast electron conductivity of the electrodes. It's interesting to see that the peak current remarkably increased after the introduction of L-cysteine on the NN-CuO/N-rGO modified electrode. The possible reason may be that L-cysteine can effective combine with Pb2+ and provide more enrichment sites for Pb2+ accumulation. All the experimental results showed that the interaction between analytical object of Pb2+ and L-Cys/NN-CuO/N-rGO has improved the electrochemical signal, and furnished a possibility for the selective recognition of Pb2+.
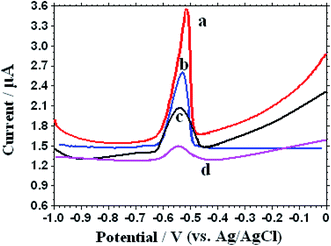 |
| Fig. 4 Different SWVs of L-Cys/NN-CuO/N-rGO/CPE (a), NN-CuO/N-rGO/CPE (b), N-rGO/CPE (c) and CPE (d) in the presence of 1.0 μM Pb2+ in 0.1 M KNO3 (pH 5.0). | |
3.5. Analytical conditions
In order to obtain the maximum efficacy of L-Cys/NN-CuO/N-rGO/CPE, some experimental conditions were optimized, such as accumulation time for Pb2+ and different pH value of electrolyte solution at the preconcentration potential −0.6 V, frequency 15 Hz and amplitude 25 mV. From Fig. 5a, the peak current response of the constructed sensor increased rapidly with the augmentation of accumulation time for Pb2+ and achieved a maximum current at 250 s. Thus, an accumulation time of 250 s was selected as the experimental accumulation time for Pb2+. In order to evaluate the effect of pH for the analytical Pb2+, different pH values of the electrolyte in the pH range of 3.0–7.0 were researched. The experimental results were displayed in Fig. 5b. In the pH range of 3.0–4.0, the current values had a trend of slow growth. However, a sharp increase of the peak current value appeared in the pH range from 4.0 to 5.0. The possible reason may be that the electrode surface is unstable in acidic environment, resulting in lower current corresponding to the decreasing of pH value. Possibly, the response current was decreased along with the increasing from pH 5 to pH 7 because of the gradual hydrolysis of partial Pb2+ with the increase of pH value.
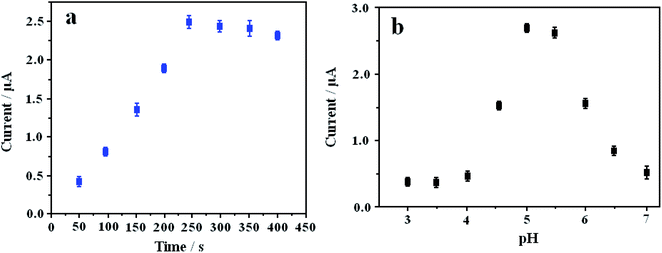 |
| Fig. 5 Influences of different accumulation time of 1.0 μM Pb2+ in 0.1 M KNO3 (pH 5.0) (a) and different pH in 1.0 μM Pb2+ in 0.1 M KNO3 (b) on the striping peak current. Error bars are the standard deviation for three determinations. | |
3.6. Analytical performance
Under the optimal conditions, SWVs were used to study the electrochemical responses of different concentrations Pb2+ on the L-Cys/NN-CuO/N-rGO/CPE in 0.1 M KNO3 (pH 5.0) at the preconcentration time 250 s and potential −0.6 V, frequency 15 Hz and amplitude 25 mV. As can be seen in Fig. 6, the SWVs peak current gradually increasing accompanied with the enhancement of Pb2+ concentration. Moreover, the peak currents are linear with the Pb2+ concentration in the ranges of 0.001–5.0 nM and 5.0–1000 nM, respectively (as displayed in Fig. 7). The limit of detection (LOD) of Pb2+ (S/N = 3) on the biosensor was determined to be 8.0 × 10−5 nM. Table 1 shows the comparative characteristics of the proposed sensor with some other reported sensors for the measurement of Pb2+. The low detection limit of Pb2+ (8.0 × 10−5 nM) is lower than some other reported Pb2+ sensor,21,23–29 except hemin/G-quadruplex bimolecular-based Pb2+ biosensors.22 Comparing with the other reported different materials-modified sensors for Pb2+ detection, the as-constructed novel biosensor showed excellent capability for Pb2+ detection, such as lower detectable concentration, broader working range, and sensitivity. And more importantly, the developed biosensor has merits in preparation, for instance, a simple and convenient hydrothermal method without any surfactants or templates, cheapness with the employment of the natural substance L-cysteine for Pb2+ specific binding, without the employment of biological DNA and enzyme, as well as environmentally friendly without any toxicant.
