DOI:
10.1039/D0RA03086D
(Review Article)
RSC Adv., 2020,
10, 20046-20056
Retracted Article: Recent application of visible-light induced radicals in C–S bond formation
Received
6th April 2020
, Accepted 29th April 2020
First published on 27th May 2020
Abstract
The sulphur centered radicals, produced from various organic compounds, in high efficiency by single-electron-transfer (SET) oxidation. These radicals are highly reactive intermediates having various applications in the construction of organosulphur compounds in the field of synthetic organic chemistry. These S-centred radical-mediated organic transformations have been achieved using photoredox catalysts, including organic dyes and transition metal catalysts, as well as in the absence of any catalyst. Compared with previous methods, photoredox catalysis is inexpensive and features the advantages of being environmentally benign, highly efficient and easy to use. This review focuses on recent developments in the photocatalyzed carbon–sulphur bond formation.
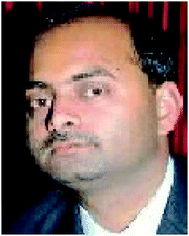 Vishal Srivastava | Vishal Srivastava is working as an Assistant Professor, Department of Chemistry, C.M.P. College, (Constituent P.G. College of Central University of Allahabad) Prayagraj, India. He has completed his Graduation (B.Sc.), Post-Graduation (M.Sc.) in Organic Chemistry and Doctoral Degree (D.Phil.) from Department of Chemistry, University of Allahabad, India. His current research work involves the designing of novel biologically active photoredox catalysed synthetic organic compounds. |
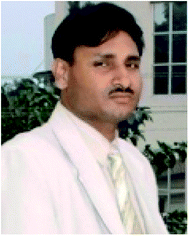 Pravin K. Singh | Pravin K. Singh is working as an Assistant Professor, Department of Chemistry, C.M.P. College, Allahabad, India (Constituent P.G. College of Central University of Allahabad). Dr Singh is actively engaged in advanced research work for the development of environmentally benign novel synthetic routes for various bioactive heterocyclic compounds. He has completed his B.Sc., M.Sc., Doctorate (D.Phil.) and Post-Doctorate (D.Sc.) from University of Allahabad, India. |
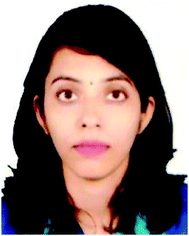 Arjita Srivastava | Arjita Srivastava is working as an Assistant Professor in the Department of Chemistry, C. M. P. P. G College, Prayagraj. She has completed her B.Sc. from Ewing Christian College, University of Allahabad and her M.Sc. in Organic Chemistry, D.Phil. from Department of Chemistry, University of Allahabad, India. Her current research interest mainly focuses on utilizing visible light and efficient photocatalysts as green tools for the synthesis of bio-active compounds. |
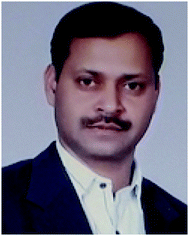 Praveen P. Singh | Praveen P. Singh is working as an Assistant Professor in the Department of Chemistry at the United College of Engineering and Research, Prayagraj, India. He obtained his B.Sc., M.Sc. in Organic Chemistry from T. D. P. G. College (V. B. S Purvanchal University) Jaunpur and D.Phil. from Department of Chemistry, University of Allahabad, India. His current research interests include the development of synthetic receptors for the recognition of biological target structures and the application of visible light chemical photocatalysis towards organic synthesis. |
1. Introduction
The construction of C–S bonds is synthetically important because of the large number of sulphur-containing natural products and pharmaceuticals as well as the increasing importance of sulphur-containing products in polymer and material chemistry.1a–e Sulphur-containing organic molecules find widespread utilization in various fields of chemistry.2,3 With an increase in the number of approved organosulphur-based drugs, several studies have been carried out with the aim of introducing the sulphur functional unit in organic molecules.4,5 Among organosulphur compounds, vinyl sulfones have been significantly important due to their unique structural motif, which is valuable for designing building blocks in materials science and pharmaceutical science, as well as their intrinsic electron-withdrawing nature; moreover, they serve as functional modulators in various synthetic transformations.6,7 The formation of the C–S bond is of great importance in the synthesis of biologically active molecules and functional materials.8 Sulphur-containing compounds with a neighboring functional group have been successfully prepared from alkenes.9–13 With this background, simultaneous construction of C(sp3)–C(F2R) and C(sp3)–S bonds across the C
C bond has received considerable attention.
Organosulphur compounds continuously provide new challenges as well as opportunities for chemists due to their inherent ability to adopt different sulphur oxidation states.14,15 In recent years, there has been an increase in the demand of organosulphur compounds with C–S bond formations by transition metal catalysis. Moreover, Brønsted acid catalysis amply demonstrates the full potential of these compounds.16,17 Many organosulphur compounds18 are biologically and pharmaceutically active, as well as ubiquitously found in many natural products. Dithioacetals serve as key intermediates19 in the synthesis of many natural products. In particular, dithioacetals are commonly used as a protecting group for carbonyls as well as directing groups for C–H activation reactions.20 Moreover, dithioacetals are known to be used as important precursors for alkylation,21 fluorination,22 hydrogenolysis,23 olefination24 and auto-oxidation25 reactions. This review mainly highlights the recent advances on visible light mediated C–S bond formation for the synthesis of organosulphur compounds via the generation of sulphur centered radicals (Fig. 1).
