DOI:
10.1039/D0RA02848G
(Paper)
RSC Adv., 2020,
10, 21629-21635
A sensitive OFF–ON–OFF fluorescent probe for the cascade sensing of Al3+ and F− ions in aqueous media and living cells†
Received
28th March 2020
, Accepted 26th May 2020
First published on 5th June 2020
Abstract
A simple Schiff-base ligand 2-hydroxy-1-naphthaldehyde semicarbazone (HNS) was synthesized and characterized. Based on the combined effect of inhibition of CH
N isomerization and chelation-enhanced fluorescence (CHEF), HNS functions as a fluorescence “turn on” sensor for Al3+ in buffered aqueous media. Based on the strong affinity of Al3+ to F− ions, the in situ generated Al3+–HNS complex can also be utilized as an effective chemosensor for F− sensing by metal displacement approach, ensuing quenching of fluorescence by the reversible return of HNS from Al3+–HNS complex. Thus a method using a single probe for the detection of both Al3+ and F− ions is developed. The system exhibits high selectivity and sensitivity for Al3+ and F− ions and the detection limits were found to be as low as 6.75 × 10−8 M and 7.89 × 10−7 M, respectively. Furthermore, the practical applicability of this probe has been examined in living cells.
1. Introduction
Aluminum is the most abundant metal in the Earth's crust and is extensively used in every sphere of life from utensils to medicine. But aluminum in excessive amounts not only hampers plant growth but damages the human nervous system and has been reported to induce Alzheimer's disease, Parkinson's disease and amyotrophic lateral sclerosis, etc.1 Therefore, detection of Al3+ ions in natural environment and living organisms is of vital importance for human health. In this context, the development of fluorescence based probes attracted more attention because of the simplicity and high sensitivity of fluorescence assays. However, the lack of spectroscopic characteristics and poor coordination ability of Al3+ (ref. 2) make it very challenging to develop a highly selective sensing system for Al3+.
Up to now, several fluorescent probes for Al3+ have been designed by various workers.3–19 And the structures of most fluorescent probes for Al3+ contain nitrogen–oxygen-rich coordination environments which provide a hard-base environment for the hard-acid Al3+. These probes, however, have some limitations such as poor water solubility and tedious synthetic procedure, which limited their practical applications. Thus, only few examples of Al3+ probes can be used for cell imaging and biological samples.5,8,9,12–15 Overall, it is still highly desirable to develop novel fluorescent probes for Al3+ which satisfies several parameters viz. easy and economical synthetic procedure, moderate water solubility and being suitable for cell imaging studies.
Moderate fluoride for human has good performance in the treatment of osteoporosis and the prevention of dental caries. However, excess presence of for human body may lead to dental fluorosis, skeletal fluorosis, osteoporosis, and osteosclerosis.20 Thus, the development of an efficient F− fluorescent sensor is quite important.21–23 Interestingly, emissive probe + Al3+ complexes can serve as anion sensors, especially to F−.24–29 And these sensors that are capable of detecting Al3+ and F− sequentially by fluorescence OFF–ON–OFF signaling response are highly effective when compared with the one-to-one probes.30–32
With these considerations in mind, we herein report an easily synthesizable Schiff base receptor 2-hydroxy-1-naphthaldehyde semicarbazone (HNS), which commendably supports the idea of the sequential sensing property for Al3+ and F− in aqueous media and live cells. 2-Hydroxynaphthalene was selected as a signaling moiety for its low fluorescence quantum yield,33 short fluorescence lifetime,34 good binding sites and low cost. The binding of Al3+ with the phenolic –OH group and Schiff base nitrogen atoms of HNS would inhibit the cis–trans isomerization around C
N and increase the rigidity of the molecular assembly to produce significant fluorescence enhancement, which shows a selective sensing property for Al3+ in buffered aqueous media. Further, the formed Al3+–HNS complex can be utilized for the sensitive detecting of fluoride owing to the strong binding affinity of Al3+ for F− ions.35 Hence, the gradual addition of F− ions into a mixture of HNS and Al3+ ions releases HNS, and shows fluorescence quenching. Thus, a single-probe sensory system was developed to detect both Al3+ cation and F− anion with good detection reversibility, which would greatly decrease the cost for detection. What's more, detection of intracellular Al3+ and F− in live SiHa cells by fluorescence imaging has been demonstrated in this study.
