DOI:
10.1039/D0RA01922D
(Paper)
RSC Adv., 2020,
10, 15870-15880
A new Bi2MoO6 nano-tremella-based electrochemical immunosensor for the sensitive detection of a carcinoembryonic antigen†
Received
28th February 2020
, Accepted 2nd April 2020
First published on 21st April 2020
Abstract
By doping molybdenum (Mo) into bismuth oxide (Bi2O3), we synthesized novel Bi2MoO6 nanohybrids with a unique tremella-like crystal structure via a one-pot hydrothermal method. Such a porous tremella structure of Bi2MoO6 possesses a significantly larger surface area than the spherical structure of Bi2O3, which is favorable for the special functionalization and effective immobilization of molecules such as antibodies on the surface of the tremella flaps. As expected, Au nanoparticles grew easily and uniformly outside the surface of the flaps of the as-prepared Bi2MoO6 NTs (Au@Bi2MoO6 NTs), showing preferable conductivity and biocompatibility, and the anti-carcinoembryonic antigen (anti-CEA) was effectively immobilized outside the obtained Au@Bi2MoO6 NTs. Acting as a novel label-free immunosensing platform, the anti-CEA-immobilized Au@Bi2MoO6 NTs were used to fabricate an electrochemical immunosensor for the quantitative detection of CEA. The as-prepared Au@Bi2MoO6-based immunosensor linearly responded to CEA in a wide concentration range from 1 pg mL−1 to 1 μg mL−1 with a detection limit calculated to be 0.3 pg mL−1 (S/N = 3), significantly comparable to numerous existing immunosensors. Moreover, the minimum detection concentration of the designed immunosensor was about ten times smaller than that of many Bi and Mo-based sensors toward CEA detection, and the detection limit was lower, revealing higher sensitivity. The satisfactory selectivity and stability made the immunosensor potentially applicable for CEA detection in real samples.
1. Introduction
Cancer is the most common type of malignancy without obvious non-specific symptoms in the early stage of malignant tumors.1,2 We are far from blocking the pace of cancer despite numerous progress achieved in cancer research over the years. However, early diagnosis of cancer can significantly improve the cure and survival rate.3,4 Common cancer screening methods include genetic testing, imaging examination, endoscopy, pathological examination, and tumor marker detection.5 Tumor marker detection is the most effective and potential means of detecting cancer at an early stage, which is mainly carried out via enzymatic examination. For example, liver cancer and lung cancer can be significantly screened by detecting alkaline phosphatase and glycoproteins, respectively. The occurrence of liver cancer and malignant teratoma can result in an increase in alpha-fetoprotein (AFP).6 Lung, colorectal and breast cancer can be diagnosed and evaluated early by detecting the concentration of carcinoembryonic antigen (CEA).7–10 Therefore, the quick and sensitive detection of tumor markers is significantly important for the early diagnosis and treatment of cancer.
In recent years, electrochemical immunosensors have received considerable attention due to their numerous advantages such as simplicity, sensitivity, and low cost.11 Voltammetric immunosensors usually use an electrolyte solution containing reversible redox species such as ferricyanide ([Fe(CN)6]3−/4−) for good reversible oxidation–reduction.12 On the other hand, unlabeled voltammetric immunosensors are fabricated by dropping the redox substance on the electrode surface to bind the antibody through chemical bonds, thereby eliminating the direct contact of the electrode with the antibody.12 The key of this approach is the selection of the material modifying the electrode so as to accelerate electron transfer and amplify the signal. Therefore, numerous materials were chosen to improve the sensitivity of the immunosensor. For example, graphene oxide is widely used because of its low cost, large specific surface area, excellent mechanical properties, good electronic properties and excellent thermal properties.7,13–15 Apart from this, mesoporous silica (SiO2),16 photoactive conducting poly(5-formylindole) nanocomposites,17 magnetic nanomaterials (Fe2O3, Fe3O4, etc.),18,19 ceria (CeO2),20 cuprous oxide (Cu2O)21 and platinum (Pt)22 are commonly used for electrode modification.
Usually, the materials with poor biocompatibility can inevitably cause toxicity, irritation, teratogenicity, and local inflammation of cells and the whole body, after undergoing a short-term contact, while the long-term contact may exert mutagenic, teratogenic and even carcinogenic effects. Also, the transportation of such materials through blood may cause abnormal coagulation function and hemolysis. Therefore, biocompatibility is a significantly important indicator to be evaluated for materials used in biological applications, including biosensors.23,24 Until now, the application of bismuth (Bi)-based nanomaterials in biosensors has attracted increasing attention due to their good stability in nature and extremely low toxicity to the body. For instance, Han used the cerium-doped CdS-sensitized BiYWO6 to detect tetracycline, reducing the detection limit to 0.01 ng mL−1 with good stability.25 Wu et al. developed a label-free photoelectrochemical (PEC) immunosensor based on CdS nanowires sensitized with a WO3@BiOI heterostructure for CEA detection with good stability and high sensitivity.26 An Ag ion-modified bismuth vanadate nano-polyhedron (BiVO4@Ag+) was utilized as a label to immobilize anti-AFB1 through affinity specific binding.27 Among those Bi-based materials, Bi2O3 is an important semiconductor material because of its narrow band-gap (about 2.8 eV), relatively high power and capacity, and electrochemical stability.28 The Bi2O3 nanoparticles (NPs) synthesized via a hot solvent method have good photocatalytic performance.29 Also, polycrystalline Bi2O3 was used to construct a new nano-bio-based amperometric glucose biosensor,30 and Bi2O3 nanorods were produced to modify an indium–tin-oxide electrode for the quantitative detection of mycotoxin,31 revealing the good biocompatibility of Bi2O3. However, the conductivity of Bi2O3 is not good enough and somehow limits its application in an electrochemical analysis. Moreover, molybdenum (Mo) containing nanomaterials have received considerable attention in recent years due to their good biocompatibility and high metallic-like electrical conductivity. The palladium NP-functionalized three-dimensional wrinkly amorphous MoSx composites were used as an electrochemical immunosensing platform to determine insulin.32 The hierarchically 1D MoO2 particles exhibited remarkable electrocatalytic activity with good long-term cycle stability for the hydrogen evolution reaction (HER) in acidic media.33 An amino-functionalized coral-like MoS2@Cu2O hybrid with increased surface area and conductivity was used to amplify the signal.34 Gao successfully developed Au@Pd/MoS2-modified multi-walled carbon nanotubes (MWCNTs) to improve high specific surface area and good biocompatibility.35
Usually, Bi2O3 shows a spherical or nanowire structure28,36 with a relatively small specific surface area and low electron transfer rate, which is not favorable for the amplification of electrical signals, the effective immobilization of biomolecules and even the deposition of other NPs. In this study, Mo was doped into Bi2O3 to prepare a Bi-based hybrid material (Bi2MoO6) via a one-pot hydrothermal method. As expected, the spherical structure of Bi2O3 was changed to a porous tremella-like structure (Bi2MoO6 NTs) with a large surface area by incorporating Mo into Bi2O3, which is beneficial for the metal NPs to grow and the antibody to bind on the flap surface of the Bi2MoO6 NTs specifically. By comparison, both the electron transfer and biocompatibility of Bi2MoO6 NTs were significantly improved, which was favorable for the fabrication of an electrochemical immunosensor. As expected, Au NPs easily grew on the flaps of Bi2MoO6 NTs (Au@Bi2MoO6 NTs), further enhancing the electron transfer performance and biocompatibility of the hybrid Bi2MoO6 NTs. To the best of our knowledge, it is the first time to synthesize tremella-like Bi2MoO6 nanohybrid and use it as an electrochemical immunosensing platform to detect CEA, where the excellent analytical performance was achieved toward CEA detection. Scheme 1 illustrates the preparation of the Bi2MoO6 NT-based electrochemical immunosensor toward CEA detection.