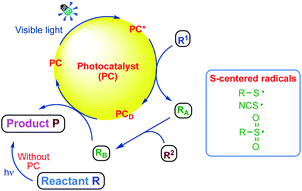 |
| Fig. 1 General scheme involving the application of photocatalysts. | |
Harnessing visible light as a safe, renewable, and inexpensive source of chemical energy to facilitate the construction of complex organic molecules has recently emerged as a powerful strategy in organic chemistry.26–29 This is because solar energy (visible light) is clean, easy to handle and an unlimited energy source, having great prospects for developing sustainable and eco-friendly protocols that can be used in organic synthesis.30 Some pioneering researchers have focused on converting solar energy into chemical energy for chemical transformations,31,32 which includes a promising strategy for the application of photoredox catalysts to initiate single electron transfer (SET) processes.33,34 Visible light photoredox catalysis has recently received considerable attention in organic synthesis owing to the ready availability, sustainability, non-toxicity and ease of handling.35–40 Recently, a superior alternative to transition metal photoredox catalysts, especially metal-free organic dyes such as eosin Y, fluorescein, rose bengal, nile red, perylene and rhodamine B, has been used as economically and ecologically superior surrogates for Ru(II) and Ir(II) complexes in visible-light promoted organic transformations involving SET.41–43 These organic dyes have great potential for application in visible-light-mediated organic synthesis,44–47 which fulfils the basic principle of green chemistry.48–57
2. Organo-photocatalysed C–S bond formation
2.1. Thiol–ene reaction
Thiol–ene reaction has emerged as a powerful tool for combining two molecules. This process has been exploited in many fields such as polymers, materials science and biology. In 2019, A. D. Dilman58 et al. (Scheme 1) reported a convenient protocol for the visible light-promoted thiol–ene click reaction. The proposed strategy has a wide substrate scope, which prescribes the use of stoichiometric ratio of thiol (1) and alkene (2) involving the lewis basic nature of the photocatalyst. The driving force for initiating the entire reaction is a proton-coupled electron transfer within the complex between the thiol and the Lewis base catalyst.
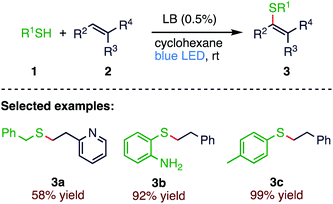 |
| Scheme 1 Photocatalyzed thiol–ene reaction. | |
2.2. Thiolation of allenyl phosphine oxides
In 2017, Wu et al.59 (Scheme 2) reported a novel method for alkenyl C–S bond formation via the photocatalytic thiolation of allenyl phosphine oxides (4) with diaryl disulfides (5).
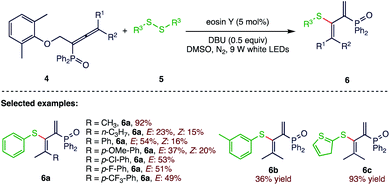 |
| Scheme 2 Thiolation of allenyl phosphine oxides. | |
Under visible light irradiation conditions α-alkenyl C−S bond formation was involved, yielding a series of novel S, P-bifunctionalized butadienes (6) with moderate to excellent yields. The present protocol is a very good example of the thiolation of allenyl phosphine oxides with diaryl disulfides in metal-free and mild conditions. This synthetic strategy has many advantages, including eco-benign procedures and good functional group compatibility.
2.3. Thioacetalization of aldehydes
In 2019, Mal60 et al. (Scheme 3) reported the application of an iodine(III) reagent as a visible-light photocatalyst for chemoselective dithioacetalization. The present protocol is chemoselective, mild, atom-economical and operationally simple. Using this synthetic method, several dithioacetals could be easily accessed at room temperature under environmentally benign condition from a variety of aliphatic and aromatic aldehydes in good to excellent yields (9). This methodology is utilized to construct C–S bonds, which might have a wide application in synthesizing complex molecules.
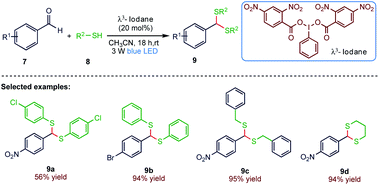 |
| Scheme 3 Thioacetalization of aldehydes. | |
2.4. Thiocyanation reaction of tertiary enaminones
Organic thiocyanates are moieties comprising enriched biological and pharmaceutical activities in both synthesized and naturally occurring molecules. In 2019, Wan61 et al. (Scheme 4) reported a visible light-induced C–H bond thiocyanation under metal-free aerobic conditions. The reaction was catalysed in the presence of rose bengal, which enables the synthesis of thiocyanated alkene derivatives and chromones using NH4SCN (11) as the thiocyano source. Besides providing a simple and efficient approach towards the synthesis of thiocyanated alkene derivatives (12), this protocol achieves the synthesis of even more divergent organic thiocyanates, including thiocyanated chromones and polyfunctionalized alkenes containing primary amino and thiocyano groups, which were achieved by varying the substrate structure or the photocatalyst species.
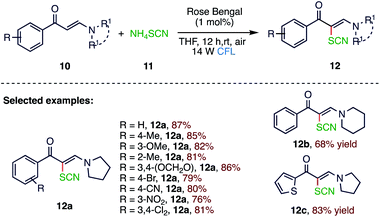 |
| Scheme 4 Thiocyanation reaction of tertiary enaminones. | |
2.5. Hydrosulfonylation reaction
Due to the medicinal and agricultural importance of sulfonyl compounds, their formation through the insertion of sulphur dioxide has been regarded as an attractive pathway. Wu et al.62 (Scheme 5) reported a photoredox catalysed sulfonylation reaction of 4-substituted Hantzsch esters (13), DABCO·(SO2)2, and electron-deficient alkenes (14) at room temperature under visible light irradiation. This sulfonylation reaction under mild conditions shows a broad substrate scope with good functional group compatibility.
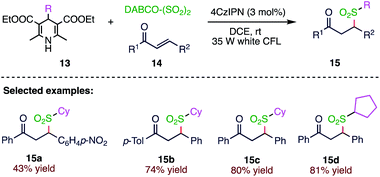 |
| Scheme 5 Reaction of 4-substituted Hantzsch ester, DABCO(SO2)2, and alkene. | |
2.6. Synthesis of 3-arylsulfonylquinoline derivatives
In 2018, Zhang63 et al. (Scheme 6) reported a visible-light-mediated, eosin Y catalyzed three-component synthesis of 3-arylsulfonylquinoline derivatives from N-propargyl aromatic amines (16), diaryliodonium salts (17) and sulphur dioxide (18). This synthetic transformation exhibits an efficient and attractive method for the straightforward synthesis of 3-arylsulfonylquinoline derivatives (19) via the formation of C–S bonds in one step. This strategy represents good functional group tolerance to afford various 3-arylsulfonylquinolines (19) and shows excellent yield in the gram-scale synthesis.
