DOI:
10.1039/D0RA02522D
(Paper)
RSC Adv., 2020,
10, 27697-27705
Synthesis of bismuth nanoparticle-loaded cobalt ferrite for electrochemical detection of heavy metal ions
Received
18th March 2020
, Accepted 15th July 2020
First published on 24th July 2020
Abstract
As an efficient modified electrode material for the detection of heavy metal ions, bismuth nanoparticles (BiNPs) were loaded on cobalt ferrite (CoFe2O4), a unique magnetic photocatalytic material, to fabricate a highly sensitive sensor. The obtained BiNPs@CoFe2O4 nanocomposites showed excellent adsorption and electrical conductivity using a Square Wave Anodic Stripping Voltammetry (SWASV) detection method. Under optimized conditions, the BiNPs@CoFe2O4/GCE sensor could simultaneously determine Pb2+ and Cd2+, with detection limits of 7.3 and 8.2 nM, respectively. In addition, the BiNPs@CoFe2O4 exhibited acceptable reproducibility and good stability, which indicated great potential for the detection of heavy metal ions in reality.
1. Introduction
Heavy metal ions (HMI) are one of the micro-pollutants that severely affect the environment. Lead (Pb), cadmium (Cd), mercury (Hg), chromium (Cr) and arsenic (As) are highly toxic among the HMIs. These harmful HMIs can cause various diseases of the human body even at low concentration. While not being biodegradable, HMIs can exist in organisms and plants for decades or even hundreds of years after being released into the environment.1–3 There are several analytical methods for detecting HMIs such as inductively coupled plasma mass spectrometry (ICP-MS), atomic absorption spectroscopy (AAS), X-ray fluorescence spectroscopy (XRF) and inductively coupled plasma optical emission spectroscopy (ICP-OES).4 However, these technical devices are very expensive and require trained personnel to operate, which limits the application of these methods. Therefore, it is of crucial importance to develop fast, accurate and reliable techniques to detect HMIs for environmental and health protection. Compared to other spectroscopic and optical techniques, electrochemical detection techniques for HMIs have advantages of high sensitivity, low cost and providing on-site and real time monitoring.5 The modification of electrode can improve electrochemical detection sensitivity and detection limit. Due to the toxicity of mercury, bismuth-based electrodes with low toxicity have been an effective alternative to mercury-based electrodes.6 The bismuth-based electrodes are environmental friendly and show excellent resolution of neighboring peaks. CoFe2O4 is a composite oxide containing trivalent iron oxide as the main component, and has attracted much attention in recent years. Compared to other magnetic materials, cobalt ferrite exhibits unique physical, chemical, magnetic and catalytic properties, thus suitable to serve as the substrate to immobilize BiNPs.7
In this study, BiNPs@CoFe2O4 nanocomposites were synthesized by in situ reduction method. The nanocomposites were characterized by SEM, TEM, XRD, XPS, and the elemental composition was analyzed by EDS spectrum analysis. After characterizing the synthesized BiNPs@CoFe2O4 nanocomposites, we modified the glassy carbon electrode (GCE) as an electrochemical sensing platform for the determination of HMIs. Afterwards, the application of the electrochemical sensor in the determination of Pb2+ and Cd2+ ions in water samples was demonstrated by SWASV technique, and its sensitivity, selectivity, reproducibility and stability were studied. The experimental results showed that the BiNPs@CoFe2O4 nanocomposite modified sensor has high electrochemical performance and adsorption performance for Pb2+ and Cd2+ ions.
2. Experimental section
2.1 Materials
Fe(NO3)3·9H2O, cobalt acetate tetrahydrate, NH4F, Bi(NO3)3, Pb(NO3)2, CdCl2, MgCl2, FeCl3, CoCl2, ZnCl2, MnCl2, CuSO4, Al(NO3)3, Ni(NO3)2, H2SO4, HNO3, C6H5Na3O7, NaBH4, CH3COOH, urea, chitosan (CS), absolute ethanol and ethylene glycol were purchased from Fengchuan Chemical Reagent Co. Ltd. (Tianjin, China), which were all of analytical grade. 0.1 M acetate buffer solution with different pH was used as the supporting electrolyte in this work, which was prepared by mixing stock solutions of 0.1 M sodium acetate and acetic acid.
2.2 Preparation of CoFe2O4
8 mM ferric nitrate nonahydrate [Fe(NO3)3·9H2O], 4 mM cobalt acetate tetrahydrate, 80 mM ammonium fluoride (NH4F) and 120 mM urea were dissolved in 30 mL ultra-pure water, stirred at room temperature for 10 min, and then dripped 0.1 M HNO3 to the solution pH of 5.0. It was then transferred to a 50 mL polytetra liner. Sealed in autoclave and reacted at 180 °C for 20 h. The sediment was collected after cooling naturally to room temperature, and finally washed three times with deionized water and ethanol. The final product (cobalt ferrite porous microspheres, CoFe2O4) was obtained after drying at 60 °C for 6 h.8
2.3 Preparation of BiNPs@CoFe2O4 nanocomposites
Certain amount of CoFe2O4 was dispersed in 5 mL of ethylene glycol and ultrasonicated for 1 h. After dissolving 5 mg of C6H5Na3O7 (worked as capping and reduction agent) in the dispersion, 5 mL of Bi(NO3)3·5H2O in ethylene glycol was added and the mixture was stirred for 12 h. Then 0.5 mL of 0.1 M NaBH4 was added to the mixture for reducing Bi3+.9–11 After shaking for 1 h, the synthesized solid products were separated by centrifugation, and then washed thoroughly with distilled water and absolute ethanol to remove any impurities. The obtained solid products were dried in a vacuum oven at 50 °C for 24 h. Finally, the BiNPs@CoFe2O4 nanocomposites were obtained.