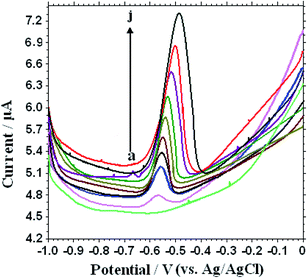 |
| Fig. 6 SWV response of different concentrations of Pb2+ solution on the L-Cys/NN-CuO/N-rGO/CPE in 0.1 M KNO3 (pH 5.0) (a → j): 0, 0.001, 0.08, 0.5, 1.0, 1.5, 2.2, 3.0, 4.0 and 5.0 nM. | |
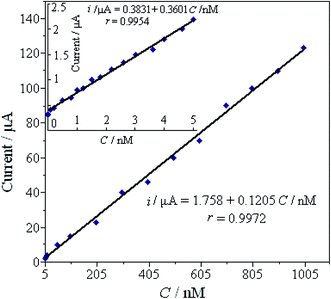 |
| Fig. 7 The linear relationship of the peak current against the concentration of Pb2+ in the higher range of 5.0–1000 nM; the inset is the linear relationship between the current and concentration of Pb2+ in the lower range of 0.001–5.0 nM. | |
Table 1 Comparison of different materials for the electrochemical detection of Pb2+
Electrode materials |
Detection limit (μM) |
Linear range (μM) |
References |
CNT/rGO/Bi |
0.00097 |
0.097–0.97 |
19 |
Hemin/G-quadruplex nanowires |
1 × 10−9 |
1× 10−8 to 0.2 |
20 |
Pb2+-Dependent DNAzyme/gold nanoparticles@Fe3O4 |
3 × 10−5 |
0.0001–0.1 |
21 |
N-BDMP/Fe3O4/IL |
0.0009 |
1.20–0.120 |
22 |
Sb2O3/MWCNTs |
0.013 |
0.024–0.169 |
23 |
MOF-177 |
0.003 |
10–120 |
24 |
Evertically aligned MoS2 nanofilm |
0.001 |
0–0.097 |
25 |
Fe3O4/GN/GE |
4.1× 10−7 |
1 × 10−6 to 0.0005; 0.0005–1 |
26 |
Bismuth–graphene |
1.9 × 10−4 |
4.8× 10−4 to 0.2413 |
27 |
L-Cys/NN-CuO/N-rGO |
8.0 × 10−8 |
1 × 10−6 to 0.005; 0.005–1 |
This work |
3.7. Reproducibility and stability
The reproducibility and stability of the biosensor are the very important properties in the actual applications. In our study, the reproducibility of the L-Cys/NN-CuO/N-rGO modified electrode was tested by measuring the stripping peak current of 1.0 μM Pb2+ in 0.1 M KNO3 (pH 5.0) for five successive determinations. The average relative standard deviation (RSD) was 4.66% with the same constructed electrode. The long-time stability of the as-prepared sensor was explored in two weeks with an evaluation every three days. The prepared modified electrode was put into a fridge (4 °C) when it is not used. The stripping peak currents of Pb2+ at the L-Cys/NN-CuO/N-rGO modified CPE retained about 87% of its original signal. These experimental results showed that the reproducibility and stability of the improved sensor were acceptable.
3.8. Selectivity of the prepared electrode
The selectivity of the proposed biosensor is examined before it was used to analyze the actual samples. We investigated the coexistent interference ions, such as Cd2+, Mn2+, Zn2+, Ni2+, Hg2+ and Ca2+ to evaluate the specificity of the prepared biosensor. As can be seen from Fig. 8, the histograms reveal the changed current of the sensor after the detection for other coexisting metal ions. It is obvious that the current signal was significantly larger with Pb2+ of 1.0 μM than those of other coexisting metal ions (1.0 μM). The experimental phenomena clearly proved that the proposed method could reasonable selectively determine Pb2+ in the presence of other interfering metal ions.
