DOI:
10.1039/D0RA02757J
(Paper)
RSC Adv., 2020,
10, 26771-26776
Chitosan nanofiber-catalyzed highly selective Knoevenagel condensation in aqueous methanol†
Received
26th March 2020
, Accepted 7th July 2020
First published on 17th July 2020
Abstract
A chitosan nanofiber (CsNF)-catalyzed Knoevenagel reaction in green solvent, namely aqueous methanol, was investigated. CsNFs solely catalyzed the desired C–C bond formations in high yield with high selectivity, while conventional small-molecule amines, such as n-hexylamine and triethylamine, inevitably promoted transesterification to produce a large amount of solvolysis byproducts. Structural and chemical analyses of CsNFs suggested that the unique nanoarchitecture, in which chitosan molecules were bundled to ensure the high accessibility of substrates to catalytic sites, was critical to the highly efficient Knoevenagel condensation. The products were obtained in high purity without solvent-consuming purification, and the CsNF catalyst was easily removed and recycled. This study highlights a novel and promising function of CsNFs in green catalysis as emerging polysaccharide-based nanofibers.
Introduction
The sustainable development of society has increasingly required biomass utilization for the green production of energy and materials.1,2 In particular, unused waste from marine bioresources is regarded as a major global issue, remaining insufficient for use compared with forest biomass. Chitin is a polysaccharide from marine biomass, and the second most abundant biopolymer on Earth after cellulose, a typical polysaccharide in forest biomass.3 Chitin is found mainly in crab and prawn shells, with a structure comprising a β-1,4-linked N-acetyl-D-glucosamine repeat unit.3 The most important derivative of this polysaccharide is chitosan, which is obtained through partial deacetylation of chitin under alkaline conditions (Scheme 1a).4 Owing to their biocompatibility and multifaceted biological activity, chitosan and its derivatives have found a wide range of applications in medical, cosmetic, and agricultural fields.5–7 Towards the Sustainable Development Goals (SDGs) of our society, a new concept of “shell biorefinery” has been proposed to convert chitin/chitosan into a variety of valuable chemicals and materials under green conditions, and to promote the green applications.8–10
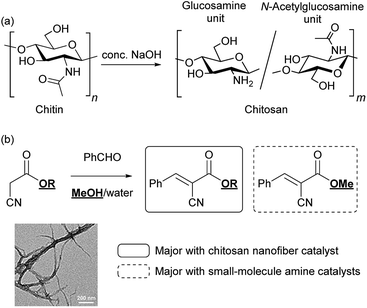 |
| Scheme 1 Schematic illustration of the research strategy: (a) structures of chitin (major: N-acetyl-D-glucosamine, minor: D-glucosamine) and chitosan (major: D-glucosamine, minor: N-acetyl-D-glucosamine); (b) selective Knoevenagel condensation using chitosan nanofibers as base catalyst. | |
Recently, natural polysaccharide nanofibers have been actively investigated to explore the possibility of using bioresources for advanced applications. Cellulose nanofibers (CNFs) have become the target of intensive research, especially in nanocomposite materials owing to their superb mechanical properties, such as high mechanical strength and low coefficients of thermal expansion.11,12 Furthermore, we recently found that the crystalline polysaccharide structures of CNFs in a nanofibrillated form play unprecedented roles in catalytic molecular transformations. The protonated form of surface-carboxylated CNFs (CNF-COOH) showed outstanding catalytic efficiency in acetal hydrolysis compared with the corresponding monomeric carboxylic acids and synthetic polymers bearing carboxy groups.13 Furthermore, adding CNFs to the reaction media promoted catalytic activity in proline-catalyzed aldol and Michael reactions.14,15
Chitosan nanofibers (CsNFs) have emerged in the last decade as a new type of natural polysaccharide nanofiber.16 The fascinating biological functions of chitosan have facilitated the intensive study of CsNFs for the development of tissue culture scaffolds and wound-dressing materials.17,18 However, current applications of marine polysaccharide nanofibers have yet to fully exploit their rich chemical functionality. Inspired by our recent works employing CNFs in catalytic transformations, as mentioned above, we envisaged that ubiquitous primary amino groups on the nanofiber would act as unique immobilized amine catalysts. Although some studies using chitosan as an amine catalyst and catalyst support in its non-nanofibrillated form, namely powder and aerogel forms, have been reported,19,20 CsNFs have rarely been employed in heterogeneous catalysis to date.21 The nanofibrillation of chitosan induces inherent surface chemical properties that make CsNFs very different to bulk catalysts and small-molecule amine catalysts. As the development of highly efficient heterogeneous catalysts is key to achieving green chemical production, CsNFs would be promising candidates as heterogeneous catalysts derived from non-fossil renewable materials.
