DOI:
10.1039/D0RA01068E
(Review Article)
RSC Adv., 2020,
10, 15328-15345
Progress in the functional modification of graphene/graphene oxide: a review
Received
4th February 2020
, Accepted 24th March 2020
First published on 17th April 2020
Abstract
Graphene and graphene oxide have attracted tremendous interest over the past decade due to their unique and excellent electronic, optical, mechanical, and chemical properties. This review focuses on the functional modification of graphene and graphene oxide. First, the basic structure, preparation methods and properties of graphene and graphene oxide are briefly described. Subsequently, the methods for the reduction of graphene oxide are introduced. Next, the functionalization of graphene and graphene oxide is mainly divided into covalent binding modification, non-covalent binding modification and elemental doping. Then, the properties and application prospects of the modified products are summarized. Finally, the current challenges and future research directions are presented in terms of surface functional modification for graphene and graphene oxide.
1. Introduction
Graphene is a carbon material,1 and in 1985, Robert Curl et al.2 prepared a C60 product. However, four years later, Krätschmer3 confirmed the cage structure of C60-fullerene. In 1991, Nippon Electric Company (NEC) Ltd. first reported carbon nanotubes and expanded the carbon material family.4 In 2004, Novoselov et al.5 successfully separated graphene from the monolithic state using a micro-computer peeling method, which challenged the scientific understanding of two-dimensional crystals. The structure of graphene is shown in Fig. 1, which is composed of a layer of independent sp2 hybrid carbon atoms. It is a two-dimensional carbonaceous material with a hexagonal honeycomb crystal structure. To now, graphene is the thinnest and strongest nanomaterial, with a sheet thickness of 0.34 nm.6 Each carbon atom in graphene is bonded to three adjacent carbon atoms through a σ bond. The remaining p electrons most likely form a π bond with the surrounding atoms due to their failure to form a bond, and the bonding direction is perpendicular to the graphene plane. The structure of graphene is very stable, and its C–C bond length is only 0.142 nm.7 The connection between each carbon atom of graphene is very strong. When an external force is applied to graphene, the atomic surface inside it is deformed and further bent to offset the external force. Thus, there is no rearrangement and misalignment between the carbon atoms, maintaining a consistently stable structure.8 When the electrons of graphene move in the internal orbit, there is no scattering phenomenon due to the interference of foreign atoms or lattice defects.9,10 This unique lattice structure gives graphene various excellent properties. Nowadays, there are numerous methods for the preparation of graphene, but the main preparation methods include mechanical stripping, liquid phase stripping, chemical vapor deposition, epitaxial growth and redox methods.11 Recently, significant research has been conducted on graphene quantum dots,12–14 and carbon doped with other elements, molecules or organic materials.15–18
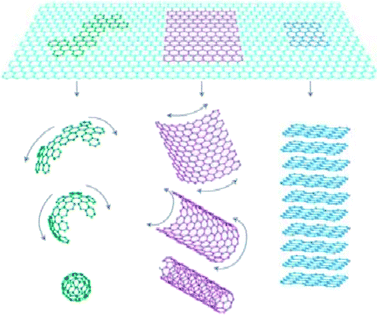 |
| Fig. 1 Carbon allotropes: graphene to fullerene, nanotubes and graphite. This figure has been reproduced from ref. 19 with permission from Springer Nature, Copyright 2007. | |
Compared with graphene (G), graphene oxide (GO) has the advantages of low production cost, large-scale production, and easy processing. It is often used as a precursor for the preparation of reduced graphene oxide (RGO).20 In recent years, with the further study of GO, scientists have found that it also has excellent properties with rich active oxygen-containing functional groups.21 These oxygen-containing groups or reduced doping elements can be used as catalytic active centers for covalent/non-covalent modification design according to the requirements of specific application fields. In addition, the presence of oxygen-containing groups also broadens the interlayer gap of graphene oxide. It can be functionalized by small molecules or polymer intercalations. At present, great progress has been achieved in the functionalization of graphene oxide. It has been applied in the fields of desalination,22 drug delivery,23 oil–water separation,24 immobilization catalysis,25 solar cells,26 energy storage,27 healthcare,28,29 etc.
