DOI:
10.1039/D0RA02361B
(Paper)
RSC Adv., 2020,
10, 15098-15106
Enhancement of floatability of low-rank coal using oxidized paraffin soap
Received
13th March 2020
, Accepted 26th March 2020
First published on 17th April 2020
Abstract
The processing of low-rank coal is becoming more urgent with depletion of high-rank coal and severe environmental pollution in China. The flotation of low-rank coal suffers poor efficiency with hydrocarbon oils unless it is at a high dose. A representative non-polar oil, diesel oil (DO), was used as a collector to float a long-frame coal. The feasibility of oxidized paraffin soap (OPS) was explored to improve flotation performance. Experimental measurements (polarized optical microscopy, zeta potential, Fourier transform infrared spectroscopy, X-ray photoelectron spectroscopy, contact-angle analysis) were used to explore the influence of OPS in low-rank-coal flotation. Poor flotation performances of coal samples were received using OPS or DO as collectors at an economical dose. The flotation efficiency of coal samples was improved considerably upon addition of OPS plus DO. OPS reduced the surface tension of aqueous solutions and promoted dispersion of DO in suspensions. Addition of OPS plus DO reduced the absolute value of the zeta potential on coal particles. Moreover, OPS improved DO adsorption on low-rank coal particles, and the hydrophobicity of low-rank coal was improved visibly. OPS reduced the contact angles of coal–bubble and coal–DO in aqueous solution, which enhanced the adhesion probability of particles to bubbles. This study suggests that OPS is an effective surfactant for improving the floatability of low-rank coal.
1. Introduction
Low-rank coals such as lignite and sub-bituminous coal are abundant in China, and account for >40% of proven coal reserves.1 With consumption of high-rank coal, low-rank coals are playing a more important part in energy supplies. “Coal fines” (CFs) refers to coal with a maximum particle size usually less than one-sixteenth inch and rarely above one-eighth inch. Large amounts of CFs are produced in mining and processing. Flotation is one of the most effective and economical methods used to purify CFs. However, a series of studies has shown that low-rank coals suffer from poor flotation efficiency with common oily collectors at an economical dose.2–5 Many polar oxygen-containing functional groups, such as carboxyl, phenolic and carbonyl, which have an affinity with water molecules via hydrogen bonding, are present on low-rank coal. Their presence results in poor hydrophobicity, deteriorating adhesion and spread of oily collectors onto coal surfaces.6 Furthermore, the porosity and pore permeability of CFs further increases consumption of oily collectors.7 Hence, conventional hydrocarbon oils are not effective and economical collectors in flotation of low-rank coal.
Recently, several attempts have been made by researchers to improve the collecting efficiency of low-rank coal.5,8–13 Exploration of effective collectors is believed to be economical for flotation promotion. Jia et al.6 employed tetrahydrofuran esters to improve the flotation performance of low-rank/oxidized coals. They found that reagents with benzene rings and oxygen functional groups were better collectors for floatation of low-rank coals. Chen et al.4 used 2-ethylhexanol to enhance the floatability of low-rank coal. They showed that the hydrophobicity of a coal surface increased visibly as the contact angle increased from 74.9° to 95.4°, and the induction time of particles and bubbles decreased from 246.0 ms to 85.4 ms simultaneously. Tian et al.9 applied carboxylic acids and alkanes as collectors to study the flotation efficiency of low-rank coal. They showed that better flotation performances could be achieved in the presence of carboxylic acids than that of alkanes. According to the results stated above, surfactants containing oxygen functional groups, such as aldehydes, ketones, and carboxylic acids, could be effective collectors to float low-rank coal. However, this strategy is restricted by the cost of synthesizing surfactants and adverse effects on the environment.
In addition, mixtures of hydrocarbon oils and polar reagents have been shown to be effective options to increase the collecting efficiency of low-rank CFs at a low dose.2,5,10,14,15 Vamvuka and Agridiotis16 employed a mixture of dodecylamine and kerosene as the collector to float lignite of size −300 ± 75 μm, and found that higher flotation selectivity and recovery could be achieved using this mixture. Xia et al.14 found that dodecane was adsorbed primarily on hydrophobic sites of the lignite surface whereas 4-Dodecylphenol was adsorbed primarily on the hydrophilic sites of the lignite surface. Hence, the flotation performance of lignite was improved using the mixture as collector. The results mentioned above suggest that mixtures of surfactants containing oxygen-functional groups and hydrocarbon oils increase the flotation performance of low-rank coals, and that they could be promising collectors for flotation of low-rank coal.