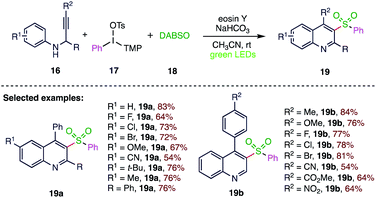 |
| Scheme 6 Synthesis of 3-arylsulfonylquinoline derivatives. | |
2.7. Sulfonylation of quinoline N-oxides
Free radical reactions have become a powerful synthetic tool in organic transformation. In 2019, He et al.64 (Scheme 7) reported an eosin Y catalysed, visible-light-induced deoxygenative C2-sulfonylation of quinoline N-oxides (20) with sulfinic acids (21). This radical reaction provides a simple process to prepare 2-sulfonylquinolines (22) in good to excellent yields. The catalyst requirement in traces, metal and base-free conditions, high scalability and operational simplicity demonstrated that the developed synthetic strategy is an eco-friendly and useful synthetic method.
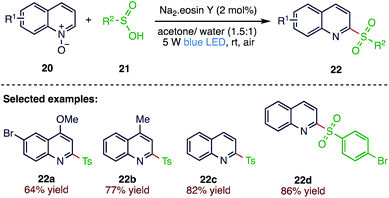 |
| Scheme 7 Sulfonylation of quinoline N-oxides. | |
2.8. Synthesis of allylic sulfones
Photoinduced reactions have attracted considerable attention in recent years. The radical process initiated by the treatment of organotrifluoroborates under photoredox catalysis has attracted growing interest. In 2019, Wu et al.65 (Scheme 8) reported potassium alkyltrifluoroborates as radical reservoirs with the insertion of sulphur dioxide in sulfonylation reaction via photoredox catalysis.
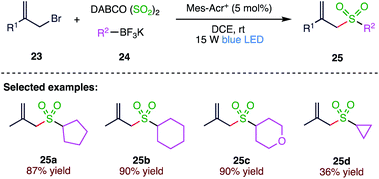 |
| Scheme 8 Synthesis of allylic sulfones. | |
In the present methodology, the generation of diverse allylic sulfones takes place at room temperature by the three-component reaction of potassium alkyltrifluoroborates (24), sulphur dioxide and allylic bromides (23) in the presence of 9-mesityl-10-methyl acridinium perchlorate under visible light irradiation. This transformation proceeds efficiently, and a broad reaction scope is demonstrated with good functional group tolerance. This reaction is initiated by the treatment of potassium alkyltrifluoroborate with an allylic bromide under visible light irradiation in the presence of a photocatalyst, thus leading to an alkyl radical, which is trapped by the sulphur dioxide to provide an alkylsulfonyl radical. This alkylsulfonyl radical then undergoes further transformation to afford the corresponding allylic sulfone (25) (Scheme 9).
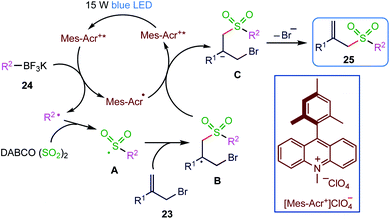 |
| Scheme 9 A plausible mechanism for the generation of allylic sulfones. | |
3. Metal-photocatalysed C–S bond formation
3.1. Difluoromethylation of thiols
Fluoroalkyl groups have received considerable attention as their combination with sulphur atoms generally increases the lipophilicity parameter. By considering these facts, Qing et al.66 (Scheme 10) reported a visible light-induced radical difluoromethylation of aryl, heteroaryl and alkyl thiols (26) with difluoromethyl triphenylphosphonium triflate (27) to afford various difluoromethyl thioethers (28) in moderate to excellent yields. The present protocol implies the application of a readily available CF2H radical source, mild reaction conditions and excellent chemoselective thiol-difluoromethylation. This protocol exhibits an attractive synthetic approach to yield a range of difluoromethyl thioethers under mild conditions with excellent S/X (X = O, N) selectivities.
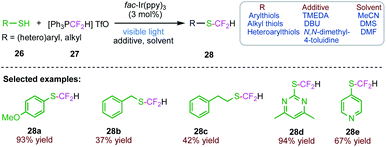 |
| Scheme 10 Difluoromethylation of thiols. | |
3.2. Radical–radical coupling to synthesize thioesters
Oh et al.67 (Scheme 11) proposed a visible-light induced photoredox catalysed synthesis of thioesters (31) from readily available starting materials: acid chlorides (29) and sodium sulfinates (30). The present method follows a direct radical–radical coupling strategy via mild and controlled photochemical approach to synthesise important synthetic building blocks such as thioesters. In this approach, the formation of thiyl radical species has been successfully achieved from sodium sulfinates with the help of acyl radical species.
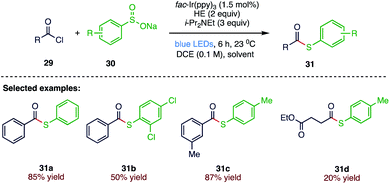 |
| Scheme 11 A radical–radical coupling to synthesize thioesters. | |
3.3. Difluoroalkylation–thiolation of alkenes
The insertion of fluoroalkyl groups into alkenes has a challenging impact on their physical, chemical, and biological properties. Cai et al.68 (Scheme 12) reported an iron-facilitated photoredox catalysed process for the difluoroalkylation–thiolation of alkenes (35). In the present protocol, the Csp3–Csp3 and Csp3–S bonds were simultaneously constructed smoothly under mild conditions. The reaction exhibits a broad substrate scope of alkenes (32) and thiols (33) with good to excellent yields. The synthetic methodology involves a radical mechanism according to the control experiments.
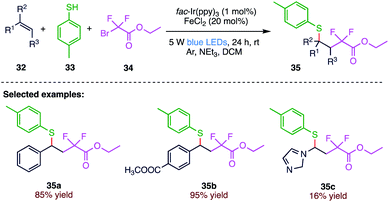 |
| Scheme 12 Difluoroalkylation–thiolation of alkenes. | |
3.4. Hydrothiolation of alkenes and alkynes
For the construction of C–S bonds, the hydrothiolation of alkenes or alkynes with thiols represents an attractive and atom economic approach. Li69 et al. (Schemes 13 and 14) reported a visible light-irradiated general and efficient hydrothiolation of alkenes and alkynes (36) over ZnIn2S4 by applying flower-like microspheres of ZnIn2S4 comprising interweaving nanoflakes, which were synthesised by a solvothermal method. In this synthetic strategy, the reactions between a broad range of thiols (37) and alkynes or alkenes (36) over irradiated ZnIn2S4 afford the corresponding hydrothiolated products (38) in moderate to excellent yields. The present protocol includes the use of solar light and a semiconductor-based photocatalyst to realize the thiol–ene and thiol–yne coupling reactions in a green solvent (methanol), with only stoichiometric amount of thiols required, and is applicable to a broad substrate scope, making this reaction protocol a green, sustainable and cost-effective strategy for the synthesis of thiolated products.