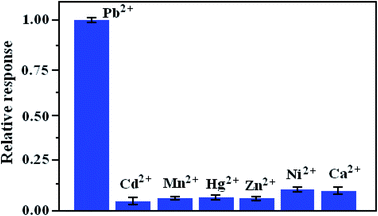 |
| Fig. 8 Effect of the relative current response of 1.0 μM Pb2+ on L-Cys/NN-CuO/N-rGO/CPE. Error bars represent standard deviation, n = 3. | |
3.9. The practicality of the proposed sensor
To evaluate the practicality of the constructed method, standard addition recovery tests for tap water, rain water, and river water (river located in Anyang, China) were researched. Before testing, all samples were filtered through filter membrane to get rid of insoluble substance, and then adjust it to the optimized pH by 0.1 M KNO3 and HNO3. The detection results are listed in Table 2. As can be observed, the recoveries are ranged from 94% to 103%, and relative standard deviations (RSDs) are 2.85–6.01%, respectively, indicating that the proposed biosensor has a potential application in the specific detection of Pb2+ in real samples.
Table 2 Determination of Pb2+ recovery by the suggested biosensor in different water samples (nM)
Samples |
Pb2+ added (nM) |
Pb2+ found (nM) |
Recovery (%) |
RSD (%) |
Tab water |
0 |
0.0192 ± 0.0010 |
— |
3.56 |
100 |
96 ± 1.05 |
96 ± 1.1 |
5.27 |
1000 |
969 ± 16.35 |
96.9 ± 1.6 |
4.10 |
Rain water |
0 |
0.0899 ± 0.0123 |
— |
2.85 |
100 |
98 ± 1.61 |
98 ± 1.6 |
3.97 |
1000 |
1000 ± 20.67 |
100.0 ± 2.1 |
3.84 |
River water |
0 |
0.186 ± 0.051 |
— |
2.97 |
100 |
94 ± 1.94 |
94 ± 1.9 |
6.01 |
1000 |
1006 ± 23.20 |
100.6 ± 2.3 |
4.32 |
Industrial effluent |
0 |
0.271 ± 0.037 |
— |
3.02 |
100 |
103 ± 2.29 |
103 ± 2.3 |
4.17 |
1000 |
1015 ± 35.71 |
101.5 ± 3.6 |
5.79 |
4. Conclusions
In conclusion, an effective method was proposed to measure Pb2+ by combining L-cysteine and NN-CuO/N-rGO as electrode materials. L-Cysteine, as the natural substance was used as specific receptor for Pb2+ analysis and NN-CuO/N-rGO nanocomposite as improved sensing material. The proposed biosensor displayed useful linear ranges, acceptable detection limit, high sensitivity and good selectivity for electrochemical detection of Pb2+. This study indicates that the present L-Cys/NN-CuO/N-rGO/CPE provide a specific potential applications in the development of other nanodevices for metal ions detections.
Conflicts of interest
There are no conflicts to declare.
Acknowledgements
We acknowledge the financial support of the National Natural Science Foundation of China (21804002), the Program for Science and Technology Innovation Talents at the University of Henan Province (20HASTIT009), and Anyang Science and Technology Bureau (No. 28).
References
- C. Jiang, Y. Li, H. Wang, D. Chen and Y. Wen, Sens. Actuators, B, 2020, 307, 127625–127632 CrossRef.
- Z. Yang, H. Wu, X. Yi, J. Tang, W. Yun, W. Han and X. Chen, Biosens. Bioelectron., 2019, 144, 111679–111688 CrossRef CAS PubMed.
- J. C. Meyer, A. K. Geim, M. I. Katsnelson, K. S. Novoselov, T. J. Booth and S. Roth, Nature, 2007, 446, 60–63 CrossRef CAS PubMed.
- S. Taniselass, M. K. M. Arshad and S. C. B. Gopinath, Biosens. Bioelectron., 2019, 130, 276–292 CrossRef CAS PubMed.
- Y. Zuo, J. Xu, X. Zhu, X. Duan, L. Lu and Y. Yu, Microchim. Acta, 2019, 186, 1–9 CrossRef CAS PubMed.
- S. Kim, K. C. Kwon, J. Y. Park, H. W. Cho, I. Lee, S. Y. Kim and J. L. Lee, ACS Appl. Mater. Interfaces, 2016, 8, 12932–12939 CrossRef CAS PubMed.
- J. H. Kim, A. Mirzaei, Y. Zheng, J. H. Lee, J. Y. Kim, H. W. Kim and S. S. Kim, Sens. Actuators, B, 2019, 281, 453–461 CrossRef CAS.