2. Experimental
2.1 Chemicals and instrumentation
2-Hydroxy-1-naphthaldehyde (98%) was purchased from Energy Chemical (Shanghai, China). Aminourea hydrochloride was purchased from Aladdin Reagent Co. (Shanghai, China). All the other chemical reagents were of analytical grade and used as received without further purification. Stock solutions of metal ions were prepared by dissolving their nitrates in water and stock solutions of anions were prepared from the corresponding sodium salts. Hexamethylenetetramine–HCl buffer solution of pH 5.3 was prepared by mixing appropriate ratios of 0.01 mol L−1 hexamethylenetetramine and 0.1 mol L−1 HCl solutions. Double distilled water was used throughout. All the solutions have been stocked in the plastic centrifugal tubes.
The UV absorption spectra were recorded on a Puxi TU-1901 UV-vis absorption spectrophotometer (China). Fluorescence measurements were performed on a LS-55 spectrofluorimeter (PerkinElmer, USA). The samples were excited at 390 nm. The excitation and emission slits were set at 6 and 4 nm, respectively. IR spectra were taken as KBr pellets on a TENSOR II infrared spectrometer (Bruker, Germany). 1H-NMR spectra were recorded on a DRX-300 spectrometer (Fällenden, Switzerland). The pH measurements were carried out on a pHS-3C acidometer (Shanghai Precision & Scientific Instrument Co., Ltd, China). High resolution mass spectra (HRMS) were obtained on a Bruker micrOTOF-Q III mass spectrometer. The fluorescence intracellular images were obtained using an Olympus FV1000 confocal microscope (Tokyo, Japan) with a 40× objective (excited at 405 nm).
2.2 Synthesis of 2-hydxoxy-1-naphthaldehyde semicarbazone (HNS)
Scheme 1 shows the facile one step synthesis of the ligand HNS. Aminourea hydrochloride (259 mg, 2.32 mmol) was dis-solved in 8 mL of ethanol. The solution was neutralized to pH 7.0 with 1.0 M of NaOH and then was mixed with the ethanol solution of 2-hydroxy-1-naphthaldehyde (200 mg, 1.16 mmol). The mixture was heated under reflux for 4 h and then cooled to room temperature. Saturated sodium chloride solution was added dropwise and the precipitated solid was filtered off, washed with cold water and dried under vacuum and recrystallized from ethanol to give the product as a light-yellow solid (170 mg, 64% yield). IR νmax (KBr): 3450, 3350, 1670, 1602, 1431, 1189 cm−1. 1H NMR (DMSO-d6) δ: 6.37 (s, 2H, NH2), 7.20 (d, 1H, ArH), 7.34 (t, 1H, ArH), 7.52 (t, 1H, ArH), 7.85 (t, 2H, ArH), 8.38 (d, 1H, ArH), 8.86 (s, 1H, NH), 10.22 (s, 1H, CH
N), 11.24 (s, 1H, OH). 13C NMR (DMSO-d6): 161.51, 161.31, 145.24, 136.86, 136.74, 134.12, 133.38, 132.95, 128.72, 127.51, 123.87, 115.25. HRMS (ESI): calcd for C12H12N3O2 (HNS + H) 230.0930, found 230.0927.
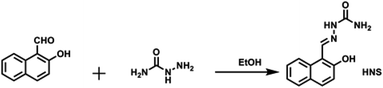 |
| Scheme 1 The synthesis of probe HNS. | |
2.3 Cell imaging study
In vitro experiments were performed using SiHa cells. SiHa cells were cultured in DMEM medium, which was supplemented with 10% fetal bovine serum (FBS) in an atmosphere of 5% CO2 at 37 °C. Immediately before the experiments, the cells were incubated with 50 μL HNS (10−3 M, ethanol solution) in 1 mL 0.1 M sterile PBS buffer for 30 min at room temperature. After incubation, the cells were washed with PBS buffer and incubated with Al3+ (100 μM) for additional 10 min at room temperature. Finally, the cells treated with HNS and Al3+ were further incubated with NaF (500 μM) for another 10 min. The fluorescence intracellular images were obtained using an Olympus FV1000 confocal microscope (Tokyo, Japan) with a 40× objective (excited at 405 nm).