However, the single component graphene material has certain limitations, such as weak electrochemical activity, easy agglomeration and difficult processing, which greatly limit the application of graphene. Therefore, functional modification of graphene and graphene oxide is crucial to expanding their application. The functionalization of graphene and graphene oxide is based on the further modification of their intrinsic structure. Herein, we introduce the functional modification methods based on the intrinsic structural chemical bonds and functional groups of graphene and graphene oxide. Firstly, we introduce the basic structure and properties of graphene and graphene oxide, and then their functionalization based on their surface structure features, which is divided into three types, functional modification of covalent bond bonding, functional modification of non-covalent bond action and element doping modification. Subsequently, we categorize and systematically summarize the reaction process and reaction conditions of typical reaction types and their research methods. Finally, the future of surface functionalization of graphene and graphene oxide is presented.
2. Structure and properties of graphene/graphene oxide
Graphene has a planar hexagonal lattice structure. There are 4 valence electrons per carbon atom, including 3 electrons (2s electron, 2px electron and 2py electron), forming plane sp2 hybrid orbitals. The remaining orbital electron forms a large π bond, and this electron can move freely in the plane. Graphene and graphene oxide show excellent electrical, mechanical, and thermal properties due to their unique structural and morphological features.30–36 They exhibit the Hall effect, tunneling effect, bipolar electric field effect and high thermal conductivity. The electrons are not easily scattered when they are transported in G, and the maximum electron mobility at room temperature can reach 2 × 105 cm2 (V s)−1,24 and thus the ideal conductivity of G is over 1 × 106 S cm−1.24 The Young's modulus of G can reach up to 1100 GPa,25 its transmittance is about 97.9% for visible light,25 and its specific surface area can be as high as 2630 m2 g−1.26 As a two-dimensional carbon material, graphene oxide has a single atomic layer, and the size of its sheets is polydisperse. Compared with graphene, there are many oxygen-containing functional groups on the graphene oxide sheet layer, thus the structure of graphene oxide is more complicated, and its properties depend on its structure. Lerf and Klinowski37,38 put up the structural model of graphene oxide, called the L–K model. It indicates that the hydroxyl and epoxy groups are randomly distributed on the graphene oxide single layer, while the carboxyl and carbonyl groups are introduced at the edge of the single layer. Graphene oxide has an unoxidized benzene ring region and an oxidized aliphatic six-membered ring region, and the relative size of these two regions depends on the degree of oxidation and random distribution on graphene oxide. However, the L–K structural model is based on certain conditions, ignoring the influence of raw graphene, oxidant and oxidation methods. Erickson et al.39 studied graphene oxide nano-plates via scanning electron microscopy (SEM), and found that graphene oxide not only has an oxidized region with high disorder and unoxidized graphene region, but also has hole defects due to overoxidation and lamellar peeling. Accordingly, in recent years, other models have been proposed, such as the dynamic structure model (DSM)40,41 and binary structure model.42 Extensive research has been conducted on the preparation and properties of G and GO by many scientists, and it has been found that the obvious difference between graphene and graphene oxide is the addition of oxygen atoms bound with some carbons, as shown in Fig. 2. As a result, graphene is hydrophobic in nature, whereas graphene oxide is hydrophilic and easily dispersed in water. In addition, graphene oxide contains both aromatic (sp2) and aliphatic (sp3) domains, which lead to an increase in the type of interactions that can occur on its surface. Graphene oxide can be reduced to graphene by a reducing agent. However, the produced graphene is not suitable for electronic applications and mechanical reinforcement of polymers due to the structural defects created during the synthesis of graphene oxide. Nevertheless, this is the preferable route for the large-scale modification of the surface properties of graphene materials by functionalization.
 |
| Fig. 2 Oxidation of graphene sheet to form graphene oxide. This figure has been reproduced from ref. 43 with permission from Chem. Rev., Copyright 2016. | |
3. Preparation of graphene oxide
The preparation of graphene oxide is generally carried out via two steps of oxidant intercalation oxidation and sheet peeling, as shown in Fig. 3. 150 years ago, Brodie44 prepared graphite oxide for the first time, but it was not noticed at that time. With the development of the graphene oxide, the main methods for the synthesis of graphene oxide are presented in Table 1. The schematic synthesis of graphene oxide by the modified Hummers' method is shown in Fig. 4.