- Y. Li, X. Wang, Q. Yang, M. S. Javed, Q. Liu, W. Xu, C. Hu and D. Wei, Electrochim. Acta, 2017, 234, 63–70 CrossRef CAS.
- Y. Xie, Y. Yu, L. Lu, X. Ma, L. Gong, X. Huang, G. Liu and Y. Yu, J. Electroanal. Chem., 2018, 812, 82–89 CrossRef CAS.
- Y. Liu, M. Li, H. Li, G. Wang, Y. Long, A. Li and B. Yang, ACS Sustainable Chem. Eng., 2019, 7, 19537–19545 CrossRef CAS.
- F. Faranak, R. Mansour, H. Mohammad Jafar and K. Hasuck, Microchim. Acta, 2018, 185, 57–66 CrossRef PubMed.
- D. Majumdar, N. Baugh and S. K. Bhattacharya, Colloids Surf., A, 2017, 512, 158–170 CrossRef CAS.
- S. Felix, X. Charles and A. N. Grace, Sens. Lett., 2017, 15, 60–64 CrossRef.
- X. Wang, J. Hao, J. Cheng, J. Li, J. Miao, R. Li, Y. Li, J. Li, Y. Liu, X. Zhu, Y. Liu, X. W. Sun, Z. Tang, M. H. Delville, T. He and R. C. Chiral, Nanoscale, 2019, 11, 9327–9334 RSC.
- Y. Cheng, H. Fa, W. Yin, C. Hou, D. Huo and F. Liu, J. Solid State Electrochem., 2015, 20, 327–335 CrossRef.
- W. Zhou, C. Li, C. Sun and Y. Yang, Food Chem., 2016, 192, 351–357 CrossRef CAS PubMed.
- S. L. Yang, G. Li, D. Wang, Z. Qiao and L. B. Qu, Sens. Actuators, B, 2017, 238, 588–595 CrossRef CAS.
- B. R. LyrioFerraz, F. R. F. Leite and A. R. Malagutti, Talanta, 2016, 154, 197–207 CrossRef PubMed.
- Q. Xu and S. F. Wang, Microchim. Acta, 2005, 151, 47–52 CrossRef CAS.
- B. Rezaei and S. Z. Mirahmadi Zare, Sens. Actuators, B, 2008, 134, 292–299 CrossRef CAS.
- X. Xuan and J. Y. Park, Sens. Actuators, B, 2018, 255, 1220–1227 CrossRef CAS.
- M. Qing, Y. Yuan, W. Cai, S. Xie and J. Zhang, Sens. Actuators, B, 2018, 263, 469–475 CrossRef CAS.
- L. Zhang, H. Deng, R. Yuan and Y. Yuan, Mikrochim. Acta, 2019, 186, 709–718 CrossRef PubMed.
- I. Vida, R. Mosayeb and M. Jafar, Anal. Bioanal. Chem. Res., 2019, 6, 405–417 Search PubMed.
- T. L. Hai, L. C. Hung, T. T. B. Phuong, B. T. T. Ha, B. S. Nguyen, T. D. Hai and V. H. Nguyen, Microchem. J., 2020, 153, 104456–104464 CrossRef CAS.
- S. Sangeetha and G. Krishnamurthy, Bull. Mater. Sci., 2020, 43, 29–37 CrossRef CAS.
- J. H. Hwang, M. A. Islam, H. Choi, T. J. Ko, K. L. Rodriguez, H. S. Chung, Y. Jung and W. Hyoung Lee, Anal. Chem., 2019, 91, 11770–11777 CrossRef CAS PubMed.
- B. He, X. F. Shen, J. Nie, X. L. Wang, F. M. Liu, W. Yin, C. J. Hou, D. Q. Huo and H. B. Fa, J. Solid State Electrochem., 2018, 22, 3515–3525 CrossRef CAS.
- W. Wonsawat, S. Chuanuwatanakul, W. Dungchai, E. Punrat, S. Motomizu and O. Chailapakul, Talanta, 2012, 100, 282–289 CrossRef CAS PubMed.
Footnote |
† Electronic supplementary information (ESI) available. See DOI: 10.1039/d0ra03149f |
|
This journal is © The Royal Society of Chemistry 2020 |
Click here to see how this site uses Cookies. View our privacy policy here.