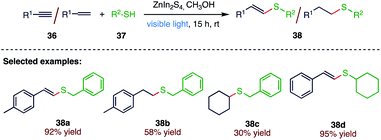 |
| Scheme 13 Hydrothiolation of alkenes and alkynes. | |
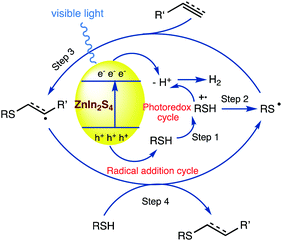 |
| Scheme 14 A plausible mechanism for the hydrothiolation of alkenes and alkynes. | |
3.5. Degenerative radical transfer of xanthates to olefins
A degenerative radical transfer of xanthates to olefins has been developed as a robust synthetic tool for the construction of new C–S bonds in a single operation. This method is featured by not only its capability of introducing a wide range of carbon substituents but also the ability of the installed xanthyl group in being transformed into a variety of functionalities. Chiba70 et al. (Scheme 15) reported an iridium-based, blue LED irradiated protocol for a photoinduced radical addition of xanthates (39) to olefins (40), leading to diverse xanthate adducts (41). This reaction proceeds through a radical chain propagation mechanism via an initiation step involving a triplet-sensitization process of xanthates by an excited iridium-based photocatalyst.
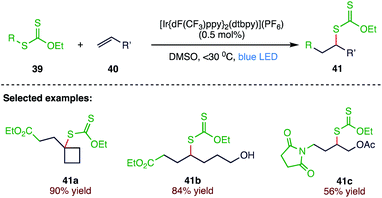 |
| Scheme 15 Degenerative radical transfer of xanthates to olefins. | |
3.6. Trifluoromethylthiolation of aryldiazonium salts
The trifluoromethylthiol group (SCF3) has attracted great attention from both academia and industry due to its special physical and chemical properties. Zhao71 et al. (Scheme 16) reported a visible light photocatalysed trifluoromethylthiolation of aryl amines (44) through the in situ generation of aryldiazonium salts (42) as key intermediates using S-trifluoromethyl 4-methoxylbenzenesulfonothioate (43). The mild reaction conditions and the readily accessible reagents provide a practical protocol to synthesise aryl trifluoromethylthioether (44).
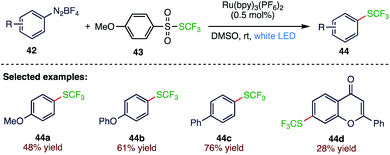 |
| Scheme 16 Trifluoromethylthiolation of aryldiazonium salts. | |
3.7. Synthesis of 4-alkyl/aryl-2-aminothiazoles
Copper is an inexpensive metal that is earth-abundant and readily available. In recent decades, the use of copper catalysts to effect traditional thermal reaction has seen unprecedented growth. Liu72 et al. (Scheme 17) reported a copper catalyzed, blue LED irradiated room-temperature synthesis of 4-alkyl/aryl-2-aminothiazoles (47) from vinyl azides (45) and ammonium thiocyanate (46). The present protocol includes a novel methodology for synthesizing a broad range of 4-alkyl/aryl-2- aminothiazoles via copper-promoted intermolecular cyclization under visible light irradiation. Moreover, the method is distinguished by a broad scope, high yield, low catalyst loading and a mild, operationally simple strategy. This new intermolecular cyclization protocol can be useful in the pharmaceutical exploitation and complete synthesis of natural products having the 2-aminothiazole structural motif.
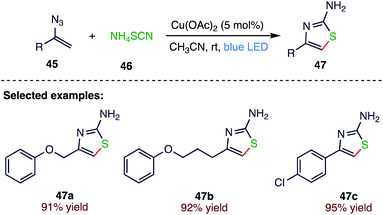 |
| Scheme 17 Synthesis of 4-alkyl/aryl-2-aminothiazoles. | |
3.8. Synthesis of 6-(sulfonylmethyl)phenanthridines
Due to optoelectronic properties and unique biological activities, phenanthridine and its analogues are privileged subunits and have attracted extensive attention in material and medicinal chemistry. Zhou et al.73 (Scheme 18) reported an efficient and simple method for the synthesis of functionalized phenanthridines (50) through a visible-light-mediated tandem sulfonylation/annulation of vinyl azides (48) and sulfonyl hydrazines (49) under mild reaction conditions. In this reaction, simple and commercially available sulfonyl hydrazines emerge as efficient sulfonylating reagents for the formation of new C–S bonds along with C–N bond formation.
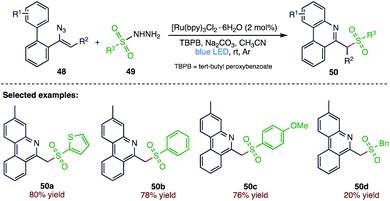 |
| Scheme 18 Synthesis of 6-(sulfonylmethyl)phenanthridines. | |
3.9. Sulfonylation of 4-methylphenols
Sulfone is an important synthon existing widely in a range of natural products and top selling drugs. Several synthetic methods have been proposed for the synthesis of sulfonyl compounds (53) due to their unique applications in organic synthesis and pharmaceuticals. Wu74 et al. (Scheme 19) reported the photocatalysed sulfonylation of benzylic C–H bonds via the three-component reaction of aryldiazonium tetrafluoroborates (52), 4-methylphenols (51) and sodium metabisulfites. In this reaction, inorganic sulphite of sodium metabisulfite is used as a surrogate of SO2. In this visible light irradiated transformation, benzylic C(sp3)–H bond sulfonylation is realized under mild conditions.