3. Results and discussion
3.1 Spectral characteristics of HNS and HNS–Al3+
HNS could be dissolved in water when 10% (v/v) of ethanol was added. So, the spectroscopic properties of HNS were investigated in an ethanol–water (1
:
9, v/v) solution at pH 5.3. As shown in Fig. 1a, the absorption spectrum of HNS exhibits structured absorption spectrum corresponding to the π–π* transitions of naphthalene unit observed in naphthalene derivatives.36 Upon addition of increasing amounts of Al3+, the longest-wavelength absorption band red shifted from 348 nm to 381 nm. The presence of two clear isosbestic points (330 and 363 nm) indicates the formation of a complex between HNS and Al3+.
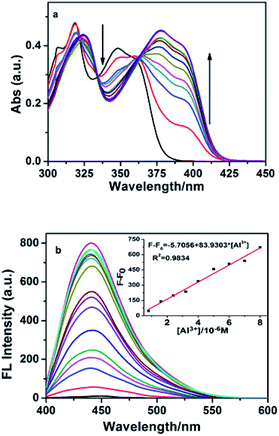 |
| Fig. 1 (a) UV/vis spectra of HNS (40 μM) in the presence of different concentrations of Al3+ (0–10 equiv.) and (b) fluorescence titration spectra of HNS (4 μM) upon the addition of Al3+ ions (0–8 equiv.) in EtOH/H2O (1 : 9, v/v, pH 5.3). The inset in (b) shows the fluorescence intensity of HNS as a function of Al3+ ion concentration) indicates the formation of a complex between HNS and Al3+. | |
Fig. 1b shows the changes in the emission intensity of HNS upon gradual increase of [Al3+]. HNS shows very weak broad emission in the range from 400 nm to 550 nm with a maximum at 449 nm on excitation at 390 nm. Upon addition of Al3+, HNS shows large fluorescence enhancement, accompanied with a blue-shift of emission maxima from 449 nm to 441 nm. The results suggest that Al3+ most likely forms stable fluorescent complex with HNS. Schiff-bases usually have low emission due to the isomerization reaction around the imine linkage C
N.37 This isomerization, however, is inhibited by the binding of Al3+ ions leading to fluorescence switching of the receptor. In addition, the chelation of HNS with Al3+ increases the rigidity of the molecular assembly, resulting in enhancement of emission intensity ascribed as CHEF.
3.2 Fluorescence sensing of Al3+
Optimization of pH on the efficiency of the sensor was essential. Fluorescence pH titrations were carried out for this purpose. Fig. 2 clearly demonstrates that at the pH range from 4.2 to 5.4, the HNS–Al3+ system showed maximum emission intensity but decrease outside this range. Most plausibly, breakage of imine linkage of HNS at too low pH prevents coordination of Al3+ while at high pH values OH− may succeed against HNS in binding to Al3+. In order to obtain a higher signal-to-noise, pH = 5.3 with hexamethylenetetramine–hydrochloric acid buffer solution was chosen throughout the experiment.
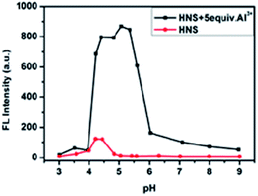 |
| Fig. 2 Effect of pH on fluorescence intensity of the Al3+–HNS complex in EtOH/H2O (1 : 9, v/v), [HNS] = 4 μM, [Al3+] = 20 μM. | |
Selectivity of HNS as the fluorescence chemosensor for Al3+ was studied in the presence of various competing metal ions. Under the same conditions as used above for Al3+, we tested the fluorescence responses of HNS toward other metal ions such as Pb2+, Na+, Cd2+, K+, Mg2+, Cu2+, Co2+, Zn2+, Ni2+, Hg2+, Ca2+, Fe3+ and In3+. As shown in Fig. 3a, significant spectral changes were observed only in the case of Al3+. In contrast, treatment with other metal ions resulted in no significant changes, demonstrating that HNS is highly selective for Al3+ over competing metal ions. Furthermore, it was observed that the emission intensity of the Al3+–HNS complex was not remarkably affected by most coexistent metal ions except Cu2+ and Fe3+ (Fig. 3b). Cu2+ showed complete quenching in fact, where luminescence was even less than that of HNS alone. A similar phenomenon was previously reported by Jang et al.38 Thus HNS can be applied as an effective fluorescent sensor for Al3+ in the presence of most competing metal ions.