2.4 Fabrication of BiNPs@CoFe2O4/GCE sensor
Before fabrication of sensor, the GCE was mechanically polished to a mirror-like surface with alumina micro powder (0.3 and 0.05 μm alumina slurries), successively sonicated with absolute ethanol, HNO3 (0.05 M) and distilled water, respectively. After that, the GCE was activated in 0.5 M H2SO4.
1 mg BiNPs@CoFe2O4 nanocomposites were dispersed in 1 mL 0.20% CS solution and then sonicated to obtain homogeneous suspension. Then, 5 μL of the suspension was directly drop-cast on the pretreated GCE and dried in air. Finally, the BiNPs@CoFe2O4/GCE sensor was obtained.
2.5 Characterization
The morphology of CoFe2O4 porous microspheres and BiNPs@CoFe2O4 nanocomposites was characterized by SEM (NanoSEM45011, FEI, America) and TEM (JEM-2100, JEOL Inc., Japan). The elemental compositions were taken on EDX (EDAX Inc., Mahwah, USA). The crystallinity was determined by XRD diffractometer (D8 Advance, Bruker, karlsruhe, Germany). The chemical state of the samples was demonstrated using XPS (Da Vinci, Bruker, Germany).
2.6 Electrochemical measurements
Electrochemical measurements were performed on CHI660 electrochemical workstation (Shanghai Chenhua, China) and a conventional three-electrode system (counter electrode – platinum wire, working electrode – BiNPs@CoFe2O4/GCE, reference electrode – saturated calomel electrode (SCE)). The tested electrochemical techniques were cyclic voltammograms (CV), electrochemical impedance spectra (EIS) and chronocoulometry (CC). The electrochemical properties of the produced sensor were determined according to previous study. The active area of BiNPs@CoFe2O4 nanocomposites was calculated using the following equation:
where Q, the absolute value of the reduction charge; n, the number of electrons transfer; F, the Faraday constant (96
485 C mol−1); A, the effective area; c, the substrate concentration; D, the diffusion coefficient (7.6 × 10−6 cm2 s−1); t, the time; Qdl, the double-layer charge; Qads, the faradaic charge consumed by adsorbed species.
2.7 Electrochemical detection of Pb2+ and Cd2+
SWASV was applied at a deposition potential of −1.1 V for 300 s in 0.1 M acetate buffer solution (pH 5.0), and performed in the potential range of −0.95 to −0.35 V with a frequency of 15 Hz, an amplitude of 25 mV, and an increment potential of 4 mV. The stability of BiNPs@CoFe2O4/GCE was investigated by repetitively determining 0.2 μM Pb2+ and 0.4 μM Cd2+ ten times and the response sensitivity was evaluated over six weeks. The reproducibility of the sensor was investigated by studying six sensors in parallel by detecting 0.2 μM Pb2+ and 0.4 μM Cd2+.
3. Results and discussion
3.1 Characterization of the BiNPs@CoFe2O4
The porous spherical morphology and microstructure of CoFe2O4 were characterized by SEM. As shown in Fig. 1A, the diameter of CoFe2O4 was about 2 μm and the microspheres are uniform and homogenously dispersed. The high-magnification SEM image (Fig. 1B) indicated that its morphology resembles a dandelion pollen grain and exhibits a highly layered porous structure. The large pores on the outer wall of the microspheres are about 200 nm. CoFe2O4 was obviously porous and possessed a relatively large space inside the microspheres.12 According to the previous report, a morphological evolution from nanoparticle aggregates to hierarchical porous CoFe2O4 structures. In the first stage of the synthesis reaction, at low pH values, Fe3+ can easily react with the OH− produced by urea and NH4F to form FeOOH and thus the nanoparticle aggregates were generated. Subsequent growth was took place around the grain boundary of the nanoparticle aggregates. With the reaction time increased, the FeOOH nanoparticle gradually dissolved and the dissolved Fe3+ combined with Co2+ can recrystallize into nanoparticles to form hierarchical CoFe2O4 porous microspheres. In this process, FeOOH nanoparticle worked as the template for porous structure. To further investigate the microstructure of BiNPs@CoFe2O4, TEM examination was conducted and the resulted picture indicated that the inner part of the microspheres is loose with large interspace (Fig. 1C), and the BiNPs (dark dots) were distributed on the CoFe2O4 microspheres hole surface and interior. The fringes of the marked lattice spacing of 0.32 nm in Fig. 1D correspond to the (012) facet of Bi.13 EDX mapping analysis (Fig. 1E and F) revealed that the Bi element was uniformly distributed on the microspheres, which confirmed the uniform and successful attachment of BiNPs on CoFe2O4.