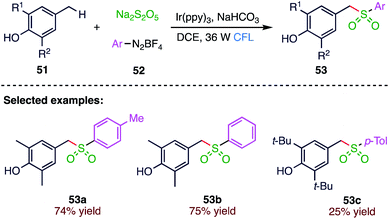 |
| Scheme 19 Sulfonylation of 4-methylphenols. | |
3.10. Chlorosulfonylation of alkynes
Han75 et al. (Scheme 20) reported a photocatalytic redox process for the one-step chlorosulfonylation of alkynes. This novel photoredox catalysed method includes regio- and stereo-selective chlorosulfonylation of alkynes (56) under visible-light irradiation. A wide range of alkynes (54) and sulfonyl chlorides (55) are competent participants in the free-radical mediated reaction to afford structurally diverse vinyl sulfones. The present protocol includes the generation of sulfonyl radical intermediates from easily available organosulfonyl chlorides under mild conditions at room temperature, thus representing an operationally convenient alternative to the reported methodologies.
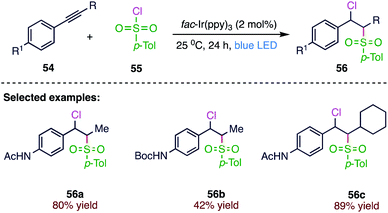 |
| Scheme 20 Chlorosulfonylation of alkynes. | |
4. C–S bond formation without photocatalyst
4.1. Synthesis of aryl sulfides
In recent years, the development of mild and sustainable protocols for carbon–heteroatom bond formation has received considerable attention. Aryl boronates and aryl sulfides have an important role in organic synthesis, catalysis, materials science, and especially in medicinal chemistry. Rueping76 et al. (Scheme 21) reported a green, efficient, photoinduced synthesis of aryl sulfides (59). In this protocol, bench stable arylazo sulfones (57) were used as radical precursors for a photocatalyst and additive-free carbon–heteroatom bond formation under visible light irradiation. During the course of the reaction, these stable and easy to handle aryldiazonium salt derivatives show a high photoreactivity under blue light irradiation. The different aryl derivatives provide products in moderate to good yields.
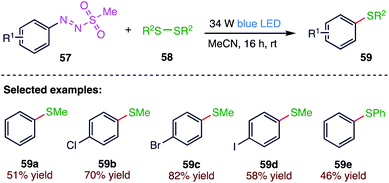 |
| Scheme 21 Synthesis of aryl sulfides. | |
4.2. Coupling of aryldiazosulfones with thiols
Sulfoxides act as highly valuable building blocks, which comprise the key structural motifs of many natural products, biologically active molecules and drug candidates. Wei77 et al. (Scheme 22) reported a facile and efficient visible-light induced oxidative method for the formation of sulfoxides via the oxidative coupling of aryldiazo sulfones (60) with thiols (61) using the O2 in air. The present methodology offers a mild and environmentally benign approach for the library synthesis of sulfoxides (62) in good yields along with favorable functional group tolerance.
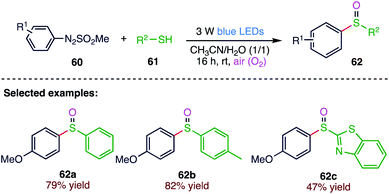 |
| Scheme 22 Coupling of aryldiazosulfones with thiols. | |
4.3. Synthesis of sulfonylated oxindoles
Manolikakes78 et al. (Scheme 23) reported a novel, visible-light mediated three-component synthesis of sulfonylated oxindoles (65) from N-arylacrylamides (64), diaryliodonium salts (63) and sulphur dioxide. This novel methodology allows the facile preparation of pharmacologically relevant oxindole scaffolds under mild reaction conditions in good to excellent yields. This reaction displays a broad tolerance towards functional groups and proceeds in the absence of any catalyst or external photosensitizer. The key step of this process is the in situ formation of sulfonyl radicals from the corresponding aryl radicals and sulphur dioxide. The present protocol includes the visible light-irradiated three component synthesis of sulfonylated oxindoles (68) via the direct incorporation of sulphur dioxide as a key building block for the sulfonyl functionality.
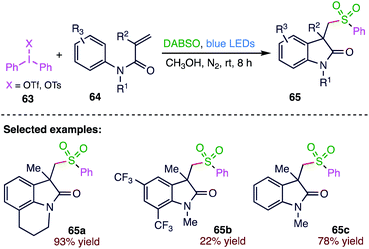 |
| Scheme 23 Synthesis of sulfonylated oxindoles. | |
4.4. Synthesis of 1,3,4-thiadiazines
Singh79 et al. (Scheme 24) reported an expeditious and proficient pH dependent, visible light-induced multicomponent strategy for the synthesis of a highly biologically significant scaffold “1,3,4-thiadiazines” and its derivatives. The significant feature of the present protocol is the utilization of visible light at room temperature to form C–S bonds via the generation of some free radical intermediates followed by intramolecular cyclization without employing harsh reaction conditions. The present protocol includes some other attributes such as being environmentally benign and cost effective, reduced reaction time and possessing adaptability towards a wide range of substrates with good to excellent yield of products (69). This photochemically induced synthetic pathway of 1,3,4-thiadiazines includes fringe benefits in terms of sustainability, operational feasibility, broad range of functional group tolerance and high yields.
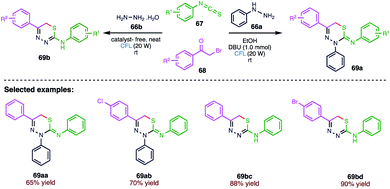 |
| Scheme 24 Synthesis of 1,3,4-thiadiazines. | |
4.5. C–H thiolation
Lang80 et al. (Schemes 25 and 26) reported a visible-light induced, intramolecular C(sp2)–H thiolation without the application of a photosensitizer, metal catalyst or base. In this protocol, photocatalysed, intramolecular C–S bond formation of aromatic substrates takes place; thioamide derivatives (70) in the presence of TEMPO smoothly cyclize to give benzothiazoles (71) via two RHAT events. This cyclization is compatible with a wide range of functional groups and will therefore be applicable for the generation of various aromatic heterocyclic compounds.