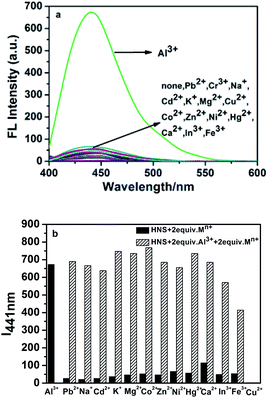 |
| Fig. 3 (a) Fluorescence spectra changes of HNS (4 μM) in the presence of various metal ions (8 μM) in EtOH/H2O (1 : 9, v/v, pH 5.3). (b) Fluorescence intensities of HNS (4 μM) at 441 nm (I441 nm) upon addition of Al3+ in the presence of interfered metal ions. | |
Based on the fluorometric spectra studies, a calibration curve was constructed by plotting the values of F − F0 against Al3+ concentration (inset of Fig. 1b). A good linear relationship between F − F0 and the concentration of Al3+ could be obtained in the range of 0.8–8 μM (R2 = 0.9834). The limit of detection was measured to be 6.75 × 10−8 M (signal to noise ratio of 3
:
1), which is satisfactory to the Al3+ detection in drinking water within World Health Organization (WHO) limit (200 μg L−1, 7.41 μM).39 In terms of sensitivity, the detection limit of the proposed method is better than or comparable to those of previously reported Schiff base probes for Al3+ detection.8,9,15 The relative standard deviation for five repeated measurements of 8.0 × 10−6 M of Al3+ was 1.08%, which illustrated that the response of HNS toward Al3+ was highly reproducible. It should be noted that this switch on sensing process could be easily observed by the naked eye. As shown in Fig. 7, HNS alone only exhibited very weak fluorescence, but gave a strong blue fluorescence in the presence of Al3+.
3.3 Binding mode of HNS with Al3+
A Job plot obtained from emission data showed a 2
:
1 stoichiometry of the complex formed between HNS and Al3+ (Fig. S4a†), which was corroborated by the mass spectra of Al3+–HNS complex. The HRMS spectrum showed a peak at m/z 483.1361 corresponding to 2HNS + Al3+–2H+ [calcd, m/z: 483.1345] (Fig. 4), indicating that the binding of Al3+ to HNS induced deprotonation of the phenolic –OH group of HNS. Based on a 2
:
1 binding mode, the association constant (K) was evaluated from the Al3+ ions titration curve (Fig. S4b†) using the modified Benesi–Hildebrand method19 and was found to be 1.14 × 104 M−1.
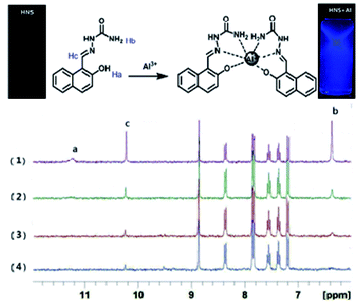 |
| Fig. 4 The ESI-MS spectra of Al3+–HNS complex. | |
Further evidence for the Al3+ coordination was obtained from the FT-IR and NMR spectral studies. In the IR spectrum of Al3+–HNS complex shown in Fig. S5,† the sharp peaks at 3450 cm−1 and 3350 cm−1 may be attributed to N–H stretching of –CONH2 group of HNS. On co-ordination with Al3+ ions, these peaks became a broad band, indicating that the primary amide participated in the binding with Al3+. In addition, the stretching vibration band of C
N at 1603 cm−1 shifted to a lower frequency by 31 cm−1, clearly indicating the coordination of imine nitrogen atom of HNS to aluminium ion.
1H NMR spectroscopy was used to ascertain the binding mode of HNS to Al3+. 1H NMR spectra of HNS were recorded in DMSO-d6 with increasing concentrations of Al3+ (as its nitrate salt). Significant spectral changes were observed as shown in Fig. 5. After addition of Al3+, the Ha protons of the phenolic hydroxyl progressively disappeared, suggesting the occurrence of deprotonation during the coordination to metal ions. The amino proton peak (Hb) at around 6.37 ppm became broader, indicating the coordination of the amino nitrogen to Al3+. Meanwhile, the imine proton peak (Hc) at around 10.22 ppm became less intense and slightly downfield shifted by 0.02 ppm, supporting the coordination of the imine nitrogen to Al3+. The overall changes in 1H NMR spectra indicate the complexation between Al3+ and HNS through amino-N, azomethine-N and phenolic-OH. Based on the Job's plot, IR spectra, mass spectra and NMR titration, we proposed a rational coordinated mode that aluminium ion is hexa-coordinated with two tridentate ligand HNS shown in Fig. 5.