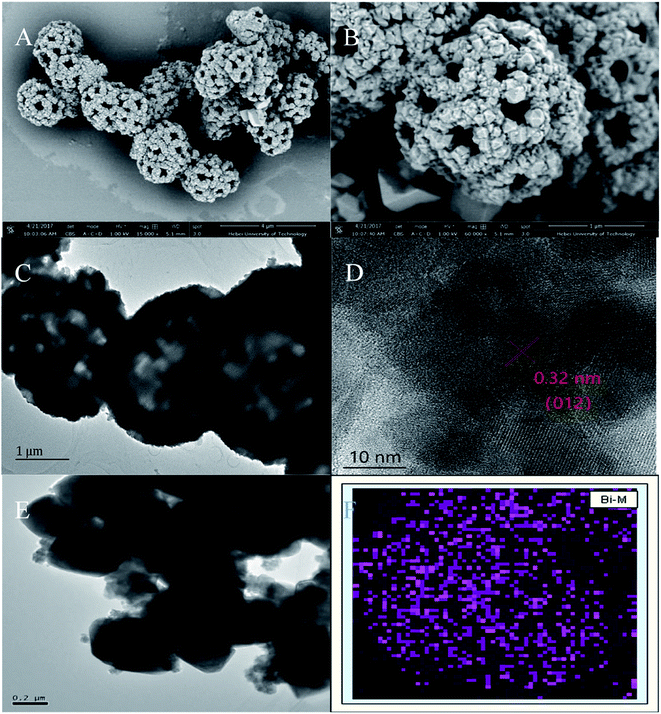 |
| Fig. 1 (A and B) SEM images of CoFe2O4, (C and D) TEM images of CoFe2O4 and BiNPs@CoFe2O4, (E and F) EDX mapping of Bi. | |
As shown in Fig. 2A, all diffraction peaks of the XRD patterns can be assigned to a single phase of CoFe2O4 and Bi with a face-centered-cubic spinel structure (JCPDS no. 22-1086 and no. 5-519). For XPS spectrum (Fig. 2B), it is show that both CoFe2O4 microspheres and BiNPs@CoFe2O4 were composed Fe, Co, C, and O. Moreover, the XPS spectra of BiNPs@CoFe2O4 showed presence of Bi, suggesting that BiNPs@CoFe2O4 had been synthesized successfully. As shown in Fig. 2C, the peaks of Bi 4f (4f7/2 and 4f5/2) were qualified to Bi(0), which indicated that nearly all bismuth in the BiNPs@CoFe2O4 was Bi(0).14 In brief, all these results confirmed the successful preparation of BiNPs@CoFe2O4.
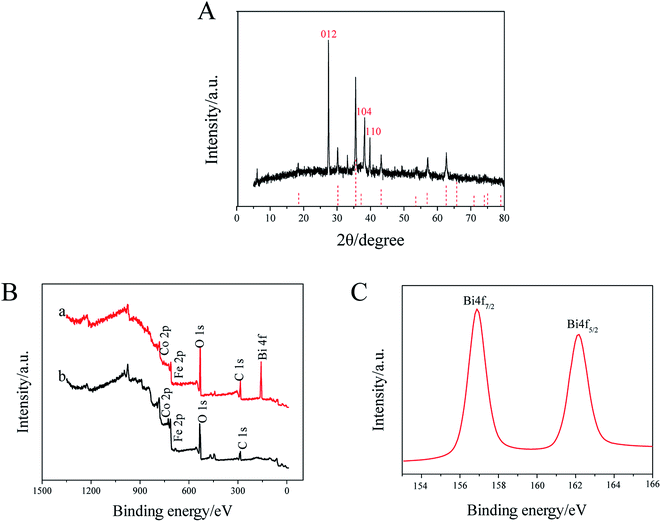 |
| Fig. 2 (A) XRD pattern of BiNPs@CoFe2O4. (B) XPS spectra of CoFe2O4 (b) and BiNPs@CoFe2O4 (a), (C) Bi 4f XPS spectrum of Bi. | |
3.2 Electrochemical characterization of the BiNPs@CoFe2O4/GCE
The cyclic voltammograms in 5 mM K3[Fe(CN)6] and 0.1 M KCl mixture solution were used to investigate the electron transfer properties of the electrodes. Comparing the cyclic voltammograms of bare GCE, CoFe2O4/GCE and BiNPs@CoFe2O4/GCE, it can be seen that BiNPs@CoFe2O4/GCE shows the largest peak current and the increase of peak current (Fig. 3A), indicating that the material of the modified electrode has good conductivity.15 It is beneficial for Fe(CN)63−/4− to be close to the electrode surface. In addition, EIS was used to study the interfacial electron transfer resistance (Ret). The data in Fig. 3B showed that three Rets of 1500 U on bare GCE, 500 U of CoFe2O4/GCE and 100 U of BiNPs@CoFe2O4/GCE were obtained respectively. The reduced Ret was attributed to the distended high electrical conductivity and specific area, which can improve the transfer of electron and mass exchange of electro-active indicators on surface.16 As shown in Fig. 3C and D, the active area of BiNPs@CoFe2O4/GCE is the largest (0.05315 cm2) after calculation, indicating that it has a large electrode active area and can be used as a platform for sensitive sensing metal ions.17 The above advantages can also be further demonstrated by the SWASV response to heavy metal ions. As shown in Fig. 4, we can observe sharp elution peaks and large peak currents, indicating that BiNPs@CoFe2O4/GCE has a good adsorption performance and electrical conductivity.