In the present study, we report a CsNF-catalyzed Knoevenagel condensation in aqueous alcohol media. Interestingly, CsNFs alone successfully catalyzed a byproduct-free, highly selective Knoevenagel condensation, even in an alcoholic media, while conventional amine catalysts were accompanied by significant and unavoidable solvolysis to form a large amount of byproducts (Scheme 1b). Therefore, Knoevenagel condensation in green solvents, namely methanol and water, was achieved, despite having previously been conducted in hazardous polar aprotic or halogenated solvents.22 The present CsNF-catalyzed transformation meets three of the twelve key criteria proposed for green pharmaceutical manufacture, as follows: (1) catalyst immobilization without significant kinetic loss, (2) replacement of polar aprotic solvents, and (3) replacement of halogenated solvents.23
Experimental
Materials
Chitosan nanofiber (CsNF) was purchased from Sugino Machine Limited, Toyama, Japan (BiNFi-s, EFo-08002, 2.0 wt%, degree of polymerization = 480; see Fig. S1–S3 and Table S1† for characterization). Chitosan powder was purchased from FUJIFILM Wako Pure Chemical Industries, Ltd., Osaka, Japan (Chitosan 100, 1st grade, viscosity = 50–150 mPa s at 5 g L−1 and 20 °C, deacetylation degree = 80.0%; see Fig. S1–S3 and Table S1† for characterization). Polyallylamine hydrochloride (molecular weight = 50
000) and 3-aminopropyl-functionalized silica (specific surface area = 500 m2 g−1) were purchased from Sigma-Aldrich Japan, Tokyo, Japan. Polyallylamine hydrochloride was desalted by dialysis prior to use. All other reagents, chemicals, and organic solvents were purchased as reagent grade from Sigma-Aldrich Japan, FUJIFILM Wako Pure Chemical Industries, Ltd., and Tokyo Chemical Industry Co., Ltd. Benzaldehyde was purified by distillation prior to use. Other reagents and chemicals were used without further purification unless otherwise noted. Water used in this study was purified using a Barnstead Smart2Pure system (Thermo Scientific Co., Ltd., Tokyo, Japan).
Representative Knoevenagel condensation procedure
In a 100 mL screw-capped vial, benzaldehyde (1) (2.0 mmol, 203.3 μL) and ethyl cyanoacetate (2a) (1.0 mmol, 106.2 μL) were added to a 3
:
1 (v/v) mixture of methanol and deionized water (30 mL) containing CsNFs (23 mg dry weight, 10 mol%-NH2 compared with 2a). After vigorously stirring at 40 °C for 4 h, the reaction was quenched with 0.1 M aq. HCl solution and the resultant mixture was extracted three times with ethyl acetate. The combined organic layer was dried over MgSO4 and concentrated, and the residue was analyzed by 1H NMR to determine the yield using dibromomethane (30 μL, 0.43 mmol) as an internal standard. A gram-scale reaction was conducted by adding 1 (20 mmol, 2033 μL) and 2a (10 mmol, 1062 μL, 1.13 g) to a 3
:
1 (v/v) mixture of methanol and deionized water (200 mL) containing CsNFs (230 mg dry weight, 10 mol%-NH2) in a 500 mL eggplant flask. After vigorously stirring at 40 °C for 24 h, the reaction mixture was filtered to remove CsNFs, dried over MgSO4, and evaporated, affording Knoevenagel product 3a in 95% isolated yield (1.91 g) without purification. The purity of the obtained product was analyzed by a gas chromatography system equipped with a flame ionization detector (GC-FID; GC-2014AFsc, Shimadzu, Kyoto, Japan) using an InertCap 5MS/NP column (GL Sciences Inc., Tokyo, Japan).