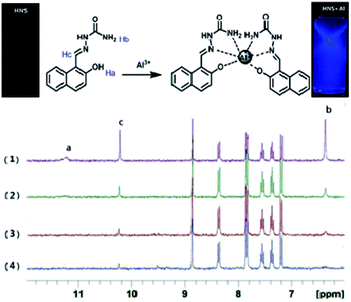 |
| Fig. 5 Top: Binding mode of Al3+–HNS system. Bottom: 1H NMR spectra of HNS in the presence of different concentrations of Al (NO3)3·9H2O in d6-DMSO: (1) HNS only; (2) HNS + Al3+ (0.25 equiv.) and (3) HNS + Al3+ (0.5 equiv.) (4) HNS + Al3+ (1 equiv.). | |
3.4 Fluorescence sensing of F−
The sensor Al3+–HNS for fluoride detection was prepared in situ by addition of 2.0 equiv. of Al3+ to C2H5OH/H2O (1
:
9, v/v) solution of HNS. The F− sensing property of Al3+–HNS was then evaluated by fluorescence titration experiments and the results were depicted in Fig. 6. As shown in Fig. 6a, the fluorescent intensity at 441 nm can be significantly quenched by F− ions. The quenching effect of F− on the fluorescence emission of the system is concentration dependent. There is a good linear relationship (R2 = 0.9957) between the fluorescence intensity and F− concentration in the range of 0.8–20 μM. Thus, probe HNS could not only act as a sensor for Al3+ but also for F− in a successive manner.
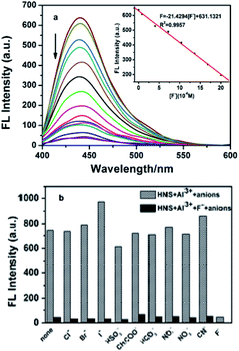 |
| Fig. 6 (a) Fluorescent emission spectra of Al3+–HNS in the presence of different concentrations of F− in ethanol/water = 1 : 9 at pH 5.3. [HNS] = 4 × 10−6 M, [Al3+] = 8 × 10−6 M, and (b) competitive signaling of fluoride ions by Al3+–HNS in the absence (the twill) and presence (the black) of other anions as background in ethanol/water = 1 : 9 (pH = 5.3). [HNS] = 4 × 10−6 M, [anions] = 8 × 10−5 M. | |
Interferences from common anions have also been studied under the same conditions as used above for F−. Out of the tested anions, significant quenching was observed only in case of fluoride (Fig. 6b). Furthermore, the other coexistent anions had a small and negligible effect on the emission intensity of the system, suggesting the high selectivity of the Al3+–HNS ensemble for F− over other anions. The detection limit of Al3+–HNS complex as a fluorescent sensor for the analysis of F− was obtained from a plot of fluorescence intensity as a function of the concentration of the different amounts of F− added. It was found that Al3+–HNS has a detection limit of 7.89 × 10−7 M for F−, which is much lower than the maximum contaminant level defined by the U.S. Environmental Protection Agency (4.0 mg L−1, 211 μM).40
This phenomenon can be supposed that the Al3+ has been captured by F− ions from the [Al3+–HNS] complex to form a new more stable complex AlF3 and resulted in the release of free HNS (Scheme 2). To elucidate the nature of the strong binding of F− to Al3+, the UV-vis spectra, 1H NMR spectra and HRMS experiments have been conducted respectively. As shown in Fig. 7, toward adding F−, the characteristic band at 381 nm of [Al3+–HNS] complex is disappeared, while the absorption response is recovered to the original state of free HNS. The 1H NMR spectrum of [Al3+–HNS] complex has been investigated in absence and in presence of Al3+ in DMSO-d6 (Fig. S6†). The broader amino proton peak (Hb) at around 6.37 ppm became sharp and the imine proton peak (Hc) at around 10.22 ppm became recovered, indicating the dissociation of the Al3+ to HNS after adding F−. This was further confirmed by HRMS studies of HNS + Al(NO3)3 (2.0 equiv.) + NaF (8.0 equiv.) (Fig. S7†). The peak of 2HNS + Al3+–2H+ [calcd, m/z: 483.1345] at m/z = 483.1355 became very weak and the peak at m/z = 230.0924 corresponded to [HNS + H+] (230.0930) displayed a prominent peak, which further proved that the [Al3+–HNS] complex released the free HNS by addition of F−. The overall changes in UV-vis spectra, 1H NMR spectra and HRMS analysis confirmed the dissociation between Al3+ and HNS, meanwhile, indirectly demonstrated the higher binding affinity of Al3+ toward F− compared to that with HNS.