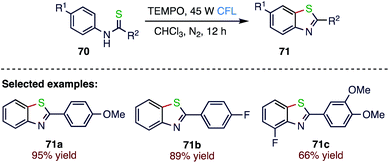 |
| Scheme 25 C–H thiolation. | |
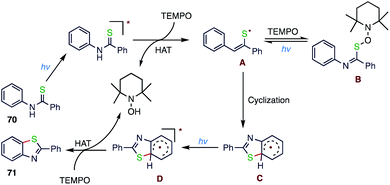 |
| Scheme 26 A plausible mechanism for C–H thiolation. | |
4.6. Synthesis of 3-sulfonated quinoline derivatives
Li81 et al. (Scheme 27) reported a visible-light-mediated oxidative cyclization of N-propargyl anilines (72) with sulfinic acids (73) in the absence of an external photocatalyst. This protocol provides a simple and atom economic way to synthesize 3-sulfonated quinolones (74) with good functional group tolerance and excellent product yields under mild reaction conditions using molecular oxygen in air as the oxidant.
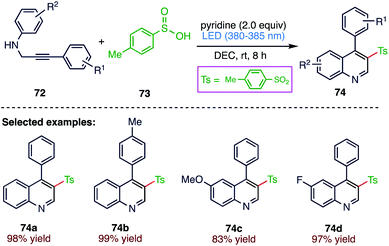 |
| Scheme 27 Synthesis of sulfonated quinoline derivatives. | |
4.7. Synthesis of 2-perfluoroalkylbenzothiazoles
In recent years, fluorine-containing organic compounds have attracted considerable attention in the fields of pharmaceuticals, agrochemicals, and materials science. Yu82 et al. (Scheme 28) reported a novel and practical blue-light irradiated fluoroalkyl radical-initiated cascade reaction to access diverse 2-fluoroalkyl-benzothiazoles by reacting commercially available fluoroalkyl radical sources, including perfluoroalkyl iodide (76) (ICnF2n+1, n = 3–8, 10), ICF(CF3)2, ICF2COOEt, ICF2CF2Cl or ICF2CF2Br, TMEDA, and structurally simple 2-isocyanoaryl thioethers (75) in THF under nitrogen atmosphere. Moreover, this one-pot protocol could be easily expanded to access a number of novel and biologically potential 2-fluoroalkylbenzoselenazoles starting from 2-isocyanophenyl)(methyl)selane, perfluoroalkyl iodides (ICnF2n+1, n = 3–8) or ICF2COOEt and TMEDA. A significant advantage of this photochemical strategy is that it proceeds in the absence of externally added photocatalysts, particularly the expensive and potentially toxic metal complexes frequently required by many previously mentioned photochemical reactions; therefore, this protocol exhibits a remarkably benign and eco-friendly feature.
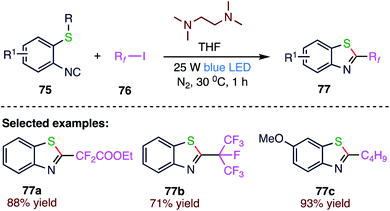 |
| Scheme 28 Synthesis of 2-perfluoroalkylbenzothiazoles. | |
4.8. Synthesis of sulfonylated coumarins
Manolikakes83 et al. (Scheme 29) reported a visible-light induced three component synthesis of sulfonylated coumarins (80) using sulphur dioxide as a key building block. This novel method enables the synthesis of biologically important sulfone containing coumarins under very mild reaction conditions in moderate to good yields with a high tolerance towards functional groups. This process is based on the in situ generation of sulfonyl radicals from aryl radicals using sulphur dioxide as the source. In this strategy, non-toxic and environmentally benign diaryliodonium salts serve as radical precursors, and unsymmetrical iodonium salts can be used as highly chemoselective aryl transfer reagents. This catalyst-free transformation is solely driven by visible light, thereby enabling a new opportunity for the sustainable synthesis of sulfones.
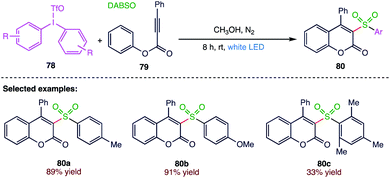 |
| Scheme 29 Synthesis of sulfonylated coumarins. | |
5. Conclusions
In conclusion, the study of novel and efficient visible light-mediated advanced reaction systems and the corresponding synthetic methodologies represent an essential developing field to improve the performance of sulphur centered radicals. The C–S bond formations and functionalizations involving sulphur-centered radical mechanisms have been carried out with the addition of organic dyes, transition-metals as well as without photocatalysts. Considering their great potential for synthetic applications, these synthetic strategies will help to promote continued interest in visible light induced photoredox catalysed organic transformations and further functionalization of sulphur centered radicals, which can be envisaged in the near future.
Conflicts of interest
There are no conflicts to declare.
References
-
(a) C. Shen, P. Zhang, Q. Sun, S. Bai, T. S. Andy Hor and X. Liu, Chem. Soc. Rev., 2015, 44, 291 RSC;
(b) C. Xia, Z. Wei, C. Shen, J. Xu, Y. Yang, W. Su and P. Zhang, RSC Adv., 2015, 65, 52588 RSC;
(c) Q. Lu, J. Zhang, F. Wei, Y. Qi, H. Wang, Z. Liu and A. Lei, Angew. Chem. Int. Ed., 2013, 52(28), 7156 CrossRef CAS PubMed;
(d) J. Xu, C. Shen, X. Zhu, P. Zhang, M. J. Ajitha, K. W. Huang, Z. An and X. Liu, Chem.–Asian J., 2016, 11, 882 CrossRef CAS PubMed;
(e) B. N. Rocke, K. B. Bahnck, M. Herr, S. Lavergne, V. Mascitti, C. Perreault, J. Polivkova and A. Shavnya, Org. Lett., 2014, 16, 154 CrossRef CAS.
- A. Barattucci, M. Aversa, A. Mancuso, T. Sa lerno and P. Bonaccorsi, Molecules, 2018, 23, 1030 CrossRef PubMed.
- J. D. Neuhaus, R. Oost, J. Merad and N. Maulide, Top. Curr. Chem., 2018, 376, 15 CrossRef PubMed.