Oxidized paraffin soap (OPS) is a sodium soap containing fatty acids. It is made from paraffin soap through high-temperature oxidation. It contains ∼50% saturated carboxylic acid, 5–10% saturated hydroxyl fatty acids, and a small amount of alcohol, aldehydes and ketones. Thus, OPS may be an effective surfactant to improve the flotation performance of low-rank coal according to its chemical composition. Moreover, OPS is environmentally friendly because it is non-toxic and readily degradable. OPS has been used widely in the flotation of scheelite, hematite, apatite, and spodumene.17–19 Here, we focused on the influence of OPS on the flotation performance of low-rank coal using diesel oil (DO) as a collector. Furthermore, how OPS improves flotation of low-rank coal using DO as collector was discussed fully.
2. Materials and methods
2.1 Coal samples and reagents
Long-flame coal lumps with low-ash content were collected from Shendong Mine, Inner Mongolia, China. Some of the coal samples were crushed, ground and sieved to receive samples of size −0.5 mm for experiments. Some of the lumps were cut and polished for analyses of wettability and the contact angle. Proximate analyses of air-dried long-frame coal are shown in Table 1. Mad, Aad, Vad and FCad denote moisture content, ash content, volatile-matter content, and fixed-carbon content, respectively.
Table 1 Proximate analysis of low-rank coal (air-dried)
Mad, % |
Vad, % |
FCad, % |
Aad, % |
10.15 |
32.11 |
52.65 |
5.09 |
DO and OPS were employed as collectors to enhance the hydrophobicity of the coal surface. 2-Octanol was used as a frothing agent to reduce the surface tension of suspensions to generate more stable and small bubbles. Reagents were used without further purification. Deionized (DI) water was employed in all experiments unless stated otherwise.
2.2 Flotation
An XFD flotation machine (Alibaba, Beijing, China) with a cell of 0.5 L was used for coal flotation at a pulp density of 7.8% solids. When DO and OPS was used as a sole collector, the DO dose was 2, 4, 8, 10 and 12 kg t−1, and the dose of OPS was 0.5, 1, 2, 3, and 4 kg t−1. When both were used as collectors (OPS first and, 2 min later, DO was added), the dose of OPS was 0.5, 1 kg t−1, whereas the dose of DO was 1, 2, and 3 kg t−1. In all flotation experiments, the constant dose of 2-octanol was 0.5 kg t−1. The airflow rate was kept at 0.20 m3 (m2 min)−1. The impeller speed of the flotation machine was maintained at 1800 rpm.
2.3 DO dispersion
An optical microscope (Axio Scope A1; Zeiss, Oberkochen, Germany) equipped with digital camera was used to analyse dispersion of DO droplets in solutions. Solutions with DO (160 mg L−1) were conditioned for 60 min in the absence or presence of OPS (80 mg L−1). One droplet of the solutions was placed on a glass plate, and a thin glass slide was used to fix the emulsion. The plates were placed under an optical microscope and the oil droplets were photographed.
2.4 Zeta potential
Analyses of zeta potential of coal samples were conducted using a ZetaPALS system (Brookhaven Instruments, Holtsville, NY, USA). Suspensions containing 8.0 g of coal solids and 100 mL NaCl (1 mM L−1) as the background electrolyte were prepared in a beaker with agitation at 500 rpm for 4 min using a magnetic stirrer at a given pH. Then, the suspension was agitated for an additional 10 min after addition of DO or OPS (for addition of OPS and DO, OPS was added first and, 5 min later, DO was added and mixing allowed for another 5 min). After agitation, the suspension was allowed to stand for 24 h to allow coarse particles to settle. Next, 20 mL of the upper part of the suspension was used to conduct measurement of the zeta potential. For each sample, 30 measurements were taken and the average data reported.
2.5 Fourier transform infrared (FTIR) spectroscopy
Collection of attenuated total reflectance-FTIR data was conducted in a wave-number range of 4000–600 cm−1 on a Vertex 80v system (Bruker, Billerica, MA, USA). Coal samples with different reagents (DO, OPS, or OPS + DO) were conditioned for 5 min in DI water. Then, the suspensions were filtered and dried at 60 °C. Four coal samples were obtained: (a) raw coal conditioned with DI water (“raw coal”), (b) raw coal conditioned with DO (“DO coal”), (c) raw coal conditioned with OPS (“OPS coal”), and (d) raw coal conditioned with both OPS and DO (“OPS + DO coal”). FTIR spectra of coal samples were obtained without KBr.
2.6 X-ray photoelectron spectroscopy (XPS)
An XPS setup (ESCALAB 250Xi; Thermo Scientific, Waltham, MA, USA) was employed to analyse the surface composition of coal samples. Coal samples were ground to −0.074 μm to allow adsorption of OPS and DO on the coal surface. Suspensions in the absence and presence of collectors (DO 320 mg L−1; OPS 160 mg L−1; OPS 80 mg L−1 + DO 160 mg L−1) were conditioned for 10 min in a flotation cell, then filtered and dried. Dried powders were used to carry out XPS measurements. The detailed principles of XPS measurement are reported elsewhere.2 The C1s peak at 284.6 eV was employed to correct binding energies.