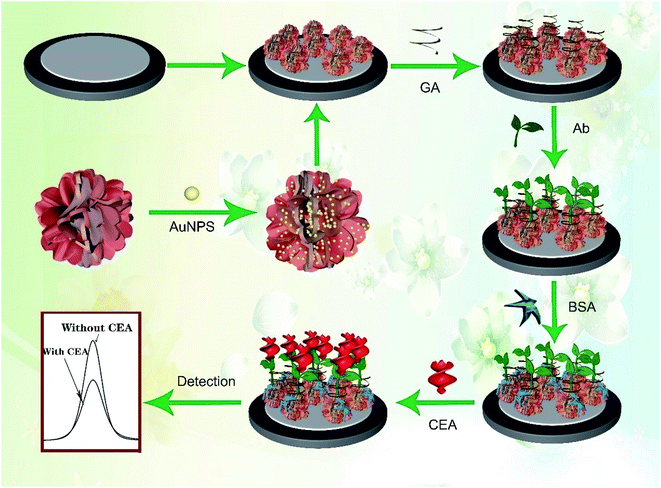 |
| Scheme 1 Schematic of the preparation of the Bi2MoO6 NT-based electrochemical immunosensor toward CEA detection. | |
2. Experimental
2.1. Reagents
The antibody of CEA (anti-CEA), CEA, prostate-specific antigen (PSA), immunoglobulin G (IgG), albumin from bovine serum (BSA) and AFP were purchased from Shanghai Linc-Bio Science Co. Ltd (Shanghai, China). Barium nitrate pentahydrate (Bi(NO3)3·5H2O), concentrated HNO3 (65–68%), polyvinylpyrrolidone (PVP), NaOH (99.99%), ethylene glycol (EG, >99%), glutaraldehyde (GA), CTAB, potassium hexacyanoferrate(II) trihydrate (K4Fe(CN)6), potassium hexacyanoferrate(III) (K3Fe(CN)6), sodium borohydride (NaBH4), sodium citrate, chloroauric acid (HAuCl4), trisodium citrate dihydrate (Na3C6H5O7·2H2O), potassium chloroaurate (KAuCl4), ascorbic acid (AA), 3-aminopropyl)triethoxysilane (APTES), disodium phosphate (Na2HPO4) and sodium dihydrogen phosphate(NaH2PO4) were obtained from Aladdin Industrial Corporation (Shanghai, China). Molybdenum acetylacetonate ((MoO2(acac)2) was provided by Tansoole (Shanghai, China). All other chemicals were of analytical reagent grade and used without any further treatment. Deionized water and absolute ethanol were used throughout the experiment.
2.2. Apparatus
Scanning electron microscopy (SEM) measurements were performed on an FEI QUANTA FEG250 coupled to an INCA Energy X-MAX-50. X-ray photoelectron spectroscopy (XPS) measurements were carried out using a single-color AlKα source with ESCALAB 250 XI. UV-Vis absorption spectra were recorded on a UV-Vis 1901 spectrophotometer (Phoenix). All electrochemical measurements were accomplished on a CHI660D workstation (Chenhua Instrument Shanghai Co., Ltd, China). X-ray diffraction (XRD) were obtained on a Rigaku Ultimate IV (Rigaku, Japan).
2.3. Preparation of the Bi2O3 nanocrystals
0.182 g of Bi(NO3)3·5H2O was dissolved in 5 mL 1.0 mol L−1 HNO3 solution, immediately followed by the addition of 0.064 g NaOH, 0.6 g PVP and 25 mL EG. The obtained solution was then transferred to a 50 mL Teflon reactor, which was sealed in a stainless steel autoclave and heated at 150 °C for 3 h. The cooled solution was centrifuged at 10
000 rpm for 10 min to collect Bi2O3 NPs, which were then thoroughly washed and freeze-dried for use.36
2.4. Preparation of the MoO2 nanocrystals
Typically, 0.08 g MoO2(acac)2 was gradually dissolved in 20 mL of a mixed solution containing absolute ethanol and 5 mL deionized water in a molar ratio of 3
:
1 under stirring. After stirring for 2 h, the solution was heated to 180 °C and kept for 10 h. Next, the solution was cooled to room temperature and then centrifuged to obtain the precipitate of MoO2 nanocrystals, which was washed with ethanol several times and freeze-dried for subsequent use.37
2.5. Preparation of the Bi2MoO6 NTs
The Bi2MoO6 NTs were prepared via a one-pot synthesis method. First, 0.05 g Bi (NO3)3·5H2O was dissolved in 20 mL absolute ethanol and stirred for 1 h. Then, 0.08 g MoO2(acac)2 was added into the solution, followed by 1 h stirring. Next, 0.5 g CTAB was successively dissolved in the mixture. After stirring for 1 h, the solution was transferred into a Teflon-lined stainless-steel autoclave for 12 h at 160 °C. Bi2MoO6 NTs were finally obtained by centrifuging the solution at 10
000 rpm for 10 min, which were then washed with ethanol and ultrapure water three times and dried in a drying oven at 60 °C for 8 h.
2.6. Amino functionalization of Bi2MoO6 NTs and preparation of gold seeds
0.012 g Bi2MoO6 NTs were dispersed into 30 mL absolute ethanol, followed by the addition of 1.0 mL of ATPES under stirring. After 3 h of stirring, the solution was refluxed at 70 °C for 1.5 h under stirring for the amino functionalization. Finally, the solution was centrifuged at 10
000 rpm for 5 min to collect the NH2 group-functionalized Bi2MoO6 NTs (Bi2MoO6–NH2), which were dispersed in 15 mL absolute ethanol for subsequent use.