- K. A. Scott and J. T. Njardarson, Top. Curr. Chem., 2018, 376, 5 CrossRef PubMed.
- M. Feng, B. Tang, S. H. Liang and X. Jiang, Curr. Top. Med. Chem., 2016, 16, 1200 CrossRef CAS PubMed.
- N. W. Liu, S. Liang and G. Manolikakes, Synthesis, 2016, 48, 1939 CrossRef CAS.
- B. K. Peters, T. Zhou, J. Rujirawanich, A. Cadu, T. Singh, W. Rabten, S. Kerdphon and P. G. Andersson, J. Am. Chem. Soc., 2014, 136, 16557 CrossRef CAS PubMed.
- M. Feng, B. Tang and X. Jiang, Curr. Top. Med. Chem., 2016, 16, 1200 CrossRef CAS PubMed.
- X. Gao, X. Pan and Y. Li, Org. Lett., 2015, 17, 1038 CrossRef CAS PubMed.
- A. A. Vieira, J. B. Azeredo and A. L. Braga, J. Org. Chem., 2015, 80, 2120 CrossRef CAS PubMed.
- H. Wang, Q. Lu and A. Lei, Angew. Chem., Int. Ed., 2016, 55, 1094 CrossRef CAS PubMed.
- S. F. Zhou, X. Pan and J. P. Zou, J. Org. Chem., 2015, 80, 3682 CrossRef CAS PubMed.
- X. R. Wang and F. Chen, Tetrahedron, 2011, 67, 4547 CrossRef CAS.
- W. Nakanishi, Hypervalent Chalcogen Compounds, in Handbook of Chalcogen Chemistry: New Perspectives in Sulphur, Selenium and Tellurium, ed. Devillanova F. A., Royal Society of Chemistry, London, 2006 Search PubMed.
- A. El-Awa, M. N. Noshi, X. M. du Jourdin and P. L. Fuchs, Chem. Rev., 2009, 109, 2315 CrossRef CAS PubMed.
- H. Liu and X. Jiang, Chem.–Asian J., 2013, 8, 2546 CrossRef CAS PubMed.
- Z. Qiao and X. Jiang, Org. Biomol. Chem., 2017, 15, 1942 RSC.
- K. L. Dunbar, D. H. Scharf, A. Litomska and C. Hertweck, Chem. Rev., 2017, 117, 5521 CrossRef CAS PubMed.
- A. B. Smith and C. M. Adams, Acc. Chem. Res., 2004, 37, 365 CrossRef CAS PubMed.
- L. Liu, G. Wang, J. Jiao and P. Li, Org. Lett., 2017, 19, 6132 CrossRef CAS PubMed.
- D. Seebach and E. J. Corey, J. Org. Chem., 1975, 40, 231 CrossRef CAS.
- R. Sasson, A. Hagooly and S. Rozen, Org. Lett., 2003, 5, 769 CrossRef CAS PubMed.
- R. Mozingo, D. E. Wolf, S. A. Harris and K. Folkers, J. Am. Chem. Soc., 1943, 65, 1013 CrossRef CAS.
- T. Y. Luh, Acc. Chem. Res., 1991, 24, 257 CrossRef CAS.
- J. R. Vale, T. Rimpilainen, E. Sievanen, K. Rissanen, C. A. M. Afonso and N. R. Candeias, J. Org. Chem., 2018, 83, 1948 CrossRef CAS PubMed.
- J. W. Tucker and C. J. R. Stephenson, J. Org. Chem., 2012, 77(4), 1617 CrossRef CAS PubMed.
- D. R. Heitz, K. Rizwan and G. A. Molander, J. Org. Chem., 2016, 81(16), 7308 CrossRef CAS PubMed.
- F. X. Felpin and S. Sengupta, Chem. Soc. Rev., 2019, 48, 1150 RSC.
- H. Chen, L. Guo and S. Yu, Org. Lett., 2018, 20(19), 6255 CrossRef CAS PubMed.
- X. Sala, I. Romero, M. Rodriguez, L. Escriche and A. Llobet, Angew. Chem., Int. Ed., 2009, 48(16), 2842 CrossRef CAS PubMed.
- D. Mandler and I. Willner, J. Am. Chem. Soc., 1984, 106(18), 5352 CrossRef CAS.
- O. Ishitani, S. Yanagida, S. Takamuku and C. Pac, J. Org. Chem., 1987, 52(13), 2790 CrossRef CAS.
- A. Inagakia and M. Akita, Coord. Chem. Rev., 2010, 254, 1220 CrossRef.
- C. K. Prier, D. A. Rankic and D. W. C. MacMillan, Chem. Rev., 2013, 113(7), 5322 CrossRef CAS PubMed.
- D. A. Nicewicz and T. M. Nguyen, ACS Catal., 2014, 4(1), 355 CrossRef CAS.
- J. Xie, H. Jina, P. Xu and C. Zhu, Tetrahedron Lett., 2014, 55(1), 218 Search PubMed.
- X. Lang, X. Chen and J. Zhao, Chem. Soc. Rev., 2014, 43(1), 473 RSC.
- J. Hu, J. Wang, T. H. Nguyen and N. Zheng, Beilstein J. Org. Chem., 2013, 9, 1977 CrossRef PubMed.
- D. Rovelli, M. Fagnoni and A. Albini, Chem. Soc. Rev., 2013, 42(1), 97 RSC.
- T. P. Yoon, M. A. Ischay and J. J. Du, Nat. Chem., 2010, 2(7), 527 CrossRef CAS PubMed.
- X. J. Yang, B. Chen, L. Q. Zheng, L. Z. Wu and C. H. Tung, Green Chem., 2014, 16, 1082 RSC.
- K. Fidaly, C. Ceballos, A. Falguières, M. S. I. Veitia, A. Guy and C. Ferroud, Green Chem., 2012, 14, 1293 RSC.
- D. T. Yang, Q. Y. Meng, J. J. Zhong, M. Xiang, Q. Liu and L. Z. Wu, Eur. J. Org. Chem., 2013, 33, 7528 CrossRef.
- Y. Q. Zou, J. R. Chen, X. P. Liu, L. Q. Lu, R. L. Davis, K. A. Jørgense and W. J. Xiao, Angew. Chem., Int. Ed., 2012, 51, 784 CrossRef CAS PubMed.