Recycling of CsNFs in the Knoevenagel reaction
In a 250 mL centrifuge bottle, 1 (4.0 mmol, 406.6 μL) and 2a (2.0 mmol, 212.4 μL) were added to a 3
:
1 (v/v) mixture of methanol and deionized water (60 mL) containing CsNFs (46 mg dry weight, 10 mol%-NH2 compared with 2a). After vigorously stirring at 40 °C for 8 h, methanol (100 mL) was added, and the resulting mixture was centrifuged (20
000×g, 15 min). After collecting the supernatant in an Erlenmeyer flask, the precipitated CsNFs were resuspended in methanol (60 mL), and the suspension was centrifuged (20
000×g, 15 min). This process was repeated again. The combined supernatants were dried over MgSO4 and evaporated to afford product 3a. The precipitated CsNFs from the last centrifugation were resuspended in methanol to a total volume of 60 mL and subjected to the next Knoevenagel reaction. The reaction time was increased to 24 h for subsequent reactions.
Results and discussion
Chitosan nanofiber-catalyzed Knoevenagel condensation
This research was initiated by the reaction of benzaldehyde (1) and ethyl cyanoacetate (2a) in a mixed solvent of methanol and water (Table 1). The reaction proceeded smoothly in the presence of CsNFs (10 mol%-NH2 compared with 2a), with desired product 3a obtained quantitatively while keeping the ester moiety intact, as confirmed by 1H NMR analysis of the crude reaction mixture (entry 1, Fig. S4†). In contrast, using n-hexylamine, a small-molecule primary amine, resulted in a significant decrease in the amount of desired product 3a, along with a large amount of methyl ester 4 as a byproduct obtained by methanolysis (entry 2). Interestingly, n-hexylamine catalyzed both the Knoevenagel condensation and solvolysis of the ester moiety by methanol simultaneously, while the CsNF catalyst selectively facilitated the C–C bond forming Knoevenagel condensation over ester solvolysis. Motivated by this astonishing selectivity, we then investigated a series of catalysts, including small-molecule amines, different forms of chitosan, and macromolecular amines. The use of triethylamine (Et3N), a small-molecule tertiary amine, resulted in a low yield of target product 3a and a large amount of methyl ester 4, as in the case of n-hexylamine (entry 3). 2-Aminoethanol, bearing both primary amino and hydroxy groups in its molecular structure, similar to CsNF, also caused significant solvolysis, giving a low yield of target product 3a (entry 4). These results strongly suggested that conventional small-molecule amines inevitably catalyzed both Knoevenagel condensation and transesterification simultaneously. In contrast, non-nanofibrillated forms of chitosan, namely powder and hydrogel bead24,25 forms, showed relatively high selectivity for Knoevenagel condensation over solvolysis, although the catalytic activity was very low in both cases (entries 5 and 6). The low catalytic efficiencies of these chitosan catalysts were possibly attributed to the lower accessibility of amino groups, which act as the catalytic sites. Polyallylamine, a polyethylene-based synthetic polymer, also formed target product 3a in a low yield (entry 7). The poor catalytic activity of polyallylamine was presumably attributed to its poor solubility and severe aggregation in the reaction media. 3-Aminopropyl silica, a commercial porous silica particle with high specific surface area, demonstrated high catalytic activity, but yielded a significant amount of solvolysis product 4 along with target product 3a (entry 8). The comparison between polymeric and small-molecule catalysts suggested that the macromolecular structure of the amine catalysts played a crucial role in achieving such high selectivity for Knoevenagel condensation over solvolysis (entries 1, 5–7 vs. 2–4). Furthermore, the high specific surface area of the nanofibrillated form of chitosan, namely CsNF, granted outstanding catalytic activity compared with other bulk forms of chitosan and polyallylamine (entries 1 vs. 5–7). Therefore, the chemical and nanoarchitectural structure unique to CsNF was essential to realizing high catalytic activity and prominent selectivity in the present Knoevenagel condensation.