A solution of gold seeds was prepared based on a previously reported method.38 Briefly, 200 μL of a 10 mM HAuCl4 solution was added into 10 mL of deionized water, and 200 μL of 10 mM trisodium citrate was then rapidly added in under stirring. 10 min later, 200 μL of a 100 mM NaBH4 solution was pipetted into the mixture, which was stirred for 30 min till the solution color changed to red.
2.7. Modification of Bi2MoO6 NTs with Au NPs
First, 1.0 mL of the above prepared Bi2MoO6–NH2 NTs was ultrasonically dispersed in 10 mL of absolute ethanol. Then, a 60 μL gold nanoseed-containing solution was subsequently added under stirring, immediately followed by the addition of a 300 μL 10 mM HAuCl4 solution. Second, 300 μL of 10 mM sodium citrate was added into the mixed solution. After the solution was stirred for 5 min, 100 μL 0.1 M AA was pipetted into the mixed solution to allow the growth of Au NPs for 30 min. Finally, the resultant product was centrifuged repeatedly at 10
000 rpm for 10 min with ethanol. After being dried in an oven at 60 °C for 8 h, the final Au NP-modified Bi2MoO6 NTs (Au@Bi2MoO6 NTs) were stored at room temperature for subsequent use. The schematic of the synthetic material is fully illustrated in Scheme 2.
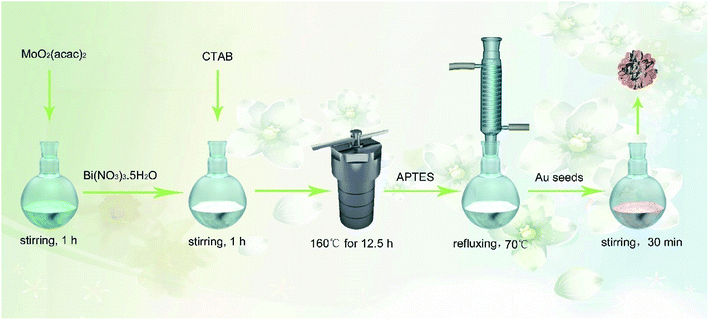 |
| Scheme 2 The schematic for the synthesis of Au@Bi2MoO6 NTs. | |
2.8. Immunoassay of CEA based on Au@Bi2MoO6 NTs
After the bare GCE was polished with 0.3 μm and 0.05 μm alumina slurry, respectively, and dried at room temperature, a certain amount of Au@Bi2MoO6 NTs was dropped onto the electrode surface. 0.2 mM GA (2.5%) was dropped on the Au@Bi2MoO6 NT-modified GCE at 30 °C for 1 h as a cross-linker to connect anti-CEA. Afterward, 6 μl of 75 ng mL−1 anti-CEA was dropped on the surface of the functionalized electrode and incubated at 30 °C for 1 h, followed by washing with PBS and air-drying at 30 °C. Then, 6 μL BSA (1%) was pipetted on the surface of the anti-CEA immobilized electrode surface and incubated for 60 min to cover the inactive sites. After being washed with PBS and air-dried sequentially, the Au@Bi2MoO6 NT-based electrochemical immunosensor was finally obtained and stored at 4 °C for CEA detection. Scheme 1 shows the fabrication of the Au@Bi2MoO6 NT-based immunosensor toward CEA detection.
2.9. Electrochemical measurements
All the electrochemical measurements were carried out in the solution of PBS (pH = 6.5) containing 5 mM [Fe(CN)6]3−/4− and 0.1 M KCl through a three-electrode system using a differently modified glassy carbon electrode (GCE, d = 3 mm), a platinum wire and a silver/silver chloride (Ag/AgCl) as the working, auxiliary and reference electrodes, respectively. The cyclic voltammetries (CV) were obtained in the scanning potential range from −0.2 to 0.8 V at a sweep speed of 0.1 V s−1. The square wave voltammetry plots were performed in the scan range from 0.0 to 0.6 V. The electrochemical impedance spectroscopy (EIS) was scanned from 0.01 to 100000 Hz (amplitude: 0.005 V, quiet time: 2 s). Before the detection, the as-prepared Au@Bi2MoO6 NT-based immunosensor was incubated with CEA at different concentrations at 30 °C for 60 min. Right after the immune combination between anti-CEA and CEA, the electrodes were washed with PBS to remove the unbound CEA.
3. Results and discussion
3.1. Characterizations of Bi2MoO6 NTs and Au@Bi2MoO6 NTs
3.1.1 SEM and XRD analysis. By comparison, both Bi2O3 and MoO2 were synthesized, showing a spherical shape with a particle size of about 300 nm (Fig. 1A and B). After Mo and Bi were mixed to prepare the hybrid, the morphology of the hybrid changed greatly, from the original sphere to a tremella-like nanostructure (Fig. 1C and D, the inset is a photo of real tremella). Although the particle size (950–1000 nm) increased, the surface area of the obtained porous Bi2MoO6 NTs was greatly enlarged due to the formation of a tremella structure. The change in morphology indicated that the two elements were not simply mixed, but formed a nanohybrid. More importantly, such a porous tremella nanostructure is good for the further surface modification of Bi2MoO6. Fig. S2† shows the XRD patterns of the as-prepared Bi2MoO6 and pure Bi2O3. The peaks from left to right correspond to (131), (002), (210), (212), (260), (331), (133) and (391) of Bi2MoO6 (JCPDS no. 84-0787), a single orthorhombic crystal, confirming the successful preparation of the Bi2MoO6 hybrid crystal. In addition, Au NPs with an average diameter of 50 nm were easily and uniformly decorated on the flap surface of Au@Bi2MoO6 NTs as expected (Fig. 1E and F).
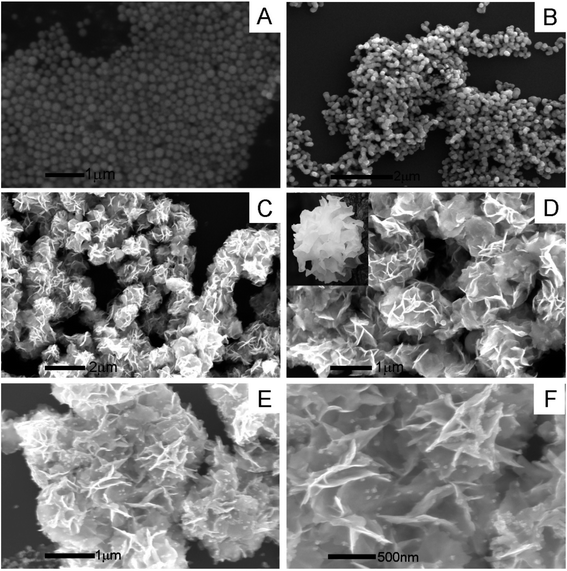 |
| Fig. 1 SEM images of (A) Bi2O3, (B) MoO2, (C and D) Bi2MoO6, and (E and F) Au@Bi2MoO6 in different magnification scales. | |
Moreover, the mapping analysis of Au@Bi2MoO6 NTs indicated that the metals of Bi, Mo and Au were uniformly distributed throughout Au@Bi2MoO6 NTs (Fig. 2), verifying the successful synthesis of the Bi2MoO6 nanohybrid and the uniform distribution of Au NPs on the flap surface of Bi2MoO6 NTs.