- D. P. Hari and B. König, Org. Lett., 2011, 13(15), 3852 CrossRef CAS PubMed.
- M. Neumann, S. Füldner, B. König and K. Zeitler, Angew. Chem., Int. Ed., 2011, 50(4), 951 CrossRef CAS PubMed.
- V. Rey, S. M. S. Catro, J. E. Arguello and A. B. Peñéñory, Tetrahedron Lett., 2009, 50(33), 4720 CrossRef CAS.
- V. Srivastava, P. K. Singh and P. P. Singh, Croat. Chem. Acta, 2014, 87(2), 91 CrossRef.
- V. Srivastava, P. K. Singh and P. P. Singh, Croat. Chem. Acta, 2015, 88(1), 59 CrossRef CAS.
- V. Srivastava, P. K. Singh and P. P. Singh, Croat. Chem. Acta, 2015, 88(3), 227 CrossRef CAS.
- V. Srivastava, P. K. Singh and P. P. Singh, Asian J. Chem., 2016, 8(10), 2159 CrossRef.
- V. Srivastava and P. P. Singh, RSC Adv., 2017, 7, 31377 RSC.
- V. Srivastava, P. K. Singh, S. Kanaujia and P. P. Singh, New J. Chem., 2018, 42, 688 RSC.
- P. K. Singh, P. P. Singh and V. Srivastava, Croat. Chem. Acta, 2018, 91(3), 383 CrossRef.
- V. Srivastava, P. K. Singh and P. P. Singh, Tetrahedron Lett., 2019, 60, 40 CrossRef CAS.
- V. Srivastava, P. K. Singh and P. P. Singh, Tetrahedron Lett., 2019, 60, 1333 CrossRef CAS.
- V. Srivastava, P. K. Singh and P. P. Singh, Tetrahedron Lett., 2019, 60, 151041 CrossRef.
- V. V. Levin and A. D. Dilman, J. Org. Chem., 2019, 84(12), 8337 CrossRef CAS PubMed.
- L. Zhang, J. Zhu, J. Ma, L. Wu and W. H. Zhang, Org. Lett., 2017, 19, 6308 CrossRef CAS PubMed.
- K. Choudhuri, M. Pramanik and P. Mal, Eur. J. Org Chem., 2019, 30, 4822 CrossRef.
- Y. Gao, Y. Liu and J. P. Wan, J. Org. Chem., 2019, 84(4), 2243 CrossRef CAS PubMed.
- X. Wang, M. Yang, W. Xie, X. Fan and J. Wu, Chem. Commun., 2019, 55, 6010 RSC.
- D. Sunab, K. Yinab and R. Zhang, Chem. Commun., 2018, 54, 1335 RSC.
- L. Yong Xie, T. G. Fang, J. X. Tan, B. Zhang, Z. Cao, L. H. Yanga and W. M. He, Green Chem., 2019, 21, 3858 RSC.
- S. Ye, X. Li, W. Xie and J. Wu, Asian J. Org. Chem., 2019, 8(6), 893 CrossRef CAS.
- Y. Ran, Q. Y. Lin, X. H. Xu and F. L. Qing, J. Org. Chem., 2017, 82, 7373 CrossRef CAS PubMed.
- G. Bogonda, D. V. Patil, H. Y. Kim and K. Oh, Org. Lett., 2019, 21(10), 3774 CrossRef CAS PubMed.
- R. Xu and C. Cai, Chem. Commun., 2019, 55, 4383 RSC.
- Y. Li, J. Cai, M. Hao and Z. Li, Green Chem., 2019, 21, 2345 RSC.
- A. Kaga, X. Wu, J. Y. J. Lim, H. Hayashi, Y. Lu, E. K. L. Yeow and S. Chiba, Beilstein J. Org. Chem., 2018, 14, 3047 CrossRef CAS PubMed.
- X. Zhao, X. Zheng, M. Tian, Y. Tong, B. Yang, X. Wei, D. Qiua and K. Lub, Org. Chem. Front., 2018, 5, 2636 RSC.
- W. L. Lei, T. Wang, K. W. Feng, L. Z. Wu and Q. Liu, ACS Catal., 2017, 7(11), 7941 CrossRef CAS.
- L. L. Mao, L. X. Quan, X. H. Zhu, C. B. Ji, A. X. Zhou, F. Chen and D. G. Zheng, Synlett, 2019, 30(08), 955 CrossRef CAS.
- X. Gong, J. Chen, L. Lai, J. Cheng, J. Sun and J. Wu, Chem. Commun., 2018, 54, 11172 RSC.
- P. Chakrasali, K. Kim, Y. S. Jung, H. Kim and S. B. Han, Org. Lett., 2018, 20(23), 7509 CrossRef CAS PubMed.
- L. Blanka, M. Fagnoni, S. Prottib and M. Rueping, Synthesis, 2019, 51(05), 1243 CrossRef.
- Q. Liu, L. Wang, H. Yue, J. S. Li, Z. Luod and W. Wei, Green Chem., 2019, 21, 1609 RSC.
- N. W. Liu, Z. Chen, An. Herbert, H. Ren and G. Manolikakes, Eur. J. Org Chem., 2018, 41(8), 5725 CrossRef.
- A. Mishra, P. Rai, J. Singh and J. Singh, ChemistrySelect, 2018, 3, 8408 CrossRef CAS.
- Z. M. Xu, H. X. Li, D. J. Young, D. L. Zhu, H. Y. Li and J. P. Lang, Org. Lett., 2019, 21, 237 CrossRef CAS PubMed.
- Y. Zhang, W. Chen, X. Jia, L. Wang and P. Li, Chem. Commun., 2019, 55, 2785 RSC.
- Y. Liu, X. L. Chen, K. Sun, X. Y. Li, F. L. Zeng, X. C. Liu, L. B. Qu, Y. F. Zhao and B. Yu, Org. Lett., 2019, 21(11), 4019 CrossRef CAS PubMed.
- Z. Chen, N. W. Liu, M. Bolte, H. Ren and G. Manolikakes, Green Chem., 2018, 20, 3059 RSC.
|
This journal is © The Royal Society of Chemistry 2020 |
Click here to see how this site uses Cookies. View our privacy policy here.