2.7 Wettability
A contact-angle analyser (DSA100; KRUSS, Hamburg, Germany) was applied to measure the contact angles of water droplets and coal plates using the pendant-droplet method. The detailed principles of measurement are reported elsewhere.20 A low-ash, low-rank coal lump was cut and polished to obtain several smooth coal plates. The latter were pre-treated with DI water, DO, OPS or both. The volume of a DI-water droplet was 6 μL. Images were recorded to analyse the contact angles of water droplets and coal plates.
2.8 Contact angle of coal–bubble/coal–oil
The contact angles of coal–bubbles and coal–DO in DI water were measured using the apparatus shown in Fig. 1. Coal plates with or without collector (DO (320 mg L−1); OPS (160 mg L−1); OPS (80 mg L−1) + DO (160 mg L−1)) pre-treatment were used to determine coal–bubble contact angles in DI water, and coal plates with or without OPS (80 mg L−1) pre-treatment were employed to determine coal–DO contact angles in DI water. First, the coal plates were inversed and immersed in DI water. Second, a bubble or DO droplet was injected. Then, the bubble or DO droplet floated to the coal surface and attached stably on the coal plate. The attachment process was recorded with a high-speed video.21,22 The frames were used to analyse the coal–bubble and coal–DO contact angles. All measurements were carried out in triplicate.
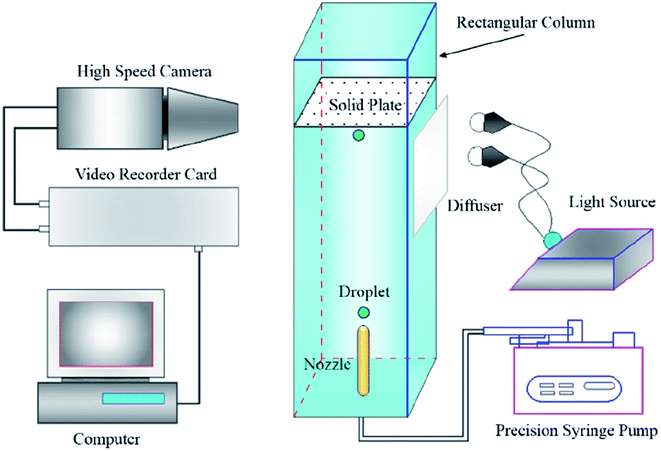 |
| Fig. 1 Apparatus of coal–collector/bubble contact-angle measurement in DI water. | |
3. Results and discussion
3.1 Flotation
The flotation results of low-rank coal as a function of DO or OPS dose are shown in Fig. 2(a). The yield of clean coal increased with DO dose, and a pleasing yield of clean coal was achieved with a larger amount of DO up to 12 kg t−1. Moreover, the yield of clean coal also increased with OPS dose, and the yield of clean coal was only 59.22% with a dose of 4 kg t−1. However, the yield of clean coal increased to an ideal value (Fig. 2(b)) with addition of OPS plus DO at a lower dose. A yield of 97.40% was achieved with sequential addition of 1 kg t−1 OPS and 3 kg t−1 DO. The flotation performance was enhanced significantly with addition of OPS plus DO. OPS reduced the dose of DO in flotation of low-rank coal to achieve a desired recovery of clean coal. The effect of OPS on flotation of low-rank coal using DO as a collector was investigated in depth by experimental analyses, as described below.
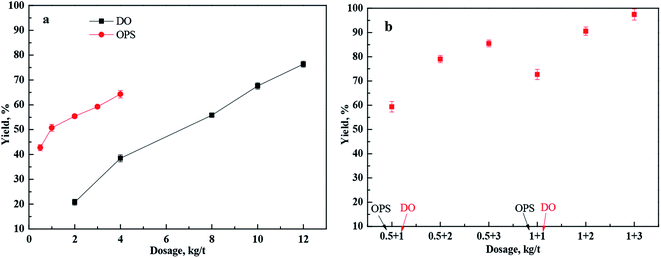 |
| Fig. 2 Flotation results of coal samples as a function of DO (a), OPS (a), and OPS + DO (b) dose. | |
3.2 DO dispersion
OPS is a water-soluble collector used for mineral flotation. Surface-tension changes were determined as a function of the OPS concentration (Fig. 3). Surface tension was reduced considerably upon OPS addition. When the concentration increased from 0 mg L−1 to 80 mg L−1, the surface tension of the solution decreased sharply from 72.23 mN m−1 to 38.81 mN m−1. When the concentration of OPS was increased further, there was a slight reduction in the surface tension of the solution.