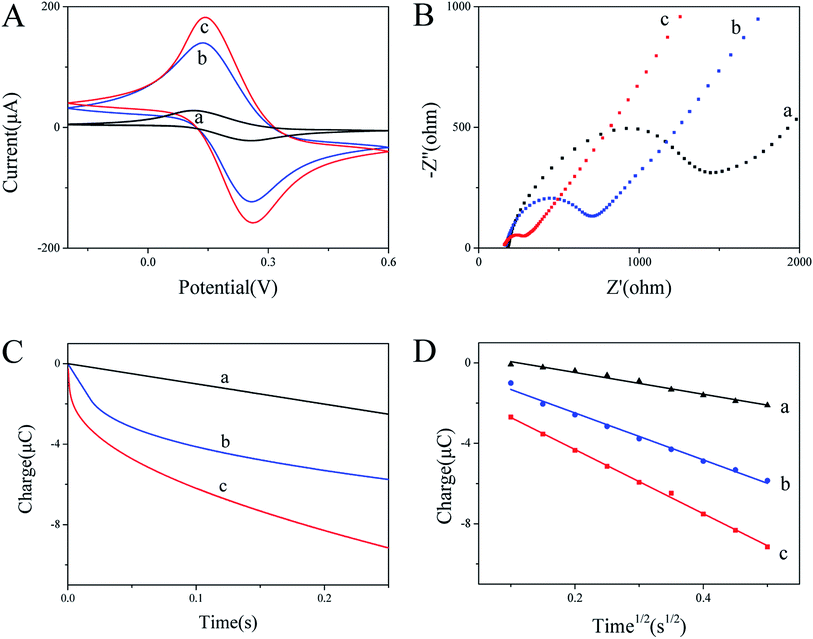 |
| Fig. 3 (A) Cyclic voltammograms of GCE bare (a), CoFe2O4/GCE (b) and BiNPs@CoFe2O4/GCE (c); (B) EIS spectra of GCE bare (a), CoFe2O4/GCE (b) and BiNPs@CoFe2O4/GCE (c); (C and D) plots of Q–t and Q–t1/2 of the tested electrodes. | |
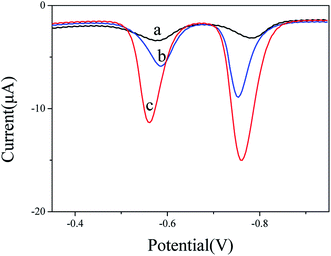 |
| Fig. 4 SWASV curves of 0.2 μM Pb2+ and 0.4 μM Cd2+ at bare GCE (a), CoFe2O4/GCE (b) and BiNPs@CoFe2O4/GCE (c) in 0.1 M acetate buffer solution (pH 5.0). | |
3.3 Optimization of working conditions for BiNPs@CoFe2O4/GCE
To optimize the sensitivity and accuracy of the modified electrode, the following parameters affecting electrode performance were optimized. Firstly, as shown in Fig. 5A, the Pb2+ and Cd2+ showed the optimum response current at pH 5.0. As shown in Fig. 5B, the deposition potential was also optimized. Obviously, the stripping voltammetric peak current of Pb2+ and Cd2+ reaches a maximum at −1.1 V. While further decreasing potentials, competitive hydrogen evolution was increased and led to the stripping voltammetric peak current of Pb2+ and Cd2+ are continuously decrease. Thus, −1.1 V was chosen as the optimal enrichment potential. Furthermore, deposition time was also optimized, as shown in Fig. 5C. It can be seen from the figure that the response signals increased rapidly due to more metal ions were reduced on the electrode. Next, the deposition time was increased to 500 s, slow increments in signals occurred due to the saturation of active sites on BiNPs. In practical applications, a higher response signal produced with shorter deposition time was favorable. Thus, in the following experiments, the deposition time of 300 s was chosen. The influence of mass fraction on response signals is shown in Fig. 5D. With increasing weight ratio of CoFe2O4, the SWASV responses of both Pb2+ and Cd2+ increased and reached maxima at 1
:
8. After reaching certain content, the SWASV peak currents of Pb2+ and Cd2+ begin to decrease, which could be attributed to the relatively low ratio of BiNPs.18
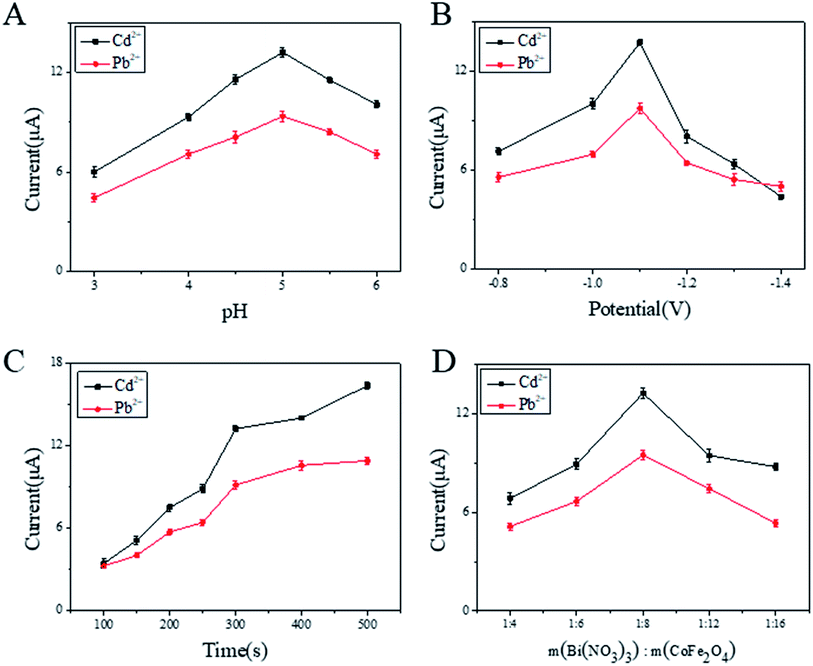 |
| Fig. 5 Optimization of experimental conditions (A–D) on SWASV response of 0.2 μM Pb2+ and 0.4 μM Cd2+ in acetate buffer at BiNPs@CoFe2O4/GCE. | |