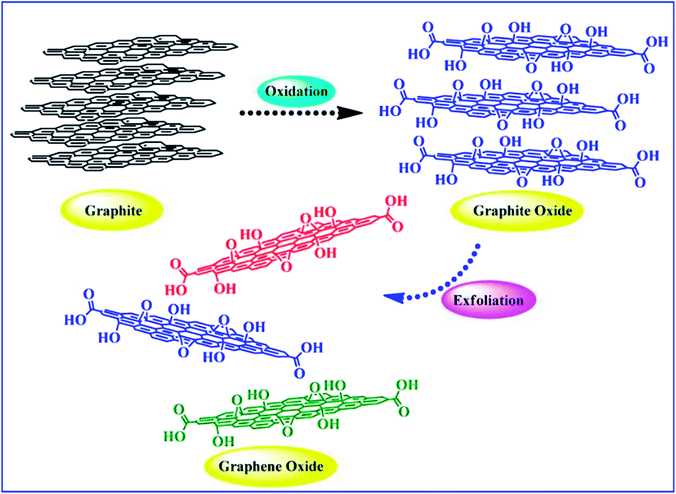 |
| Fig. 3 Preparation of graphene oxide. | |
Table 1 The main methods of synthesize graphene oxide
Scientists |
Reagent |
Reaction time |
Reaction temperature |
Characteristics |
Brodie44 |
KClO3, HNO3 |
3–4 h |
60 °C |
The first method |
Staudenmaier45 |
KClO3, HNO3, H2SO4 |
96 h |
RT |
|
Hummers46 |
HNO3 |
20 h |
RT |
Only using HNO3 |
David47 |
KMnO4, NaNO3, H2SO4 |
<2 h |
35 °C |
Reaction time is short |
Eigler48 |
KMnO4, NaNO3, H2SO4 |
16 h |
10 °C |
High quality RGO |
Peng49 |
K2FeO4, HSO4 |
1 h |
RT |
No heavy metal manganese pollution |
Marcano50 |
H2SO4, H3PO4, KMnO4 |
12 h |
50 °C |
Low toxicity |
Panwar51 |
H2SO4, H3PO4, KMnO4, HNO3 |
3 h |
50 °C |
High yield |
Shen52 |
Benzoyl peroxide |
10 min |
110 °C |
No liquid |
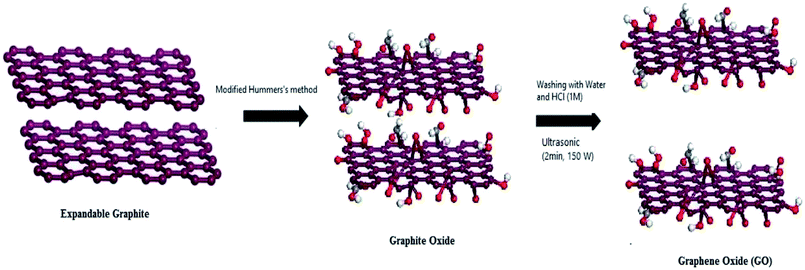 |
| Fig. 4 Schematic synthesis of graphene oxide by the modified Hummers' method. This figure has been reproduced from ref. 53 with permission from Chem. Mater., Copyright 1999. | |
The different methods have advantages and disadvantages. In the early stages, nitric acid and KClO3 were used, but harmful gases such as ClO2, NO2 and N2O4 were generated during the reaction, which took a long time.52,54 The Hummers' method was used widely; however, this method produces NO2, N2O4 and heavy metal pollution. Also, the products contained Na+ and NO3− ions, which were difficult to remove. Besides, the oxidizing agent (NaNO3/KMnO4) had strong oxidizing properties in concentrated sulfuric acid, and there was a high risk of explosion when Mn2O7 contacted with organic matter at 55 °C.55 Subsequently, Marcano50 used the less corrosive phosphoric acid to produce graphene oxide in situ. This method does not involve the release of intense exothermic or toxic gases, and the prepared graphene oxide has a higher degree of oxidation and structural regularity, but requires a large amount of KMnO4 and concentrated sulfuric acid, thus increasing the cost of processing raw materials and the treatment of waste liquids. The preparation of graphene oxide using the K2FeO4/H2SO4 oxidation system49 at room temperature for 1 h is a safe and efficient method. Using benzoyl peroxide52 as an oxidant at 110 °C, graphene oxide can also be prepared by reaction for 10 min. Although this method is highly efficient, benzoyl peroxide itself is extremely unstable and there may be a risk of explosion when the reaction temperature is high. Besides the effect of oxidants on graphene oxide, the graphite raw materials and the reaction methods also affect the properties of graphene oxide, where relatively few hydroxyl and carboxyl groups are