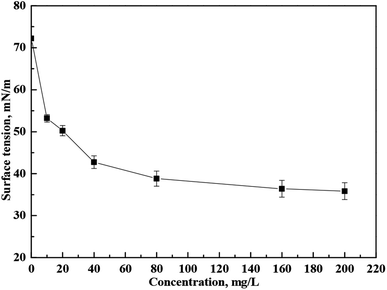 |
| Fig. 3 Change in surface tension as a function of OPS concentration. | |
When DO (160 mg L−1) was dispersed in DI water, the solution was cloudy. When DO (160 mg L−1) was dispersed in 80 mg L−1 OPS solution, the solution was clear (Fig. 4). The oil-droplet images obtained using the optical microscope (Fig. 5) showed some DO droplets in DI water but no noticeable DO droplets were in the OPS solution. Some researchers23,24 have found that smaller oil droplets increase the speed of particle collision, improve flotation recovery and increase the adsorption energy of oil onto coal surfaces. Xing et al.25 combined a flotation column with collector emulsification to purify a micro-fine, low-rank coal. They also found that collector emulsification increased the collision probability between the coal particle and diesel. Therefore, we deduced that, upon OPS addition, the surface tension of the solution decreased, and the size of oil droplets was minimized, which resulted in frequent collision between coal particles and DO. Hence, the flotation efficiency of low-rank coal was enhanced upon OPS addition.
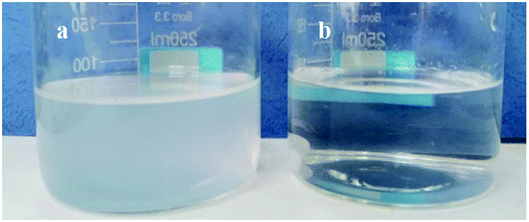 |
| Fig. 4 DO dispersed in DI water (a) and OPS solution (b). | |
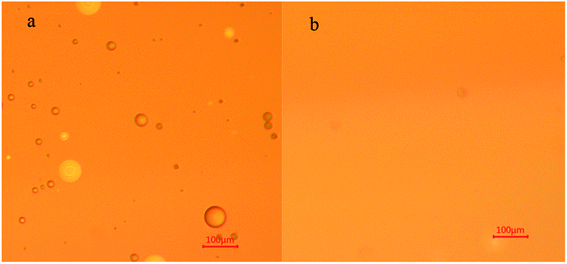 |
| Fig. 5 Microscopic photograph of DO droplets in DI water (a) and OPS solution (b). | |
3.3 Zeta potential
The zeta potential of coal samples as a function of DO and OPS concentrations is shown in Fig. 6. With increasing DO concentrations, the absolute value of the zeta potential of coal particles decreased gradually, whereas it increased slightly with increasing OPS concentrations. Oxygenated functional groups on coal surfaces leads to generation of electric potentials.21 DO is a hydrocarbon, and the oxygenated functional groups on the coal surface resulted in an absolute decrease in the zeta potential. OPS bonded with the oxygenated functional groups on the coal surface via hydrogen bonds. Hence, the –COOH bonded on the coal surface led to a zeta-potential change on coal particles. Addition of OPS plus DO decreased the absolute value of the zeta potential on coal samples considerably (Fig. 7). OPS improved the adsorption capacity of DO to coal particles, so the absolute value of the zeta potential on the coal surface decreased as more oxygenated functional groups became hindered. Bubbles in a flotation are negatively charged.26,27 The decrease in the absolute value of the zeta potential helped to improve the flotation performance of low-rank coal because of the lower electrostatic repulsion between particles and bubbles.3
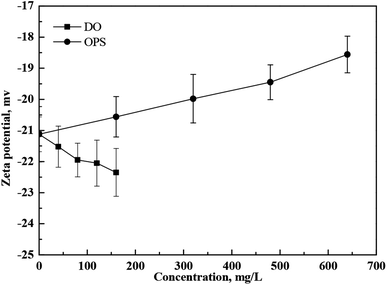 |
| Fig. 6 Zeta-potential changes of coal particles as a function of DO and OPS concentrations. | |
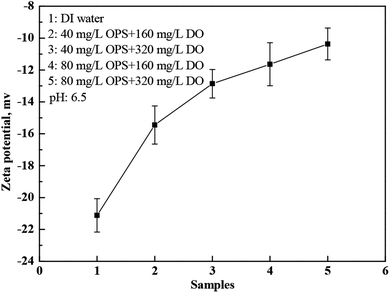 |
| Fig. 7 Zeta-potential changes of coal particles in DI water with and without addition of OPS plus DO. | |
3.4 Chemical composition of the coal surface
The FTIR spectra of coal samples are shown in Fig. 8. Spectral peaks at 3691 and 3621 cm−1 were the peaks of H2O.28 The hydrophobic groups of –CH3 and –CH2 were characterized by peaks around 2920, 2850, 1435, and 1374 cm−1. The hydrophilic groups of –C
O and –COOH were characterized by peaks ∼1587 cm−1.14,29 The peaks of hydrophobic groups for coal samples conditioned with DO, OPS, and OPS + DO were enhanced, especially the peaks at 2920 and 2850 cm−1, whereas the peaks of hydrophilic groups of –C
O and –COOH were decreased considerably (Fig. 8). A more pronounced change was observed when the coal sample was conditioned with OPS plus DO. Therefore, the flotation performance of coal samples using OPS plus DO as collectors was better than that using OPS and DO alone.