3.4 Analytical performance
As shown in Fig. 6A, the dissolution peak voltammetric potential of Pb2+ and Cd2+ on BiNPs@CoFe2O4/GCE are around −0.6 V and −0.8 V. With the increasing concentrations of Pb2+ and Cd2+, the stripping peak currents exhibited a rapid and sensitive current response. The linear functional relationship between the amperometric response and the concentration was divided into two ranges, 0.06 to 0.6 μM and 0.08 to 0.8 μM, respectively. The linear equations were evaluated as I/μA = −0.89174 + 49.97671C/μM and I/μA = −2.55857 + 42.70202C/μM for Pb2+ and Cd2+, with correlation coefficients of 0.99681 and 0.99333, respectively. The limits of detection were calculated to be 7.3 nM (1.51 ppb) for Pb2+ and 8.2 nM (0.92 ppb) for Cd2+, which are much lower than the respective concentrations 10 μg L−1 and 3 μg L−1 specified by the World Health Organization.19
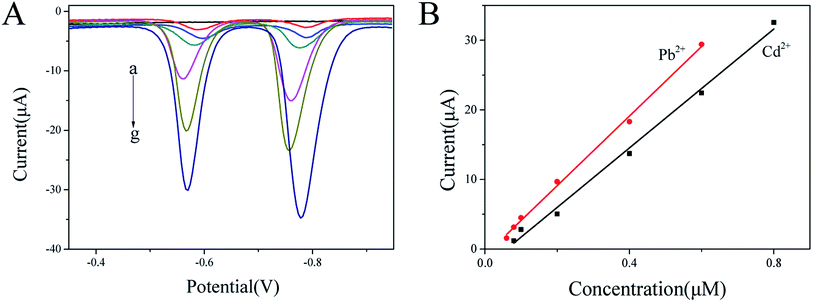 |
| Fig. 6 (A) SWASV responses of BiNPs@CoFe2O4/GCE for the simultaneous analysis of Pb2+ and Cd2+ at 0, 0.06, 0.08, 0.1, 0.2, 0.4 and 0.6 μM for Pb2+, and 0, 0.08, 0.1, 0.2, 0.4, 0.6 and 0.8 μM Cd2+, respectively. (B) Calibration curves for Pb2+ and Cd2+. | |
3.5 Interference study
Mutual interference between heavy metal ions becomes a major problem in simultaneously detecting metal ions.20 Therefore, the interference of Pb2+ and Cd2+ was evaluated. By keeping one ion concentration constant and increasing another ion concentration, the dissolution peak currents of the two are substantially unchanged. Furthermore, a good linear relationship between Pb2+ and Cd2+ are obtained in the ranges of 0.06–0.6 μM and 0.08–0.6 μM, respectively. The linear equations were evaluated as I/μA = −1.40265 + 50.99795C/μM and I/μA = −1.57632 + 38.80912C/μM, for Pb2+ and Cd2+, with correlation coefficients of 0.99499 and 0.99869, respectively. The detection slopes of both Pb2+ and Cd2+ were similar to those shown in Fig. 7 (50.99795 and 49.97671 for Pb2+ and 38.80912 and 42.70202 for Cd2+), which indicated that no mutual interference between Pb2+ and Cd2+ at BiNPs@CoFe2O4/GCE.