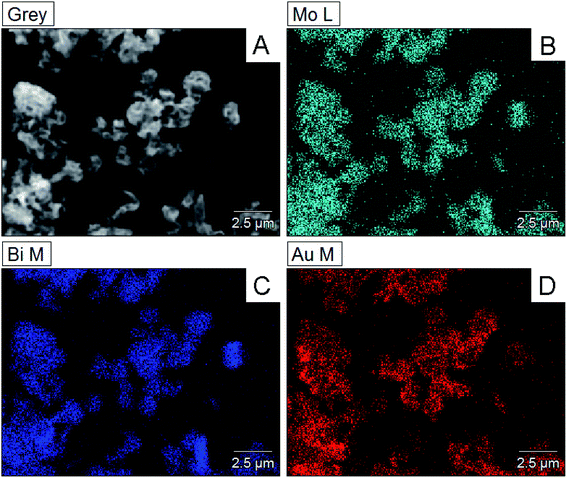 |
| Fig. 2 The mapping analysis of (A) Au@Bi2MoO6; and elements of (B) Mo, (C) Bi and (D) Au. | |
3.1.2 XPS and UV characterizations. XPS was also performed to determine the elemental composition of the as-prepared Au@Bi2MoO6 NTs, as shown in Fig. 3. The binding peaks of Mo, Bi, O and Au elements are observed in the survey spectrum (Fig. 3A), confirming their coexistence inside the Au@Bi2MoO6 NTs. In the fitted spectrum of Mo3d (Fig. 3B), the two peaks at 235.05 eV and 235.1 eV correspond to the binding between Mo and O. The Bi–O bond is reflected by the presence of a binding peak at 164.15 eV and 158.85 eV, as shown in Fig. 3C, the fitted spectrum of Bi4f.39 Also, the binding of O with both Bi and Mo was proved by the presence of a peak at 531.95 eV in the O1s fitted spectrum (Fig. 3E). All the results were indicative of the successful formation of Bi2MoO6 NTs. In addition, in Fig. 3D, the Au4f spectrum represented the XPS signature of the Au4f doublet (4f5/2 and 4f7/2) at 87.00 eV and 83.35 eV, suggesting the presence of Au NPs in Bi2MoO6 NTs. Fig. 3F shows the UV-vis absorption spectra of Bi2MoO6 NTs before and after the modification of Au NPs. By comparison, a strong absorption peak at 570 nm is observed for Bi2MoO6 NTs after Au NPs grew outside, further confirming the formation of Au NPs on Bi2MoO6 NTs.
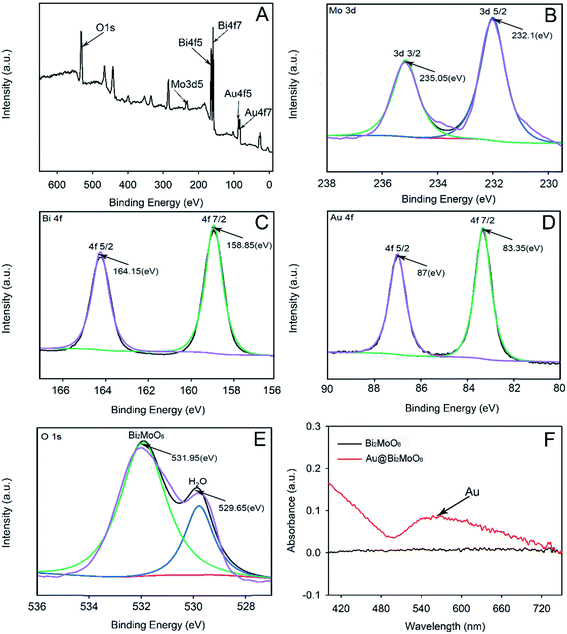 |
| Fig. 3 XPS spectra of the Bi2MoO6 NTs. (A) Survey spectrum of Bi2MoO6 NTs and the fitted spectra of (B) Mo3d, (C) Bi4f, (D) Au4f, (E) O1s. (F) UV spectra of Bi2MoO6 NTs and Au@Bi2MoO6 NTs. | |
3.1.3 Properties of electron transfer and biocompatibility. Fig. 4A and B show the CV and EIS plots of bare GCE and GCEs modified with Bi2O3, MoO2, Bi2MoO6 and Au@Bi2MoO6 NTs, respectively. After the modification of Bi2O3, the current slightly decreased and the semi-circle clearly increased compared with the bare GCE, revealing the poor conductivity of Bi2O3. As expected, the incorporation of Mo into Bi2O3 enhanced the electron transfer rate of Bi2MoO6 NTs, indicating the good conductivity of Bi2MoO6 NTs, which was likely caused by the lattice occupancy of Mo inside the Bi2O3 crystal structure, and the decoration of Au NPs further improved the conductivity of Bi2MoO6 NTs, preferred for the fabrication of the electrochemical immunosensor.
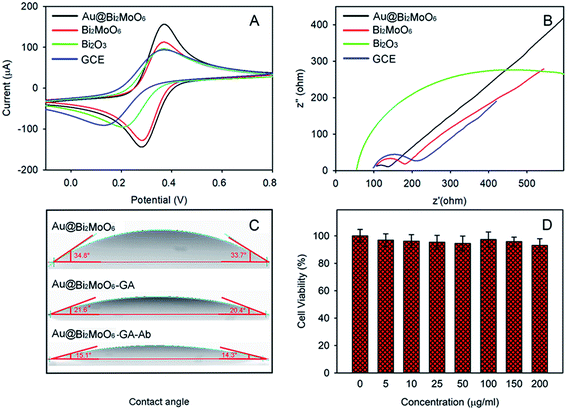 |
| Fig. 4 (A) CV and (B) EIS plots of bare GCE and GCEs modified with Bi2O3, Bi2MoO6 and Au@Bi2MoO6 NTs, respectively; (C) contact angles of Au@Bi2MoO6 NTs, Au@Bi2MoO6-GA and Au@Bi2MoO6-GA-Ab; (D) cellular activity of 4T1 treated with Au@Bi2MoO6 NTs at different concentrations. | |
Both contact angle and toxicity toward 4T1 cells were tested to explore the hydrophilicity and biocompatibility of Au@Bi2MoO6 NTs (Fig. 4C and D). As shown in Fig. 4C, the initial contact angle of Au@Bi2MoO6 NTs was 33.7–34.8°, showing favorable hydrophilicity. The modification of GA further reduced the contact angle to 20.4–21.6°, revealing better hydrophilicity, which is helpful for the immobilization of biomolecules, and the contact angle continued to decrease to 14.3–15.1° after the Au@Bi2MoO6 NT-modified GCE was incubated with anti-CEA, revealing the successful immobilization of anti-CEA on Au@Bi2MoO6 NTs.