obtained on graphene oxide prepared from highly crystalline graphite powder.56 During the low-temperature and mid-temperature stages of graphene oxide preparation, a modified Hummers' method with the help of auxiliary ultrasound equipment was used, and the layer spacing of graphene oxide became larger.57 However, there were many holes in the graphene oxide due to excessive oxidation. When the reaction temperature was over 50 °C, the graphene oxide became unstable. If this reaction occurred at a relatively low temperature, the hole defects of graphene oxide would diminish significantly.58 Currently, the feasibility of the industrial preparation of graphene with graphene oxide as the precursor is high, but the existing graphene oxide preparation methods generally have technical problems such as complicated purification steps and many defects. Therefore, compared with mechanically exfoliated high-quality graphene, the prepared reduced graphene oxide is inferior in structure and properties.
The proper functionalization of graphene and graphene oxide prevents agglomeration during the reduction of graphene and graphene oxide,59 and preserves their inherent properties. The functionalization of graphene and graphene oxide results in new functions, and subsequently can possess excellent mechanical properties, electrical properties, thermal properties, etc. Currently, the methods for the functionalization of graphene and graphene oxide mainly include covalent bond functionalization, non-covalent bond functionalization, and other atomic doping functionalization.60
4. Reduction of graphene oxide
4.1 Sodium borohydride reducing agent
Si et al.61 prepared graphene from graphene oxide using the sodium borohydride reducing agent and then sulfonation with the aryl diazonium salt of sulfanilic acid. The light sulfonated graphene was easily dispersed in water at a suitable concentration (2 mg mL−1) in the pH range of 3–10. By observing the AFM images shown in Fig. 5, they found that the lateral dimension of graphene oxide was several micrometers and its thickness was 1 nm, but there were some differences with the reaction of chemically reduced GO (RGO), where the lateral dimension varied from several hundred nanometers to several micrometers, and the thickness was about 1.2 nm. During the experiment, excessive ultrasonic treatment may cause some small hole-like defects to be introduced in graphene oxide, causing the single graphene sheet to be folded over on one edge with isolated small fragments of graphene on its surface (Fig. 5(d)), which was the reason why the AFM images showed an increase in RGO thickness to 10 μm.