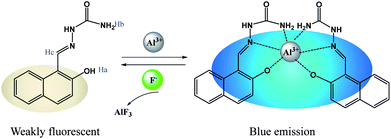 |
| Scheme 2 Probable sensing mechanism for Al3+ and F− by HNS. | |
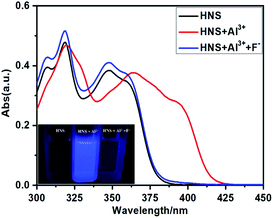 |
| Fig. 7 UV/vis spectra of HNS (40 μM) in the presence of Al3+ (4 equiv.) and Al3+ (4 equiv.) + F− (8 equiv.). Inset: the visual change exhibited for HNS in the presence of Al3+ and F− under UV light. | |
3.5 Cellular imaging by fluorescence microscopy
To demonstrate the potential of HNS to image Al3+ in living matrices, we carried out the experiments in SiHa cells. First, an MTT assay in SiHa cells has been performed to explore the cytotoxicity of HNS (Fig. S8†). The result confirmed that the HNS has no significant cytotoxicity on SiHa cells at a low concentration. As shown in Fig. 8, the cells showed no detectable fluorescence upon incubation only with HNS (50 μM) for 30 min. However, bright blue fluorescence was observed after the cells were incubated with receptor HNS (50 μM) and Al3+ (100 μM) simultaneously. Cells were subsequently incubated with a 500 μM solution of F− for 10 min under the same conditions, whereupon a significant decrease in the fluorescence was observed. During the whole process, the cells remained in good cell morphological state, demonstrating the low cytotoxicity of the probe further. These results demonstrate that HNS has good cell-membrane permeability and can be used for sequential detection of Al3+ and F− in an in vitro cellular system.
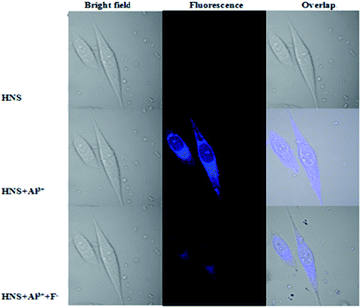 |
| Fig. 8 Fluorescence images of SiHa cells treated with HNS, Al3+ and F−. (Left) Bright field image; (middle) fluorescence image; (right) merged image. | |
4. Conclusions
In conclusion, we have developed an “off–on” type chemosensor HNS for Al3+ in weak acid aqueous solution. The receptor showed a high sensitivity for Al3+ detection at concentrations ranging from 0.8 μM to 8 μM with a detection limit of 67.5 nM. Moreover, fluorescence of the Al3+·HNS complex was drastically quenched by F− via Al3+ displacement. Thus the Al3+·HNS ensemble represents itself as an effective chemosensor for F− too. The detection limit of F− is quite low (0.789 μM) using this ensemble. Furthermore, HNS has been utilized successfully for sequential detection of Al3+ and F− ions in living cells. These merits of HNS, i.e., requiring facile one-step synthesis, working in aqueous media, possessing low detection limits, displaying high selectivity, and being suitable for monitoring Al3+ in living cell, make HNS a promising candidate as a chemosensor for Al3+ and F− ions.
Conflicts of interest
There are no conflicts to declare.
Acknowledgements
This work was financially supported by the National Natural Science Foundation of China (No. 21976113), the Natural Science Foundation of Shanxi Province (No. 201801D221142, 201801D221059), the Scientific and Technologial Innovation Programs of Higher Education Institutions in Shanxi (No. 2019L0787) and Beijing Natural Science Foundation (No. 7194337).