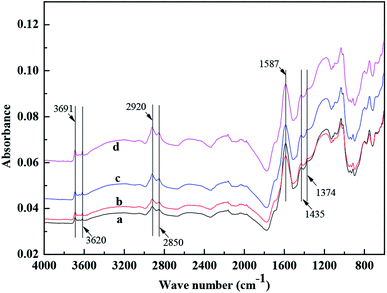 |
| Fig. 8 ATR-FTIR of raw coal (a), DO coal (b), OPS coal (c), and OPS + DO coal (d). | |
To identify the surface-chemistry changes of coal samples in the absence and presence of DO, OPS, or OPS + DO, XPS was employed to determine the surface functional groups of the low-rank coal. The XPS wide-energy spectra of coal samples are shown in Fig. 9 and, according to them, the semi-quantitative results of surface chemical composition are listed in Table 2. Apparently, the organic carbon contents of the DO coal, OPS coal, and OPS + DO coal were much higher, and the inorganic mineral element (Al, Si) contents of DO coal, OPS coal, and OPS + DO coal were much lower, than that of raw coal. Moreover, the oxygen content of OPS + DO coal was much lower than that of raw coal, DO coal and OPS coal. Adsorption of OPS + DO on the coal surfaces resulted in these phenomena. Addition of OPS plus DO improved the organic carbon elements and reduced the oxygen content on the coal surface visibly. Therefore, OPS addition was beneficial to DO adsorption on the raw coal surface, and this conclusion is consistent with the flotation results using OPS plus DO.
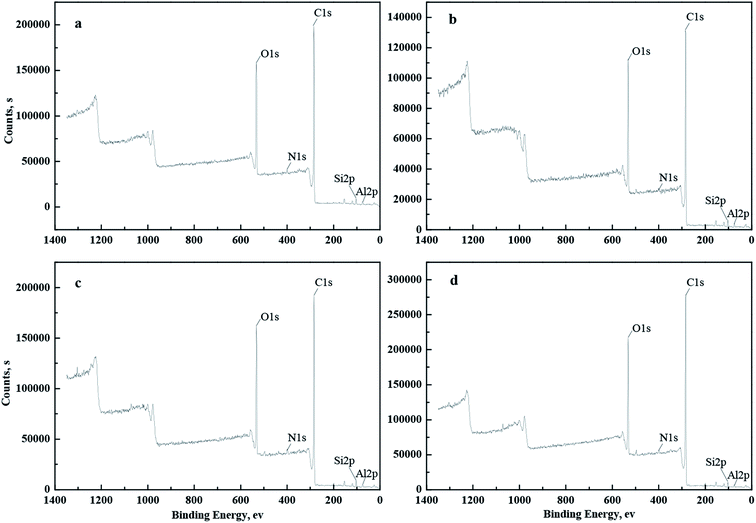 |
| Fig. 9 XPS wide-scan spectra of coal samples: (a) Raw coal, (b) DO coal, (c) OPS coal, and (d) OPS + DO coal. | |
Table 2 Surface chemical composition on coal samples (semi-quantitative)
Types |
C1s, % |
O1s, % |
N1s, % |
Si2p, % |
Al2p, % |
Raw coal |
71.03 |
20.61 |
2.5 |
2.81 |
2.84 |
DO coal |
71.67 |
20.31 |
2.99 |
2.64 |
1.54 |
OPS coal |
71.28 |
21.03 |
2.77 |
2.58 |
1.77 |
OPS + DO coal |
74.23 |
19.84 |
1.6 |
2.01 |
0.92 |
To identify the surface differences of coal samples further, fitting of C1s peaks was done. The fitting C1s peaks of coal samples are shown in Fig. 10. Peaks at about 284.60, 285.60 and 288.46 eV represent the groups of C–C and C–H, C–O, and C
O, respectively.30,31 The peak-split results of C1s on coal samples are shown in Table 3. Apparently, more C–H and C–C groups were determined on coal samples pre-treated with DO, OPS, and OPS + DO, and the number of C–O and C
O groups was also reduced. Moreover, addition of OPS plus DO further increased the number of C–C/C–H groups and reduced the number of C–O and C
O groups significantly. These findings were consistent with the results of XPS wide-energy spectra. These results provided further proof that OPS addition improved DO adsorption, which resulted in more C–H and C–C groups and fewer C–O and C
O groups on the coal surfaces.