Table 1 Selectivity between C–C bond formation and solvolysis in base-catalyzed Knoevenagel condensationa
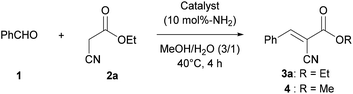
|
Entry |
Catalyst |
% Yieldb |
3a |
4 |
Unless otherwise noted, the reaction was carried out using 1 (2.0 mmol, 203.3 μL) and 2 (1.0 mmol, 106.2 μL) in MeOH (22.5 mL)/H2O (7.5 mL). Determined by 1H NMR analysis of the crude product using CH2Br2 as an internal standard. Not detected. |
1 |
CsNF |
>95 |
Trace |
2 |
n-Hexylamine |
40 |
48 |
3 |
Triethylamine |
21 |
45 |
4 |
2-Aminoethanol |
40 |
60 |
5 |
Chitosan powder |
51 |
n.d.c |
6 |
Chitosan hydrogel beads |
32 |
n.d.c |
7 |
Polyallylamine |
25 |
n.d.c |
8 |
3-Aminopropyl silica |
63 |
26 |
The difference in catalytic performance between CsNFs and small-molecule amines was further investigated in terms of transesterification activity. The time course of solvolysis for 2a/3a using a catalytic amount of CsNF or n-hexylamine was monitored by 1H NMR using CD3OD as solvent at room temperature (Fig. 1). As expected, both 2a and 3a were stable in the presence of CsNFs. In sharp contrast, 2a was almost completely solvolyzed within 1 h in the presence of n-hexylamine, while 3a was less reactive to solvolysis under the same conditions. These results indicated that the following reaction pathway was dominant for n-hexylamine as catalyst: ethyl ester 2a was first solvolyzed to the corresponding methyl ester, which then reacted with 1 to afford 4, although chitosan nanofibers affected neither ethyl esters 2a nor 3a. Such differences between CsNFs and small-molecule amines are possibly attributed to their basicities. Chitosan is a remarkably weak amine base (pKaH = 6.4),26 with a basicity four orders of magnitude smaller than those of common small-molecule amines, such as n-hexylamine and triethylamine. Such strong bases promoted solvolysis rather than the Knoevenagel condensation, possibly based on each activation energy. On the other hand, chitosan nanofibers could not act as a base for undesirable solvolysis, but surprisingly catalyzed the Knoevenagel condensation, owing to abundant and accessible amines exposed on the solid surface. This was attributed to amino groups at the C2 position of chitosan being electronically influenced by acetal at the C1 position, which are involved in intra- and intermolecular hydrogen bonds. Therefore, the moderate basicity of CsNFs only promoted Knoevenagel condensation, while keeping the ester moiety of 2a intact without solvolysis. In contrast, n-hexylamine as catalyst was active for both Knoevenagel condensation and ester solvolysis owing to its relatively high basicity.
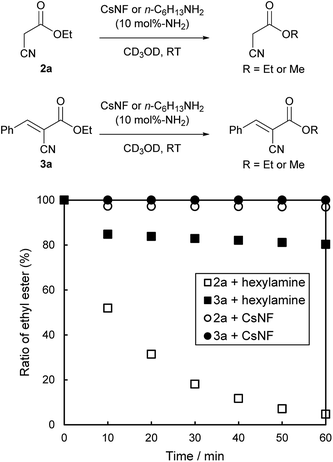 |
| Fig. 1 Time course of solvolysis ratio of 2a and 3a in CD3OD. | |
The superior catalytic activity of CsNFs over other forms of chitosan was rationalized by the amount of accessible catalytic sites. Transmission electron microscopy (TEM) observation showed the nanofibrillated structure of CsNFs (Fig. S1a†), suggesting an extremely high specific surface area and, therefore, a large portion of amino groups exposed at nanofiber interfaces. In contrast, a considerable number of amino groups are possibly embedded inside the powder form of chitosan polymer aggregates (Fig. S1c†). The amount of accessible amino groups in the catalytic reaction was quantified by separately reacting CsNFs and chitosan powder with salicylaldehyde, which quantitatively forms a stable imine with primary amines.24 Surprisingly, 92% of total amino groups formed imines in CsNFs, while imine formation was observed for 57% of total amino groups in chitosan powder. These results indicated that a large amount of catalytic sites was not available for the catalytic reaction in chitosan aggregates, resulting in low catalytic activity. The extremely high ratio of surface-exposed amino groups in CsNFs was further rationalized by XRD analysis (Fig. S2†), showing the higher crystallinity of CsNFs in which chitosan molecules were bundled in most parts of the fibers. FTIR results did not show any clear difference in the hydrogen bond networks between chitosan nanofibers and powders (Fig. S3†). Therefore, most of the amino groups were available for the catalytic reactions when the crystalline CsNFs (Fig. S2a†) were subjected to the reactions rather than powdery chitosan polymer aggregates with relatively low crystallinity (Fig. S2c†), although both chitosan samples possess the same chemical and intermolecular structures.