Fig. 4D shows the cellular activity of the 4T1 cells treated with Au@Bi2MoO6 NTs at different concentrations. Clearly, Au@Bi2MoO6 NTs did not affect the viability of the 4T1 cells even when the concentration of Au@Bi2MoO6 NTs reached 200 ppm. These results indicate the high biocompatibility and low cytotoxicity of Au@Bi2MoO6 NTs.
3.2. Electrochemical behaviors of the Bi2MoO6@Au NT-based immunosensor
The CVs of the as-prepared Bi2MoO6@Au NT immunosensor were carried out in 5.0 mM [Fe(CN)6]3−/4− containing 0.1 M KCl (pH = 6.5) at different scan rates to study the electrochemical process. As demonstrated in Fig. 5A, the peak current (I) was dependent on the scan rate: both the anodic and cathodic peak currents increased with the increase in the scan rate from 10 to 400 mV s−1 and were directly proportional to the square root of the scan rate (v1/2), as shown in Fig. 5B, revealing that the electrochemical process of the as-prepared Bi2MoO6@Au NT-based immunosensor was controlled by diffusion.
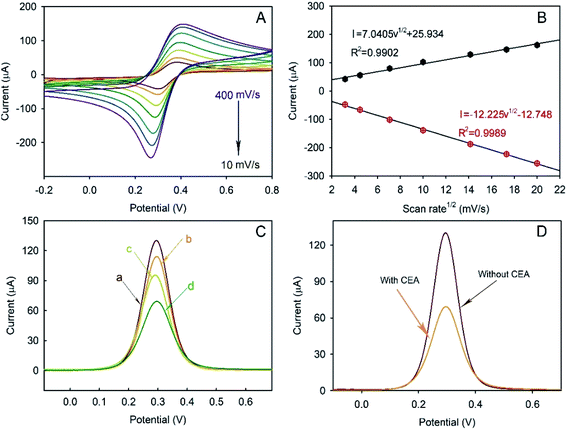 |
| Fig. 5 (A) CVs of the proposed immunosensor at different scan rates (from outer to inner: 400, 300, 200, 100, 50, 20 and 10 mV s−1); (B) the linear relationship between the square root of the scan rate and peak current; (C) voltammetric plots of GCEs modified with (a) Au@Bi2MoO6 NTs, (b) Au@Bi2MoO6-Ab, (c) Au@Bi2MoO6-Ab-BSA and (d) Au@Bi2MoO6-Ab-BSA-CEA; (D) DPV plots of Au@Bi2MoO6 NTs before and after the incubation of CEA. | |
DPV plots of differently modified GCEs were obtained to verify the successful layer-by-layer preparation (Fig. 5C). As can be seen, the Au@Bi2MoO6 TN-modified GCE (curve a) shows the highest current peak, which decreased after Ab was immobilized (curve b). BSA was used to cover the non-specific site on the electrode surface, which led to a further current decrease (curve c). The incubation of Au@Bi2MoO6-Ab-BSA with CEA continuously reduced the current, confirming the successful immobilization of CEA (curve d). The decreased in current can be attributed to the deposited proteins, which may prevent the interfacial electron transfer and reduce the active sites accessible to ferricyanide,40 verifying the successful layer-by-layer modification of biomolecules on the Au@Bi2MoO6 NT-modified electrode. Fig. 5D compares the response signal of the Au@Bi2MoO6 NT-based immunosensor before and after the immobilization of CEA. A sensitive response to CEA was observed using the as-prepared immunosensor.
3.3. Analytical performance, reproducibility and selectivity
As discussed above, a label-free immunosensor was designed based on Au@Bi2MoO6 NTs that directly converted the competitive immune response into measurable electrochemical signals. In order to achieve the best analytical performance of the Au@Bi2MoO6 NT-based immunosensor toward CEA detection, the experimental conditions were well optimized, which included the concentration of Au@Bi2MoO6 NTs, the pH of PBS, the concentration of anti-CEA and the antibody–antigen reaction (see the ESI S2 and Fig. S2†). Under the optimal conditions, the analytical performance with SWV of the Au@Bi2MoO6 NT-based immunosensor was examined toward CEA at different concentrations. As shown in Fig. 6A and B, the peak current was proportionally weakened as the concentration of CEA increased owing to the binding of more CEA molecules to anti-CEA on the electrode surface (Fig. 6A). A linear relationship between the peak current (I) and the logarithm of the CEA concentration (c) was achieved from 1 pg mL−1 to 1000 ng mL−1, where an equation of I = 96.791 − 6.0913
lg
c, was obtained with the correlation coefficient and limit of detection (S/N = 3) calculated to be 0.9976 and 0.3 pg mL−1, respectively (Fig. 6B). The concentration of CEA in healthy adults is usually below 2.5 ng mL−1 and higher than 20 ng mL−1 in bodies at risk of cancer. However, the concentration of CEA varies between cancers. For example, the low level of CEA in colon tissue was reported to be 2.5–5.0 μg L−1, but could greatly increase in patients with liver cancer.7 Even so, the detection range of the designed immunosensor was sufficient to support the CEA detection in samples of various cancers with good sensitivity.
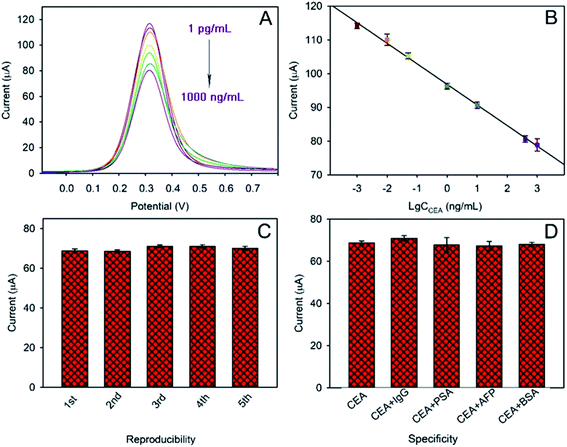 |
| Fig. 6 (A) Voltammetric responses and (B) the calibration curve of the Au@Bi2MoO6 NT-based immunosensor to 0.001, 0.01, 0.05, 1, 10, 400 and 1000 ng mL−1 CEA in the solution containing 5 mM [Fe(CN)6]3−/4− and 0.1 M KCl. (C) The reproducibility and (D) the specificity of the immunosensor. | |
In addition, Table 1 compares the analytical behaviors of the current Au@Bi2MoO6 NT-based immunosensor with some other existing immunosensors toward CEA with respect to detection range and limit. The minimum detection concentration of the designed immunosensor was about ten times smaller than that of numerous existing immunosensors toward CEA detection, including Mo and Bi-based sensors, and the detection limit was lower, revealing higher sensitivity. Upon the comparison, a conclusion could be made that the Au@Bi2MoO6 NT-based immunosensor showed more comparable advantages in both detection range and detection limit, providing a promising alternative for CEA detection.