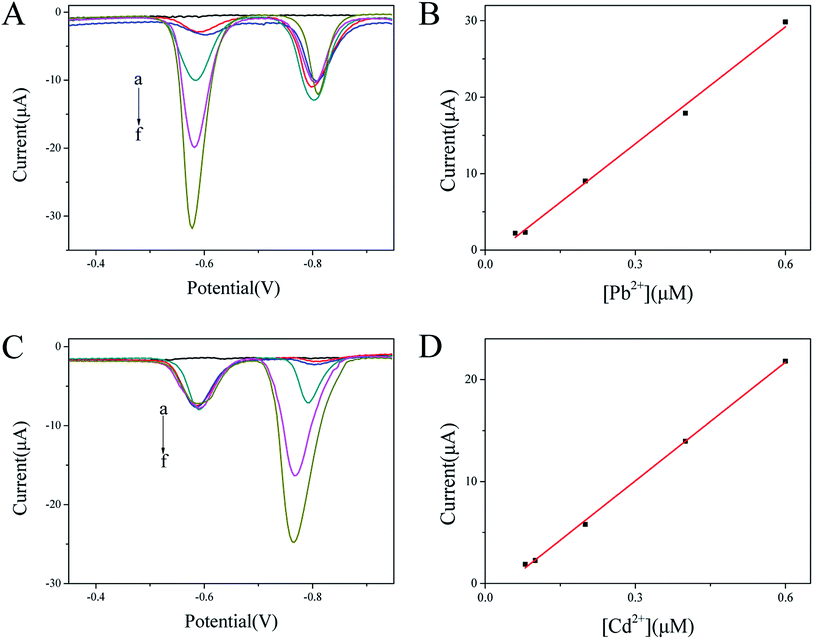 |
| Fig. 7 (A) SWASV curves of Pb2+ at 0, 0.06, 0.08, 0.2, 0.4 and 0.6 μM in presence of 0.4 μM Cd2+ at BiNPs@CoFe2O4/GCE. (B) Corresponding linear calibration plots against Pb2+. (C) SWASV curves of Cd2+ at 0, 0.08, 0.1, 0.2, 0.4 and 0.6 μM in presence of 0.2 μM Pb2+ at BiNPs@CoFe2O4/GCE. (D) Corresponding linear calibration plots against Cd2+. | |
In order to explore the anti-interference performance of this sensor, interfering ions such as Mg2+, Al3+, Cu2+, Zn2+, Mn2+, Co2+, Ni2+ and Fe3+ were added to 0.2 μM Pb2+ and 0.4 μM Cd2+ at a concentration of 10 μM. The results in Fig. 8 show little changes in response signals except Cu2+. In the state of adding Cu2+, the signals were significantly reduced, which may be due to the intermetallic compounds formation that can be avoided by adding ferrocyanide for forming Cu2+ stable complexes.21 In addition, the anions such as Cl−, SO42− and NO3− did not have obvious effect on the stripping peak current. The results demonstrated that the prepared the sensor had anti-interference ability.
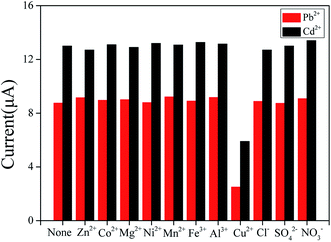 |
| Fig. 8 Selectivity of BiNPs@CoFe2O4/GCE for simultaneous detection of Pb2+ and Cd2+. | |
3.6 Reproducibility and stability of the sensors
Reproducibility and long-term stability are important parameters to evaluate the performance of the sensors. The repeatability of the BiNPs@CoFe2O4/GCE was performed by stripping voltammetry of 0.2 μM Pb2+ and 0.4 μM Cd2+ ten times. The relative standard deviation (RSD) was found to be 3.2% and 2.5%. These results indicated that the BiNPs@CoFe2O4/GCE sensors possessed excellent stability. When it was stored six weeks, the sensor remained about 94% and 93% of its original response for Pb2+ and Cd2+, respectively. The reproducibility of six electrodes which were prepared in parallel with the same modification method was also studied. The corresponding relative standard deviations of detecting 0.2 μM Pb2+ and 0.4 μM Cd2+ were 3.7% and 2.7%, respectively. The high stability and good reproducibility was recognized to the structure stability nature and binding strength of BiNPs@CoFe2O4 nanocomposites on GCE.22
3.7 Practical assay in tap water
Recovery experiments were used to demonstrate the applicability of the sensors in tap water samples. The concentrations of Pb2+ and Cd2+ were calculated through the standard addition method, with additions of 150 nM Pb2+ and 150 nM Cd2+. As shown in Table 1, the recovery rate of Pb2+ and Cd2+ are 98.9% ∼ 103.6%. The above results show that the sensors can be used for the detection of Pb2+ and Cd2+ in real samples.