Substrate scope of CsNF-catalyzed Knoevenagel condensation
With optimal reaction conditions in hand, the scope and limitations of the present CsNF-catalyzed Knoevenagel condensation were investigated using various cyanoacetates bearing diverse functionalized ester side chains (Table 2). For all substrates examined, desired product 3 was obtained with very high selectivity using CsNFs compared with solvolysis to form product 4. In contrast, the reactions catalyzed by n-hexylamine afforded predominantly solvolysis product 4 and trace amounts of desired product 3. Therefore, direct transformation of functionalized cyanoacetates was achieved only when CsNFs were used as a base catalyst. Allyl and propargyl cyanoacetates (2b and 2c), which bear electron-rich double and triple bonds, respectively, afforded corresponding Knoevenagel products 3b and 3c with high selectivity only under the CsNF-catalyzed conditions. Benzyl ester 3d was also applicable to the present selective transformation, although a prolonged reaction time was required to obtain a moderate yield. Cyanoacetate bearing heteroatoms on its ester moiety was also tolerated in the reaction. Oxygen-bearing 2-ethoxyethyl ester 2e and nitrogen-bearing 2-aminoethyl ester 2f in its Boc-protected form afforded the corresponding Knoevenagel products 3e and 3f with high selectivity only under the CsNF-catalyzed conditions. Notably, an additional ester group at the terminus of the cyanoacetate ester moiety was also tolerated under the CsNF-catalyzed conditions, affording product 3g with high selectivity. In all cases, using n-hexylamine as a homogeneous base catalyst afforded large amounts of byproduct 4 from solvolysis. Furthermore, derivatization of the reaction product was demonstrated using Knoevenagel product 3b. Alkene metathesis of allyl ester 3b with methyl acrylate was performed using Hoveyda–Grubbs catalyst to afford desired cross metathesis product 5 in 69% yield (Scheme S1†).
Table 2 Substrate scope of CsNF-catalyzed Knoevenagel condensationa
Large-scale reaction with solvent-free workup process and CsNF recycling
The present CsNF-catalyzed Knoevenagel condensation has significant advantages, as follows: (i) the reaction affords the desired product with very high purity; (ii) heterogeneous catalyst CsNFs is easily removed by simple filtration or centrifugation; and (iii) environmentally benign and easily removable methanol is used as the solvent. To further demonstrate the usefulness of the present CsNF-catalyzed reaction in a preparative process, a gram-scale reaction was conducted, with the as-prepared product isolated using a simple centrifugation method (Scheme 2). Accordingly, 2a (1.13 g) reacted smoothly with 1 under the optimized conditions, although a prolonged reaction time was required to achieve complete conversion. The resulting reaction mixture was centrifuged to remove solid CsNFs suspended in the reaction media, and desired product 3a was obtained in 95% isolated yield. Notably, the purity of the as-obtained product was 97% by GC-FID analysis, even without any solvent-consuming extraction and column chromatography processes (Fig. S5 and S6†). In contrast to conventional Knoevenagel condensations, which often use halogenated or aprotic high-boiling-point solvents as reaction media and/or in purification processes,22 the present CsNF-catalyzed process can be conducted in environmentally benign alcohol media, and requires a simple workup without consuming additional organic solvents in all processes.