Notes and references
-
(a) E. Altschuler, Med. Hypotheses, 1999, 53, 22 CrossRef CAS PubMed;
(b) J. R. Walton, NeuroToxicology, 2006, 27, 385 CrossRef CAS PubMed;
(c) B. Wang, W. Xing, Y. Zhao and X. Deng, Environ. Toxicol. Pharmacol., 2010, 29, 308 CrossRef CAS PubMed.
- K. Soroka, R. S. Vithanage, D. A. Phillips, B. Walker and P. K. Dasgupta, Anal. Chem., 1987, 59, 629 CrossRef CAS.
- A. Gupta and N. Kumar, RSC Adv., 2016, 6, 106413 RSC.
- D. Kara, A. Fisher and S. J. Hill, J. Environ. Monit., 2007, 9, 994 RSC.
- Z. Liu, S. Li, G. Ge, Y. Li, C. Zhao, H. Zhang and Z. Yang, RSC Adv., 2019, 9, 5377 RSC.
- Y. Lu, S. S. Huang, Y. Y. Liu, S. He, L. C. Zhao and X. S. Zeng, Org. Lett., 2011, 13, 5274 CrossRef CAS PubMed.
- D. Maity and T. Govindaraju, Chem. Commun., 2012, 48, 1039 RSC.
- S. Sen, T. Mukherjee, B. Chattopadhyay, A. Moirangthem, A. Basu, J. Marek and P. Chattopadhyay, Analyst, 2012, 137, 3975 RSC.
- A. Sahana, A. Banerjee, S. Das, S. Lohar, D. Karak, B. Sarkar, S. K. Mukhopadhyay, A. K. Mukherjee and D. Das, Org. Biomol. Chem., 2011, 9, 5523 RSC.
- L. Cao, C. Jia, Q. Zhang, D. Chen, C. Zhang and Y. Qian, Chem. Res. Chin. Univ., 2014, 30(3), 362 CrossRef.
- Y. Wang, L. J. Hou, Y. B. Wu, L. L. Shi, Z. B. Shang and W. J. Jin, J. Photochem. Photobiol., A, 2014, 281, 40 CrossRef CAS.
- Y. P. Dai, X. Y. Liu, P. Wang, J. X. Fu, K. Yao and K. X. Xu, RSC Adv., 2016, 6, 99933 RSC.
- Q. Jiang, M. X. Li, J. Song, Y. Q. Yang, X. Xu, H. J. Xu and S. F. Wang, RSC Adv., 2019, 9, 10414 RSC.
- H. H. Wang, B. Wang, Z. H. Shi, X. L. Tang, W. Dou, Q. X. Han, Y. G. Zhang and W. S. Liu, Biosens. Bioelectron., 2015, 65, 91 CrossRef CAS PubMed.
- S. K. Sheet, B. Sen, R. Thounaojam, K. Aguan and S. Khatua, J. Photochem. Photobiol., A, 2017, 332, 101 CrossRef CAS.
- Y. J. Liu, F. F. Tian, X. Y. Fan, g F. L. Jian and Y. Liu, Sens. Actuators, B, 2017, 240, 916 CrossRef CAS.
- G. Kumar, K. Paul and V. Luxami, Sens. Actuators, B, 2018, 263, 585 CrossRef CAS.
- X. J. Sun, Y. Q. Ma, H. Fu, Z. Y. Xing, Z. G. Sun, Y. Shen and J. L. Li, J. Fluoresc., 2019, 29, 577 CrossRef CAS.
- J. C. Qin, T. R. Li, B. D. Wang, Z. Y. Yang and L. Fan, Spectrochim. Acta, Part A, 2014, 133, 38 CrossRef CAS PubMed.
- A. Amalraj and A. Pius, J. Fluorine Chem., 2015, 178, 73 CrossRef CAS.
- L. Tang, G. Y. Zhao, Z. L. Huang, N. N. Wang and J. J. Guo, Chem. Res. Chin. Univ., 2013, 29(2), 214 CrossRef CAS.
- M. Bineci, M. Bağlan and S. Atılgan, Sens. Actuators, B, 2016, 222, 315 CrossRef CAS.
- Y. Wang, Y. F. Song, L. Zhang, G. G. Dai, R. F. Kang, W. N. Wu, Z. H. Xu, Y. C. Fan and L. Y. Bian, Talanta, 2019, 203, 178 CrossRef CAS PubMed.