Table 1 Analytical performances of different electrochemical immunosensors toward CEA detectiona
Immunosensor |
Linear range (ng mL−1) |
Detection limit (pg mL−1) |
Ref. |
CNTs: carbon nano-tube arrays; CSs: carbon spheres; 3D-G: 3D graphene; pDA: polydopamine; Con A: concanavalin A; NHs: nanohybrids; PB: Prussian blue; NB-ERGO: Nile blue A hybridized electrochemically reduced graphene oxide; MWCNTs: multiple-wall carbon nanotubes; CS: chitosan; CdS: cadmium sulfide. |
Cu-Au NP/CNT-CSs |
0.025–25 |
0.5 |
41 |
3D-G/pDA/Con A |
0.1–750 |
90 |
42 |
Co2(OH)2CO3-CeO2 |
0.002–75 |
0.51 |
43 |
Au-g-C3N4·NHs |
0.02–80 |
6.8 |
44 |
AuNP/TiO2-Gr/Thi/GCE |
0.1–10.0 |
10 |
45 |
Au-PB-Fe3O4 |
0.01–80 |
4 |
46 |
AuNP/NBeERGO/GCE |
0.001–40 |
0.45 |
7 |
AuNP/MWCNT/CS/GCE |
0.3–2.5 |
10 |
47 |
MoS2 nanosheets |
0.1–100 |
34 |
48 |
Mo-Mn3O4/MWCNT/CS |
0.1–125 |
4.9 |
8 |
I-BiOCl/CdS-10 |
0.01–40 |
2 |
49 |
Au@Bi2MoO6 NTs |
0.001–1000 |
0.3 |
This work |
Five different electrodes were separately modified with Au@Bi2MoO6 NTs to fabricate the immunosensor so as to evaluate the reproducibility. Fig. 6C shows the response of the five individually prepared immunosensors to 1.0 ng mL−1 of CEA under the same conditions. It was found that no obvious difference in the peak current was observed among the five immunosensors with a relative standard deviation of 2.16%, revealing the satisfactory reproducibility of the designed Au@Bi2MoO6 NT-based immunosensor for CEA detection.
Moreover, IgG, PSA, AFP and BSA were selected to examine the specificity of the Au@Bi2MoO6 NT-based immunosensor for CEA detection (Fig. 6D). In the presence of each interferent at 100 ng mL−1, the fabricated immunosensor did not show a significantly different response to 1 ng mL−1 CEA, and the detection behavior with the interfering substance present (100 fold higher) was not much different from that only with CEA, proving the satisfactory specificity of the present immunosensor, which is indicative of the preferable selectivity.
4. Conclusions
In this study, we synthesized a novel Bi2MoO6 nanohybrid with a tremella-like structure by doping Mo into Bi2O3. The combination of Bi with Mo led to the change of the morphology from sphere to tremella with a larger surface area and obvious enhancement in the conductivity due to the synergistic effects of Bi and Mo. After being decorated with Au NPs, both the conductivity and biocompatibility of the synthesized Au@Bi2MoO6 NTs were further improved, and thus used as an electrochemical immunosensing platform to detect CEA. The proposed immunosensor exhibited promising analytical performance toward CEA in a wide concentration range. The detection limit was comparable to that of other immunosensors for CEA detection. Moreover, both the specificity and the reproducibility of the immunosensor were qualified and superior to other materials in terms of biocompatibility, showing a great prospect in clinical CEA detection.
Conflicts of interest
There is no conflict of interest in this work.
Acknowledgements
This work was financially supported by the Shanghai Natural Science Foundation (19ZR1434800 and 19ZR1461900). The authors greatly appreciate these supports.
References
- P. A. Crosbie, R. Shah, Y. Summers, C. Dive and F. Blackhall, Prognostic and predictive biomarkers in early stage NSCLC: CTCs and serum/plasma markers, Transl. Lung Cancer Res., 2013, 2(5), 382–397 CAS.
- Y. W. Xu, Y. H. Peng, B. Chen, Z. Y. Wu, J. Y. Wu and J. H. Shen, et al., Autoantibodies as potential biomarkers for the early detection of esophageal squamous cell carcinoma, Am. J. Gastroenterol., 2014, 109(1), 36–45 CrossRef CAS PubMed.
- L. Zhao, M. Cheng, G. Liu, H. Lu, Y. Gao and X. Yan, et al., A fluorescent biosensor based on molybdenum disulfide nanosheets and protein aptamer for sensitive detection of carcinoembryonic antigen, Sens. Actuators, B, 2018, 273, 185–190 CrossRef CAS.
- L. A. Torre, R. L. Siegel, E. M. Ward and A. Jemal, Global Cancer Incidence and Mortality Rates and Trends–An Update, Cancer Epidemiol., Biomarkers Prev., 2016, 25(1), 16–27 CrossRef PubMed.
- A. B. Chinen, C. M. Guan, J. R. Ferrer, S. N. Barnaby, T. J. Merkel and C. A. Mirkin, Nanoparticle Probes for the Detection of Cancer Biomarkers, Cells, and Tissues by Fluorescence, Chem. Rev., 2015, 115(19), 10530–10574 CrossRef CAS PubMed.
- F. Y. Kong, B. Y. Xu, Y. Du, J. J. Xu and H. Y. Chen, A branched electrode based electrochemical platform: towards new label-free and reagentless simultaneous detection of two biomarkers, Chem. Commun., 2013, 49(11), 1052–1054 RSC.
- Y. S. Gao, X. F. Zhu, J. K. Xu, L. M. Lu, W. M. Wang and T. T. Yang, et al., Label-free electrochemical immunosensor based on Nile blue A-reduced graphene oxide nanocomposites for carcinoembryonic antigen detection, Anal. Biochem., 2016, 500, 80–87 CrossRef CAS PubMed.
- W.-T. Li, Y. Wang, F.-F. Deng, L.-L. Liu, H.-J. Nan and H. Li, Electrochemical Immunosensor for Carcinoembryonic Antigen Detection Based on Mo-Mn3O4/MWCNTs/Chits Nanocomposite Modified ITO Electrode, Nanotechnology, 2015, 10(8), 1550111 CAS.