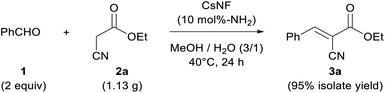 |
| Scheme 2 Gram-scale Knoevenagel condensation catalyzed by CsNF using methanol and water only throughout the process. | |
Finally, the recyclability of CsNFs as catalyst in the present Knoevenagel condensation was examined (Fig. 2). As CsNF catalyst was readily suspended and heterogeneous, it was easily recovered by centrifugation after the reaction. The recovered CsNFs were redispersed in another aqueous methanol media, and subjected to the next catalytic reaction. CsNFs could be reused four times without any significant loss of conversion, although the reaction time needed to be increased to 24 h. Furthermore, the selectivity for 3a over 4 remained high, with no trace of 4 detected in all runs. In the fifth run, the product yield dropped owing to CsNF loss during centrifugation after recycling five times. This seems to be because the amount of CsNFs recovered after the fifth run was 43% of the initial amount. TEM images revealed that nearly no morphological change of CsNFs occurred during the repeated uses (Fig. S1†). XRD data implied some change in the crystalline structure of the recycled CsNFs, whose (020) diffraction plane shifted from approx. 10° to 7° at a 2θ diffraction angle, possibly influencing the hydrated structure of CsNFs (Fig. S2†). This structural variation may cause the catalytic activity, to be further investigated in our future work. FTIR results show two characteristic peaks at 757 cm−1 and 694 cm−1, presumably originating from the C–H bending of monosubstituted aromatic compound (Fig. S3†). This implies the imine formation of benzaldehyde and CsNFs, probably indicating the reaction progress in the CsNFs-mediated Knoevenagel condensation.
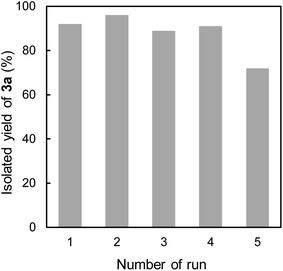 |
| Fig. 2 Recyclability of CsNFs in Knoevenagel condensation. | |
Conclusions
A chitosan nanofiber (CsNF)-catalyzed Knoevenagel condensation reaction has been successfully developed as a green process. In the reactions of benzaldehyde with various cyanoacetates using environmentally benign aqueous methanol media, CsNFs smoothly catalyzed the desired C–C bond formation. In contrast, small-molecule amine catalysts resulted in undesired transesterification through solvolysis with methanol. The CsNF-catalyzed reaction was applicable to gram-scale production, in which only a highly pure product was obtained without needing any solvent-consuming purification processes. Furthermore, CsNFs were easily recovered after the reaction and reused at least four times without significant loss of conversion and selectivity. The present research highlights an unprecedented catalytic function of marine polysaccharides in a nanofiber form, whose advanced applications are a central issue in utilizing unused waste biomass for the development of a green and sustainable society.
Conflicts of interest
There are no conflicts to declare.
Acknowledgements
This research was supported by an Advanced Low Carbon Technology Research and Development (ALCA) Program from the Japan Science and Technology Agency (grant no. JPMJAL1505 to T. K.), a Grant-in-Aid (KAKENHI) for Challenging Exploratory Research from the Japan Society for the Promotion of Science (grant no. JP18K19233 to T. K.), a Research Fellowship for Young Scientists from the Japan Society for the Promotion of Science (grant no. JP17J04176 to K. K.), and a Short-term Intensive Research Support Program from the Faculty of Agriculture, Kyushu University (M. H. and T. K.). We thank Simon Partridge, PhD, from Edanz Group (http://www.edanzediting.com/ac) for editing a draft of this manuscript.
Notes and references
- G. W. Huber, S. Iborra and A. Corma, Chem. Rev., 2006, 106, 4044–4098 CrossRef CAS PubMed.
- I. Delidovich, P. J. C. Hausoul, L. Deng, R. Pfützenreuter, M. Rose and R. Palkovits, Chem. Rev., 2016, 116, 1540–1599 CrossRef CAS PubMed.
- M. Rinaudo, Prog. Polym. Sci., 2006, 31, 603–632 CrossRef CAS.
- M. N. V. Ravi Kumar, React. Funct. Polym., 2000, 46, 1–27 CrossRef.