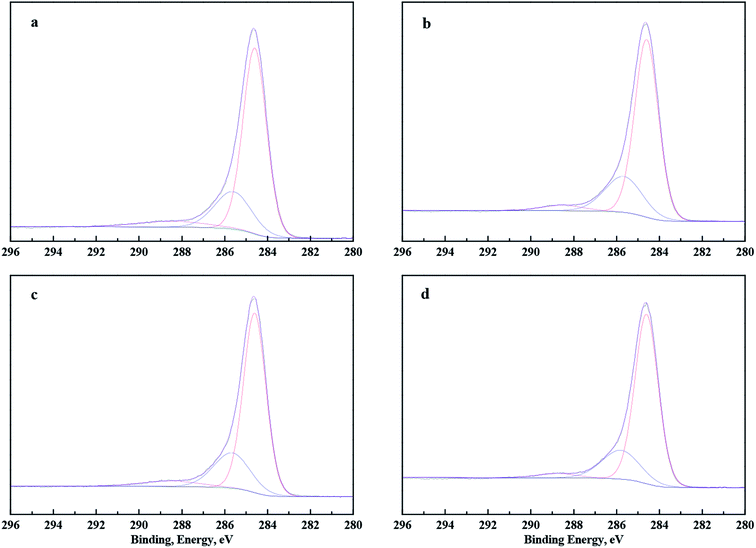 |
| Fig. 10 XPS C 1s spectra of raw coal (a), DO coal (b), OPS coal (c), and OPS + DO coal (d). | |
Table 3 Peak-split results of C on coal samples
Types |
C–C/C–H, % |
C–O, % |
C O, % |
Raw coal |
70.90 |
22.47 |
6.63 |
DO coal |
72.73 |
23.38 |
3.89 |
OPS coal |
71.63 |
22.71 |
5.66 |
OPS + DO coal |
75.76 |
21.07 |
3.17 |
In conclusion, sole addition of DO and OPS increased the number of C–C and C–H groups on the coal surface. Moreover, addition of OPS plus DO further increased the number of C–C and C–H groups, and reduced the number of C–O and C
O groups on a low-rank coal surface.
3.5 Wettability changes
To reflect hydrophobicity changes of coal samples before and after pre-treatment using DO, OPS, or OPS + DO, a contact-angle analyser was used to analyse the wettability of the coal plates (Fig. 11). The contact angle of raw coal was only 56°, which indicated that there was strong hydrophilicity for a low-rank coal surface. When the plates were pre-treated with DO solution (320 mg L−1) and OPS solution (160 mg L−1), respectively, the contact angle increased to 66° and 63°, and the hydrophobicity of coal samples was enhanced. When the coal plates were pre-treated with OPS (80 mg L−1) and DO (160 mg L−1) in sequence, the contact angle increased to 81°, which indicated that the hydrophobicity of the coal sample was enhanced significantly. Xia et al.32 studied the effect of candle soot and hydrocarbon oil on coal flotation. They found that candle soot enhanced the hydrophobicity of the coal surface, so the floatability of coal particles was increased. As shown above, the hydrophobicity of low-rank coal was improved upon addition of OPS plus DO, which was consistent with the flotation results.