Table 1 Determination of Pb2+ and Cd2+ in water samples with the BiNPs@CoFe2O4/GCE
Samples |
Added (nM) |
Result (nM) |
Recovery (%) |
Tap water |
Pb2+ 150 |
148.3 ± 1.05 |
98.9 |
Cd2+ 150 |
155.4 ± 1.31 |
103.6 |
As can be seen in Table 2, the detection limits of BiNPs@CoFe2O4/GCE were compared with the reported electrodes modified with other materials. The detection limit of Cd2+ is obviously lower than that of most other modified electrodes, but the detection limit of Pb2+ is not ideal, which may be due to that BiNPs@CoFe2O4 nanocomposites integrated the advantages of BiNPs and CoFe2O4 including high surface area and good conductivity. Therefore, the BiNPs@CoFe2O4 nanocomposites can be applied as a good electrode-modification material for fast and convenient determining of heavy metal ions in environmental systems.
Table 2 List of various electrochemical sensors for Pb2+ and Cd2+ detection
Electrochemical platform |
Technique |
Analyte (HMI) |
LOD |
Bi–C nanocomposite23 |
SWASV |
Pb2+ |
0.65 ppb |
Cd2+ |
0.81 ppb |
RGO/Bi nanocomposite24 |
ASV |
Pb2+ |
0.55 ppb |
Cd2+ |
2.8 ppb |
BiNPs25 |
SWASV |
Pb2+ |
2 ppb |
Cd2+ |
5 ppb |
Nano-Bi26 |
SWASV |
Pb2+ |
1.97 ppb |
Cd2+ |
2.54 ppb |
NPCGS/Bi nanocomposite18 |
SWASV |
Pb2+ |
0.66 ppb |
Cd2+ |
0.46 ppb |
CoFe2O4/Bi nanocomposite |
SWASV |
Pb2+ |
1.51 ppb |
Cd2+ |
0.92 ppb |
4. Conclusions
Through combining the advantages of BiNPs and CoFe2O4, BiNPs@CoFe2O4 nanocomposites with high surface area and good conductivity were prepared. BiNPs@CoFe2O4 was used to modify electrochemical electrode for heavy metal ions detection as sensing technology. The obtained sensor can simultaneously detect Pb2+ and Cd2+ with high sensitivity and good exactness. Thus, the BiNPs@CoFe2O4 nanocomposites can be applied as an excellent electrode-modification material for fast and convenient determining of heavy metal ions in environmental systems.
Conflicts of interest
There are no conflicts to declare.
References
- P. B. Tchounwou, C. G. Yedjou, A. K. Patlolla and D. J. Sutton, Heavy Metal Toxicity and the Environment, Molecular, Clinical and Environmental Toxicology, 2012, 101, 133–164 CrossRef PubMed
. - A. Afkhami, F. S. Felehgari, T. Madrakian, H. Ghaedi and M. Rezaeivala, Fabrication and application of a new modified electrochemical sensor using nano-silica and a newly synthesized Schiff base for simultaneous determination of Cd2+, Cu2+ and Hg2+ ions in water and some foodstuff samples, Anal. Chim. Acta, 2013, 771, 21–30 CrossRef CAS PubMed
. - T. Gong, J. Liu, X. Liu, J. Liu, J. Xiang and Y. Wu, A sensitive and selective platform based on CdTe QDs in the presence of L-cysteine for detection of silver, mercury and copper ions in water and various drinks, Food Chem., 2016, 213, 306–312 CrossRef CAS PubMed
. - L. Pujol, D. Evrard, K. G. Serrano, M. Freyssinier, A. R. Cizsak and P. Gros, Electrochemical sensors and devices for heavy metals assay in water: the French groups' contribution, Front. Chem., 2014, 2, 19 Search PubMed
. - G. Aragay and A. Merkoci, Nanomaterials application in electrochemical detection of heavy metals, Electrochim. Acta, 2012, 84, 49–61 CrossRef CAS
. - N. Serrano, A. Alberich, J. M. D. Cruz, C. Arino and M. Esteban, Coating methods, modifiers and applications of bismuth screen-printed electrodes, TrAC, Trends Anal. Chem., 2013, 46, 15–29 CrossRef CAS
. - L. Zhao, H. Zhang, Y. Xing, S. Song, S. Yu, W. Shi, X. Guo, J. Yang, Y. Lei and F. Cao, Studies on the magnetism of cobalt ferrite nanocrystals synthesized by hydrothermal method, J. Solid State Chem., 2008, 181, 245–252 CrossRef CAS
. - A. S. Teja and P. Y. Koh, Synthesis, properties and applications of magnetic iron oxide nanoparticles, Prog. Cryst. Growth Charact. Mater., 2009, 55, 22–45 CrossRef CAS
. - C. Stubenrauch, T. Wielputz, T. Sottmann, C. Roychowdhury and F. J. Disalvo, Microemulsions as templates for the synthesis of metallic nanoparticles, Colloids Surf., A, 2008, 317, 328–338 CrossRef CAS