- A. P. Yang, J. Liu, H. Y. Lei, Q. W. Zhang, L. Zhao and G. H. Yang, CA72-4 combined with CEA, CA125 and CAl9-9 improves the sensitivity for the early diagnosis of gastric cancer, Clin. Chim. Acta, 2014, 437, 183–186 CrossRef CAS PubMed.
- C. Aggarwal, N. J. Meropol, C. J. Punt, N. Iannotti, B. H. Saidman and K. D. Sabbath, et al., Relationship among circulating tumor cells, CEA and overall survival in patients with metastatic colorectal cancer, Ann. Oncol., 2013, 24(2), 420–428 CrossRef CAS PubMed.
- P. Yanez-Sedeno, S. Campuzano and J. M. Pingarron, Multiplexed Electrochemical Immunosensors for Clinical Biomarkers, Sensors, 2017, 17(5), 965 CrossRef PubMed.
- W. Shi and Z. Ma, A novel label-free amperometric immunosensor for carcinoembryonic antigen based on redox membrane, Biosens. Bioelectron., 2011, 26(6), 3068–3071 CrossRef CAS PubMed.
- H. Li, J. He, S. Li and A. P. Turner, Electrochemical immunosensor with N-doped graphene-modified electrode for label-free detection of the breast cancer biomarker CA 15-3, Biosens. Bioelectron., 2013, 43, 25–29 CrossRef CAS PubMed.
- L. Li, B. Liang, F. Li, J. Shi, M. Mascini and Q. Lang, et al., Co-immobilization of glucose oxidase and xylose dehydrogenase displayed whole cell on multiwalled carbon nanotube nanocomposite films modified electrode for simultaneous voltammetric detection of D-glucose and D-xylose, Biosens. Bioelectron., 2013, 42, 156–162 CrossRef CAS PubMed.
- L.-B. Zhang, S.-R. Yang, J.-Q. Wang, Y. Xu and X.-Z. Kong, A facile preparation and electrochemical properties of nickel based compound–graphene sheet composites for supercapacitors, Chin. Chem. Lett., 2015, 26(5), 522–528 CrossRef CAS.
- Z. Qiu, J. Shu and D. Tang, Bioresponsive Release System for Visual Fluorescence Detection
of Carcinoembryonic Antigen from Mesoporous Silica Nanocontainers Mediated Optical Color on Quantum Dot-Enzyme-Impregnated Paper, Anal. Chem., 2017, 89(9), 5152–5160 CrossRef CAS PubMed.
- G. Nie, Y. Tang, B. Zhang, Y. Wang and Q. Guo, Label-free photoelectrochemical immunosensing platform for detection of carcinoembryonic antigen through photoactive conducting poly(5-formylindole) nanocomposite, Biosens. Bioelectron., 2018, 116, 60–66 CrossRef CAS PubMed.
- S. Kumar, M. Umar, A. Saifi, S. Kumar, S. Augustine and S. Srivastava, et al., Electrochemical paper based cancer biosensor using iron oxide nanoparticles decorated PEDOT:PSS, Anal. Chim. Acta, 2019, 1056, 135–145 CrossRef CAS PubMed.
- X. Zhang, N. Bao, X. Luo and S. N. Ding, Patchy gold coated Fe3O4 nanospheres with enhanced catalytic activity applied for paper-based bipolar electrode-electrochemiluminescence aptasensors, Biosens. Bioelectron., 2018, 114, 44–51 CrossRef CAS PubMed.
- P. D. Tam and C. X. Thang, Label-free electrochemical immunosensor based on cerium oxide nanowires for Vibrio cholerae O1 detection, Mater. Sci. Eng., C, 2016, 58, 953–959 CrossRef CAS PubMed.
- F. Li, Y. Li, J. Feng, Y. Dong, P. Wang and L. Chen, et al., Ultrasensitive amperometric immunosensor for PSA detection based on Cu2O@CeO2-Au nanocomposites as integrated triple signal amplification strategy, Biosens. Bioelectron., 2017, 87, 630–637 CrossRef CAS PubMed.
- M. Li, P. Wang, F. Li, Q. Chu, Y. Li and Y. Dong, An ultrasensitive sandwich-type electrochemical immunosensor based on the signal amplification strategy of mesoporous core-shell Pd@Pt nanoparticles/amino group functionalized graphene nanocomposite, Biosens. Bioelectron., 2017, 87, 752–759 CrossRef CAS PubMed.
- R. Jain and R. Sharma, Novel bismuth/multi-walled carbon nanotubes-based electrochemical sensor for the determination of neuroprotective drug cilostazol, J. Appl. Electrochem., 2012, 42(5), 341–348 CrossRef CAS.
- Y. Chen, P.-X. Yuan, A.-J. Wang, X. Luo, Y. Xue and L. Zhang, A novel electrochemical immunosensor for highly sensitive detection of prostate-specific antigen using 3D open-structured PtCu nanoframes for signal amplification, Biosens. Bioelelctron., 2019, 126, 187–192 CrossRef CAS PubMed.
- Q. Han, R. Wang, B. Xing, H. Chi, D. Wu and Q. Wei, Label-free photoelectrochemical aptasensor for tetracycline detection based on cerium doped CdS sensitized BiYWO6, Biosens. Bioelelctron., 2018, 106, 7–13 CrossRef CAS PubMed.
- Q. Han, H. Chi, H. Wang, D. Wu and Q. Wei, Using PbS-Au heterodimers as signal quencher for the sensitive photoelectrochemical immunoassay of amyloid β-protein, Anal. Chim. Acta, 2019, 1092, 85–92 CrossRef CAS PubMed.
- J. Feng, Y. Li, Z. Gao, H. Lv, X. Zhang and Y. Dong, et al., A competitive-type photoelectrochemical immunosensor for aflatoxin B1 detection based on flower-like WO3 as matrix and Ag2 S-enhanced BiVO 4 for signal amplification, Sens. Actuators, B, 2018, 270, 104–111 CrossRef CAS.
- Y. Qiu, H. Fan, X. Chang, H. Dang, Q. Luo and Z. Cheng, Novel ultrathin Bi2O3 nanowires for supercapacitor electrode materials with high performance, Appl. Surf. Sci., 2018, 434, 16–20 CrossRef CAS.
- J. Hu, G. Xu, J. Wang, J. Lv, X. Zhang and T. Xie, et al., Photocatalytic property of a Bi2O3 nanoparticle modified BiOCl composite with a nanolayered hierarchical structure synthesized by in situ reactions, Dalton Trans., 2015, 44(12), 5386–5395 RSC.