- Chitosan in the Preservation of Agricultural Commodities, ed., S. Bautista-Baños, G. Romanazzi and A. Jiménez-Aparicio, Academic Press, Massachusetts, 2016 Search PubMed.
- Chitosan Based Biomaterials Volume 1-Fundamentals, ed., J. Jennings and J. Bumgardner, Woodhead Publishing, Cambridge, 2016 Search PubMed.
- Chitosan Based Biomaterials Volume 2-Tissue Engineering and Therapeutics, ed. J. Jennings and J. Bumgardner, Woodhead Publishing, Cambridge, 2016 Search PubMed.
- N. Yan and X. Chen, Nature, 2015, 524, 155–157 CrossRef CAS PubMed.
- X. Chen, H. Yang and N. Yan, Chem.–Eur. J., 2016, 22, 13402–13421 CrossRef CAS PubMed.
- H. Y. Yang, G. Gözaydın, R. R. Nasaruddin, J. R. G. Har, X. Chen, X. N. Wang and N. Yan, ACS Sustainable Chem. Eng., 2019, 7, 5532–5542 CrossRef CAS.
- O. Nechyporchuk, M. N. Belgacem and J. Bras, Ind. Crops Prod., 2016, 93, 2–25 CrossRef CAS.
- B. Thomas, M. C. Raj, B. K. Athira, H. M. Rubiyah, J. Joy, A. Moores, G. L. Drisko and C. Sanchez, Chem. Rev., 2018, 118, 11575–11625 CrossRef CAS PubMed.
- Y. Tamura, K. Kanomata and T. Kitaoka, Sci. Rep., 2018, 8, 5021 CrossRef PubMed.
- K. Kanomata, N. Tatebayashi, X. Habaki and T. Kitaoka, Sci. Rep., 2018, 8, 4098 CrossRef PubMed.
- N. J. Ranaivoarimanana, K. Kanomata and T. Kitaoka, Molecules, 2019, 24, 1231 CrossRef PubMed.
- S. Ifuku, Molecules, 2014, 19, 18367–18380 CrossRef PubMed.
- R. Jayakumar, M. Prabaharan, S. V. Nair and H. Tamura, Biotechnol. Adv., 2010, 28, 142–150 CrossRef CAS PubMed.
- K. Azuma, S. Ifuku, T. Osaki, Y. Okamoto and S. Minami, J. Biomed. Nanotechnol., 2014, 10, 2891–2920 CrossRef CAS PubMed.
- E. K. Abdelkrim, ChemSusChem, 2015, 8, 217–244 CrossRef PubMed.
- O. Mahé, J. F. Brière and I. Dez, Eur. J. Org. Chem., 2015, 2559–2578 CrossRef.
- Y. Tsutsumi, H. Koga, Z. D. Qi, T. Saito and A. Isogai, Biomacromolecules, 2014, 15, 4314–4319 CrossRef CAS PubMed.
- L. Kurti and B. Czako, Strategic Applications of Named Reactions in Organic Synthesis, Academic Press, Massachusetts, 2005 Search PubMed.
- M. C. Bryan, P. J. Dunn, D. Entwistle, F. Gallou, S. G. Koenig, J. D. Hayler, M. R. Hickey, S. Hughes, M. E. Kopach, G. Moine, P. Richardson, F. Roschangar, A. Steven and F. J. Weiberth, Green Chem., 2018, 20, 5082–5103 RSC.
- K. R. Reddy, K. Rajgopal, C. U. Maheswari and M. Lakshmi Kantam, New J. Chem., 2006, 30, 1549–1552 RSC.
- D. Kühbeck, G. Saidulu, K. R. Reddy and D. D. Díaz, Green Chem., 2012, 14, 378–392 RSC.
- Q. Z. Wang, X. G. Chen, N. Liu, S. X. Wang, C. S. Liu, X. H. Meng and C. G. Liu, Carbohydr. Polym., 2006, 65, 194–201 CrossRef CAS.
Footnote |
† Electronic supplementary information (ESI) available. See DOI: 10.1039/d0ra02757j |
|
This journal is © The Royal Society of Chemistry 2020 |