- X. Y. Sun, L. L. Wu, J. S. Shen, X. G. Cao, C. Wen, B. Liu and H. Q. Wang, RSC Adv., 2016, 6, 97346 RSC.
- D. Romi, D. P. Singh, B. S. Chauhan, S. Srikrishna, A. K. Panday, L. H. Choudhury and V. P. Singh, Sens. Actuators, B, 2018, 258, 881 CrossRef.
- X. Y. Kong, L. J. Hou, X. Q. Shao, S. M. Shuang, Y. Wang and C. Dong, Spectrochim. Acta, Part A, 2019, 208, 131 CrossRef CAS PubMed.
- J. X. Fu, B. Li, H. H. Mei, Y. X. Chang and K. X. Xu, Spectrochim. Acta, Part A, 2020, 227, 117678 CrossRef CAS PubMed.
- S. D. Hiremath, R. U. Gawas, S. C. Mascarenhas, A. Ganguly, M. Banerjee and A. Chatterjee, New J. Chem., 2019, 43, 5219 RSC.
- R. Purkait, C. Patra, A. D. Mahapatra, D. Chattopadhyay and C. Sinha, Sens. Actuators, B, 2018, 257, 545 CrossRef CAS.
- Y. W. Liu, C. H. Chen and A. T. Wu, Analyst, 2012, 137, 5201 RSC.
- S. M. Basheer, J. Haribabu, N. S. P. Bhuvanesh, R. Karvembu and A. Sreekanth, J. Mol. Struct., 2017, 1145, 347 CrossRef CAS.
- C. L. Li, P. H. Lu, S. F. Fu and A. T. Wu, Sensors, 2019, 19, 623 CrossRef PubMed.
- F. P. Schwarz and S. P. Wasik, Anal. Chem., 1976, 48, 524 CrossRef CAS PubMed.
- D. P. Roek, J. E. Chateauneuf and J. F. Brennecke, Ind. Eng. Chem. Res., 2000, 39, 3090 CrossRef CAS.
- R. B. Martin, Chem. Rev., 1996, 141, 23 Search PubMed.
- R. Azadbakht and S. Rashidi, Spectrochim. Acta, Part A, 2014, 127, 329–334 CrossRef CAS PubMed.
-
(a) J. S. Wu, W. M. Liu, X. Q. Zhuang, F. Wang, P. F. Wang, S. L. Tao, X. H. Zhang, S. K. Wu and S. T. Lee, Org. Lett., 2007, 9, 33 CrossRef CAS PubMed;
(b) D. Ray and P. K. Bharadwaj, Inorg. Chem., 2008, 47, 2252 CrossRef CAS PubMed;
(c) H. Y. Li, S. Gao and Z. Xi, Inorg. Chem. Commun., 2009, 12, 300 CrossRef CAS;
(d) W. M. Liu, L. W. Xu, R. L. Sheng, P. F. Wang, H. P. Li and S. K. Wu, Org. Lett., 2007, 9, 3829 CrossRef CAS PubMed;
(e) L. Li, Y. Q. Dang, H. W. Li, B. Wang and Y. Q. Wu, Tetrahedron Lett., 2010, 51, 618 CrossRef CAS.
- Y. K. Jang, U. C. Nam, H. L. Kwon, I. H. Hwang and C. Kim, Dyes Pigm., 2013, 99, 6 CrossRef CAS.
- X. Y. Shi, H. Wang, T. Y. Han, X. Feng, B. Tong, J. B. Shi, J. G. Zhi and Y. P. Dong, J. Mater. Chem., 2012, 22, 19296 RSC.
- R. J. Carton, Fluoride, 2006, 39(3), 163 CAS.
Footnote |
† Electronic supplementary information (ESI) available: 1H NMR spectrum, 13C NMR spectrum and HRMS spectrum of HNS. Job's plot for HNS and Al3+ complexation in EtOH/H2O. Benesi–Hildebrand plot from fluorescence titration data of HNS with Al3+. FTIR spectra of HNS and Al3+–HNS complex. 1H NMR spectrum, 13C NMR spectrum, and HRMS spectrum of Al3+–HNS complex after adding F−. See DOI: 10.1039/d0ra02848g |
|
This journal is © The Royal Society of Chemistry 2020 |