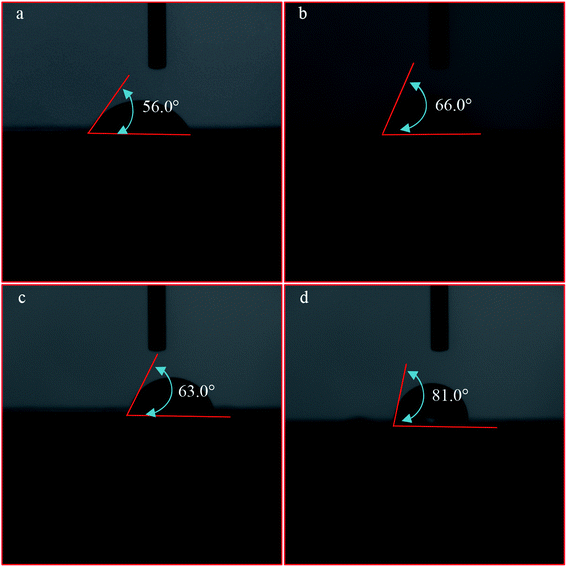 |
| Fig. 11 Contact angle of low-rank coal pretreated with DI water (a), DO (b), OPS (c), and OPS + DO (d). | |
3.6 Coal–bubble and coal–oil contact angles
As shown above, OPS application affected DO adsorption on a low-rank coal surface. Hence, the contact angles of coal–bubble and coal–DO in DI water were measured in the absence and presence of OPS. Contact angles of bubbles on the low-rank coal before and after OPS pre-treatment changed significantly from 142° to 116.3° (Fig. 12). There are large attractive forces between a high-hydrophobicity particle surface and bubbles.33,34 As shown above, OPS improved the hydrophobicity of the low-rank coal. Thus, we deduced that the attractive forces between coal particles and bubbles were intensified upon OPS addition. This finding was in good agreement with the flotation results using OPS as a collector. There was a significant change, from 131.1° to 106.1°, for the contact angles of DO droplets and coal plates pre-treated with and without OPS (Fig. 13). This finding suggested that OPS was beneficial for DO adsorption on coal particles. The oleophilic property of a substrate is very conducive to the spread of oil droplets on a solid surface.35 OPS addition increased the number of C–C/C–H groups on the low-rank coal and increased its hydrophobicity. Hence, the spread of oil droplets on the coal surface was enhanced by OPS. This finding was in accordance with flotation results using OPS plus DO.
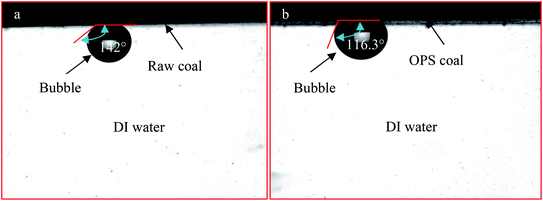 |
| Fig. 12 Coal–bubble contact angle in deionized water: (a) raw coal and (b) OPS coal. | |
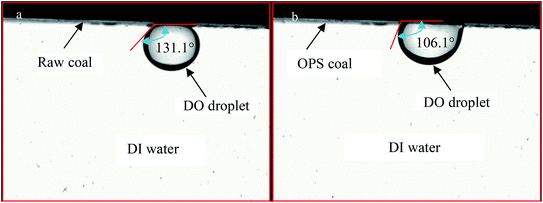 |
| Fig. 13 Contact angle of coal–DO droplets in DI water: (a) raw coal and (b) OPS coal. | |
4. Conclusions
The influence of OPS on flotation performance of low-rank coal was studied. Several advanced methods were utilized to reveal the mechanisms underlying the improved effect of OPS upon coal flotation. We drew five main conclusions.
(1) A desired yield of clean coal was not obtained using OPS or DO as a collector at an economical dose. Flotation recovery of low-rank coal was improved considerably upon addition of OPS plus DO.
(2) OPS reduced the surface tension of an aqueous solution and DO dispersion in suspensions was promoted.
(3) Addition of both OPS and DO reduced the absolute value of the zeta potential on the coal surface.
(4) OPS improved DO adsorption on the coal surface and the hydrophobicity of low-rank coal was improved visibly.
(5) OPS reduced the contact angles of coal–bubble and coal–DO droplets in aqueous solution, and enhanced the adhesion probability of particles to bubbles.
Conflicts of interest
There are no conflicts to declare.
Acknowledgements
Fundamental Research Funds for the Central Universities (Grant no. 2019QNA11) supported the research, for which the authors express their appreciation.
References
- H. Hu, M. Li, L. Li and X. Tao, J. China Univ. Min. Technol., 2019, 30(2), 217–223 Search PubMed.
- Y. Xia, R. Zhang, Y. Xing and X. Gui, Fuel, 2019, 235, 687–695 CrossRef CAS.
- Y. Xia, Z. Yang, R. Zhang, Y. Xing and X. Gui, Fuel, 2019, 239, 717–725 CrossRef CAS.
- S. Chen, L. Tang, X. Tao, H. He, L. Chen and Z. Yang, Powder Technol., 2017, 167, 61–67 CrossRef CAS.
- S. Dey, Fuel Process. Technol., 2012, 94, 151–158 CrossRef CAS.
- R. Jia, G. H. Harris and D. W. Fuerstenau, Int. J. Miner. Process., 2000, 58, 99–118 CrossRef CAS.
- Z. Yang, Y. Xia, M. Li, Z. Ma, Y. Xing and X. Gui, Fuel, 2019, 239, 63–69 CrossRef CAS.