- S. N. Ding, D. Shan, H.-G. Xue and S. C. J. Bioelectrochemistry, A promising biosensing-platform based on bismuth oxide polycrystalline-modified electrode: characterization and its application in development of amperometric glucose sensor, Bioelectrochemistry, 2010, 79(2), 218–222 CrossRef CAS PubMed.
- P. R. Solanki, J. Singh, B. Rupavali, S. Tiwari and B. D. Malhotra, Bismuth oxide nanorods based immunosensor for mycotoxin detection, Mater. Sci. Eng., C, 2016, 70, 564–571 CrossRef PubMed.
- Z. Gao, Y. Li, C. Zhang, S. Zhang, F. Li and P. Wang, et al., Label-free electrochemical immunosensor for insulin detection by high-efficiency synergy strategy of Pd NPs@3D MoSx towards H2O2, Biosens. Bioelectron., 2019, 126, 108–114 CrossRef CAS PubMed.
- B. Zhang, Y. Xue, A. Jiang, Z. Xue, Z. Li and J. Hao, Ionic Liquid as Reaction Medium for Synthesis of Hierarchically Structured One-Dimensional MoO2 for Efficient Hydrogen Evolution, ACS Appl. Mater. Interfaces, 2017, 9(8), 7217–7223 CrossRef CAS PubMed.
- F. Li, Y. Li, J. Feng, Z. Gao, H. Lv and X. Ren, et al., Facile synthesis of MoS2@Cu2O-Pt nanohybrid as enzyme-mimetic label for the detection of the Hepatitis B surface antigen, Biosens. Bioelectron., 2018, 100, 512–518 CrossRef CAS PubMed.
- Z. Gao, Y. Li, X. Zhang, J. Feng, L. Kong and P. Wang, et al., Ultrasensitive electrochemical immunosensor for quantitative detection of HBeAg using Au@Pd/MoS2@MWCNTs nanocomposite as enzyme-mimetic labels, Biosens. Bioelectron., 2018, 102, 189–195 CrossRef CAS PubMed.
- Z. Li, Y. Hu, Z. Miao, H. Xu, C. Li and Y. Zhao, et al., Dual-Stimuli Responsive Bismuth Nanoraspberries for Multimodal Imaging and Combined Cancer Therapy, Nano Lett., 2018, 18(11), 6778–6788 CrossRef CAS PubMed.
- W. Liu, X. Li, W. Li, Q. Zhang, H. Bai and J. Li, et al., Highly stable molybdenum dioxide nanoparticles with strong plasmon resonance are promising in photothermal cancer therapy, Biomaterials, 2018, 163, 43–54 CrossRef CAS PubMed.
- Q. Yan, L. Cao, H. Dong, Z. Tan, Y. Hu and Q. Liu, et al., Label-free immunosensors based on a novel multi-amplification signal strategy of TiO2-NGO/Au@Pd hetero-nanostructures, Biosens. Bioelectron., 2019, 127, 174–180 CrossRef CAS PubMed.
- J. C. Jung, H. Lee, H. Kim, Y.-M. Chung, T. J. Kim and S. J. Lee, et al., Effect of Oxygen Capacity and Oxygen Mobility of Pure Bismuth Molybdate and Multicomponent Bismuth Molybdate on their Catalytic Performance in the Oxidative Dehydrogenation of n-Butene to 1,3-Butadiene, Catal. Lett., 2008, 124(3–4), 262–267 CrossRef CAS.
- B. Kavosi, A. Salimi, R. Hallaj and F. Moradi, Ultrasensitive electrochemical immunosensor for PSA biomarker detection in prostate cancer cells using gold nanoparticles/PAMAM dendrimer loaded with enzyme linked aptamer as integrated triple signal amplification strategy, Biosens. Bioelectron., 2015, 74, 915–923 CrossRef CAS PubMed.
- D. T. Tran, V. H. Hoa, L. H. Tuan, N. H. Kim and J. H. Lee, Cu-Au nanocrystals functionalized carbon nanotube arrays vertically grown on carbon spheres for highly sensitive detecting cancer biomarker, Biosens. Bioelectron., 2018, 119, 134–140 CrossRef CAS PubMed.
- J. Liu, J. Wang, T. Wang, D. Li, F. Xi and J. Wang, et al., Three-dimensional electrochemical immunosensor for sensitive detection of carcinoembryonic antigen based on monolithic and macroporous graphene foam, Biosens. Bioelectron., 2015, 65, 281–286 CrossRef CAS PubMed.
- N. Alizadeh, A. Salimi and R. Hallaj, Mimicking peroxidase activity of Co2(OH)2CO3-CeO2 nanocomposite for smartphone based detection of tumor marker using paper-based microfluidic immunodevice, Talanta, 2018, 189, 100–110 CrossRef CAS PubMed.
- L. Chen, X. Zeng, P. Si, Y. Chen, Y. Chi and D. H. Kim, et al., Gold nanoparticle-graphite-like C3N4 nanosheet nanohybrids used for electrochemiluminescent immunosensor, Anal. Chem., 2014, 86(9), 4188–4195 CrossRef CAS PubMed.
- K. Huang, Z. Wu, Y. Wu and Y. Liu, Electrochemical immunoassay of carcinoembryonic antigen based on TiO2–graphene/thionine/gold nanoparticles composite, Can. J. Chem., 2012, 90(7), 608–615 CrossRef CAS.
- Y. Zhuo, P. X. Yuan, R. Yuan, Y. Q. Chai and C. L. Hong, Bienzyme functionalized three-layer composite magnetic nanoparticles for electrochemical immunosensors, Biomaterials, 2009, 30(12), 2284–2290 CrossRef CAS PubMed.
- K. J. Huang, D. J. Niu, W. Z. Xie and W. Wang, A disposable electrochemical immunosensor for carcinoembryonic antigen based on nano-Au/multi-walled carbon nanotubes-chitosans nanocomposite film modified glassy carbon electrode, Anal. Chim. Acta, 2010, 659(1–2), 102–108 CrossRef CAS PubMed.
- L. Zhao, M. Cheng, G. Liu, H. Lu, Y. Gao and X. Yan, et al., A fluorescent biosensor based on molybdenum disulfide nanosheets and protein aptamer for sensitive detection of carcinoembryonic antigen, Sens. Actuators, B, 2018, 273, 185–190 CrossRef CAS.
- H. Wang, B. Zhang, J. Xi, F. Zhao and B. Zeng, Z-scheme I-BiOCl/CdS with abundant oxygen vacancies as highly effective cathodic material for photocathodic immunoassay, Biosens. Bioelectron., 2019, 141, 111443 CrossRef CAS PubMed.
Footnote |
† Electronic supplementary information (ESI) available. See DOI: 10.1039/d0ra01922d |
|
This journal is © The Royal Society of Chemistry 2020 |