. - X. Hu, D. Pan, H. Han, M. Lin, Y. Zhu and C. Wang, Preparation of bismuth-based microrods and their application in electroanalysis, Mater. Lett., 2017, 190, 83–85 CrossRef CAS
. - K. Chen, W. Fan, C. Huang and X. Qiu, Enhanced stability and catalytic activity of bismuth nanoparticles by modified with porous silica, J. Phys. Chem. Solids, 2017, 110, 9–14 CrossRef CAS
. - I. Safarik and M. Safarikova, Magnetic nano- and microparticles in biotechnology, Chem. Pap., 2009, 63, 497–505 CAS
. - P. Xiong, P. Bai, A. Li, B. Li, M. Cheng, Y. Chen, S. Huang, Q. Jiang, X. H. Bu and Y. Xu, Bismuth Nanoparticle@Carbon Composite Anodes for Ultralong Cycle Life and High-Rate Sodium-Ion Batteries, Adv. Mater., 2019, 31, 1904771 CrossRef CAS PubMed
. - L. Shi, Y. Li, X. Rong, Y. Wang and S. Ding, Facile fabrication of a novel 3D graphene framework/Bi nanoparticle film for ultrasensitive electrochemical assays of heavy metal ions, Anal. Chim. Acta, 2017, 968, 21–29 CrossRef CAS PubMed
. - C. Shen, L. Wang, A. Zhou, B. Wang, X. Wang, W. Lian, Q. Hu, G. Qin and X. Liu, Synthesis and Electrochemical Properties of Two-Dimensional RGO/Ti3C2Tx Nanocomposites, Nanomaterials, 2018, 8, 80–88 CrossRef PubMed
. - Y. Liu, W. Wang, Y. Ying, Y. Wang and X. Peng, Binder-free layered Ti3C2/CNTs nanocomposite anodes with enhanced capacity and long-cycle life for lithium-ion batteries, Dalton Trans., 2015, 44, 7123–7126 RSC
. - Z. Wang, H. Guo, E. Liu, G. Yang and K. N. Win, Bismuth/Polyaniline/Glassy Carbon Electrodes Prepared with Different Protocols for Stripping Voltammetric Determination of Trace Cd and Pb in Solutions Having Surfactants, Electroanalysis, 2010, 22, 209–215 CrossRef CAS
. - L. Cui, J. Wu and H. Ju, Synthesis of Bismuth-Nanoparticle-Enriched Nanoporous Carbon on Graphene for Efficient Electrochemical Analysis of Heavy-Metal Ions, Chem.–Eur. J., 2015, 21, 11525–11530 CrossRef CAS PubMed
. - M. B. Gumpu, S. Sethuraman, U. M. Krishnan and J. B. B. Rayappan, A review on detection of heavy metal ions in water – an electrochemical approach, Sens. Actuators, B, 2015, 213, 515–533 CrossRef CAS
. - P. A. Dimovasilis and M. I. Prodromidis, Bismuth-dispersed xerogel-based composite films for trace Pb(II) and Cd(II) voltammetric determination, Anal. Chim. Acta, 2013, 769, 49–55 CrossRef CAS PubMed
. - L. Cui, J. Wu and H. Ju, Electrochemical sensing of heavy metal ions with inorganic, organic and bio-materials, Biosens. Bioelectron., 2015, 63, 276–286 CrossRef CAS PubMed
. - S. Lee, S. Bong, J. Ha, M. Kwak, S. K. Park and Y. Piao, Electrochemical deposition of bismuth on activated graphene-nafion composite for anodic stripping voltammetric determination of trace heavy metals, Sens. Actuators, B, 2015, 215, 62–69 CrossRef CAS
. - P. Niu, C. F. Sanchez, M. Gich, C. Ayora and A. Roig, Electroanalytical Assessment of Heavy Metals in Waters with Bismuth Nanoparticle-Porous Carbon Paste Electrodes, Electrochim. Acta, 2015, 165, 155–161 CrossRef CAS
. - P. K. Sahoo, B. Panigrahy, S. Sahoo, A. K. Satpati, D. Li and D. Bahadur, In situ synthesis and properties of reduced graphene oxide/Bi nanocomposites: as an electroactive material for analysis of heavy metals, Biosens. Bioelectron., 2013, 43, 293–296 CrossRef CAS PubMed
. - M. Cadevall, J. Ros and A. Merkoci, Bismuth nanoparticles integration into heavy metal electrochemical stripping sensor, Electrophoresis, 2015, 36, 1872–1879 CrossRef CAS PubMed
. - G. J. Lee, C. K. Kim, M. G. Lee and K. R. Chang, Simultaneous Voltammetric Determination of Zn, Cd and Pb at Bismuth Nanopowder Electrodes with Various Particle Size Distributions, Electroanalysis, 2010, 22, 530–535 CrossRef CAS
.
|
This journal is © The Royal Society of Chemistry 2020 |
Click here to see how this site uses Cookies. View our privacy policy here.