- X. Lyu, X. You, M. He, W. Zhang, H. Wei, L. Li and Q. He, Fuel, 2018, 211, 529–534 CrossRef CAS.
- Q. Tian, Y. Zhang, G. Li and Y. Wang, Int. J. Coal Prep. Util., 2017, 39, 44–53 CrossRef.
- R. Jia, G. H. Harris and D. W. Fuerstenau, Int. J. Coal Prep. Util., 2002, 22, 123–149 CrossRef CAS.
- G. Cheng, Z. Li, Y. Cao and Z. Jiang, Int. J. Coal Prep. Util., 2020, 4, 59–76 CrossRef.
- B. Wen, W. Xia and J. M. Sokolovic, Powder Technol., 2017, 319, 1–11 CrossRef CAS.
- P. Meshram, B. K. Purohit, M. K. Sinha, S. K. Sahu and B. D. Pandey, Renewable Sustainable Energy Rev., 2015, 41, 745–761 CrossRef CAS.
- W. Xia, C. Ni and G. Xie, Int. J. Coal Prep. Util., 2015, 36, 262–271 CrossRef.
- X. You, M. He, W. Zhang, H. Wei, X. Lyu, Q. He and L. Li, Powder Technol., 2018, 332, 323–330 CrossRef CAS.
- D. Vamvuka and V. Agridiotis, Int. J. Miner. Process., 2001, 61, 209–224 CrossRef CAS.
- L. Xu, Y. Hu, J. Tian, H. Wu, Y. Yang, X. Zeng, Z. Wang and J. Wang, Miner. Eng., 2016, 89, 84–92 CrossRef CAS.
- Z. Gao, D. Bai, W. Sun, X. Cao and Y. Hu, Miner. Eng., 2015, 72, 23–26 CrossRef CAS.
- C. Liu, Q. Feng, G. Zhang, W. Chen and Y. Chen, Int. J. Miner. Process., 2016, 157, 210–215 CrossRef CAS.
- S. Li, X. Ma, J. Wang, Y. Xing, X. Gui and Y. Cao, Miner. Eng., 2020, 146, 106146 CrossRef.
- Y. Yu, J. Liu, R. Wang, J. Zhou and K. Cen, Energy Convers. Manage., 2012, 57, 8–12 CrossRef CAS.
- J. Wang, Y. Cao, Y. Xing, G. Li, Y. Liao, S. Li and M. An, J. Dispersion Sci. Technol., 2019, 1–7, DOI:10.1080/01932691.2019.1645025.
- W. Xie, K. Huang, D. Wang, Y. Zhang and X. Liu, Energy Procedia, 2012, 14, 750–755 CrossRef CAS.
- X. Q. Wu, R. J. Gochin and A. J. Monhemius, Int. J. Miner. Process., 2004, 74, 327–336 CrossRef CAS.
- Y. Xing, X. Gui, Y. Cao, D. Wang and H. Zhang, J. Cleaner Prod., 2016, 153, 657–672 CrossRef.
- A. M. Elmahdy, M. Mirnezami and J. A. Finch, Int. J. Miner. Process., 2008, 89, 40–43 CrossRef CAS.
- A. Bueno-Tokunaga, R. Pérez-Garibay and D. Martínez-Carrillo, Int. J. Miner. Process., 2015, 140, 50–57 CrossRef CAS.
- J. He, C. Liu and Y. Yao, Powder Technol., 2018, 325, 333–339 CrossRef CAS.
- O. Ozdemir, O. F. Ersoy, O. Guven, H. Turgut, M. Cinar and M. S. Çelik, Physicochem. Probl. Miner. Process., 2018, 54, 1070–1082 CAS.
- B. Wang, Y. Peng and S. Vink, Energy Fuels, 2013, 27, 4869–4874 CrossRef CAS.
- W. Xia, C. Niu and C. Ren, J. Cleaner Prod., 2017, 168, 1032–1038 CrossRef CAS.
- W. Xia, Y. Li and A. V. Nguyen, J. Cleaner Prod., 2018, 195, 1183–1189 CrossRef CAS.
- C. Shi, X. Cui, L. Xie, Q. Liu, D. Y. C. Chan, J. N. Israelachvili and Z. Hongbo, ACS Nano, 2015, 9, 95–104 CrossRef CAS PubMed.
- N. Ishida, Colloids Surf., A, 2007, 300, 293–299 CrossRef CAS.
- Y. Han, Z. Yang, L. He, X. Luo, R. Zhou, K. Shi and J. Su, Colloids Surf., A, 2018, 543, 15–27 CrossRef CAS.
|
This journal is © The Royal Society of Chemistry 2020 |
Click here to see how this site uses Cookies. View our privacy policy here.