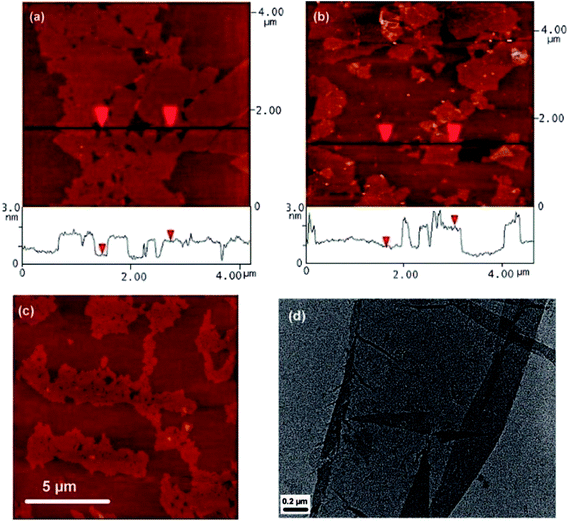 |
| Fig. 5 Images of isolated graphene oxide and graphene sheets. (a) AFM image of graphene oxide sheets on freshly cleaved mica, the height difference between two arrows is 1 nm, indicating a single graphene oxide sheet; (b) AFM image of water-soluble graphene on freshly cleaved mica; the height difference between two arrows is 1.2 nm; (c) AFM image of large graphene oxide sheets on mica, small holes in the sheets are caused by over-exposure to sonicaion; (d) TEM image of a partially folded water-soluble graphene sheet. This figure has been reproduced from ref. 61 with permission from Nano Lett., Copyright 2008. | |
Choi et al.62 reported a simple method for the fabrication of multifunctional fibers with mechanically strong RGO cores and highly conductive chemical vapor deposition (CVD) graphene shells (rGO@Gr fibers), which showed outstanding electrical conductivity as high as 137 S cm−1 and a failure strain value of 21%. These values are believed to be the highest values among polymer-free graphene fibers. We also demonstrated the use of the rGO@Gr fibers in high power density supercapacitors with enhanced mechanical stability and durability, which enables their practical applications in various smart wearable devices in the future. The main method involved the preparation of the CVD graphene film, GO fiber fabrication, fabrication of graphene–GO fiber (a reduction solution (HI
:
AcOH = 2
:
5 v/v)) and testing the thermal conductivity.
4.2 Alcohol reducing agent reduction method
The reduction of GO with an alcohol is a relatively mild reduction method because this treatment method does not cause severe damage to the edge morphology of GO, and highly conductive RGO can be prepared.
Su et al.63 reduced GO with ethanol vapor at high temperature to obtain highly conductive RGO. Experiments showed that the impedance of GO was 188–418 kΩ μm−2, and after ethanol vapor reduction at 900 °C, the impedance was reduced by 43 kΩ μm−2. This result was similar to the result from reducing GO at 1100 °C under vacuum (40–100 kΩ μm−2). Besides, they also studied the reduction of GO with H2 at high temperature. The results indicated that ethanol vapor was more effective at reducing GO at the same temperature.
4.3 Phenolic reduction method
In addition to alcohol reducing agents, some phenolic reducing agents are also used to reduce GO. Wang et al.64 studied the peeling of GO for 20 h into a GNS sheet by hydroquinone upon refluxing GO with hydroquinone. The results showed that the product RGO had a low oxygen content and maintained an ordered crystal structure, but agglomeration occurred within only a few hours in water. It was also found that the thermal stability of the product was worse than that of graphite powder.
4.4 Thermal exfoliation and reduction
Thermally reduced graphene oxide (TRG) can be produced via the rapid heating of dry GO under inert gas and high temperature. The properties of thermal reduction products are directly related to the heating rate, reduction temperature and time. Too fast heating rate and too long reduction time will greatly expand the volume of the product, and the specific surface area is much lower than the theoretical value of graphene. Hyunwoo Kim65 reviewed that in top-down processes, graphene or modified graphene sheets are produced by separation/exfoliation of graphite or graphite derivatives. In general, these methods are suitable for large-scale production for polymer composite applications. For the process of TRG, heating GO in an inert environment for 30 s at 1000 °C causes the reduction and flaking of GO, thereby producing TRG sheets. Delamination occurs when the pressure generated by the gas (CO2) due to the decomposition of the epoxy and hydroxyl groups of GO exceeds the van der Waals force holding the graphene oxide sheets together.
Fadil66 also reported a polymer/GO material, where its preparation involved aqueous mini-emulsion copolymerization of St and nBA in the presence of nano-dimensional GO (small and large sheets) and the conventional surfactant SDS. The obtained latex comprising polymer particles armoured with GO sheets were used directly for film formation at ambient temperature.
4.5 Other methods
There as other reduction methods such as sulfur-containing compound reducing agent reduction method, SO2 reduction, ultraviolet radiation reduction method, and electrochemical reduction method. Some researchers prepared RGO using sulfur-containing compound reducing agents, and found that sulfur-containing compounds have good reducing properties. The oxygen content in the reduced product is relatively low and stably dispersed in solution. When SO2 gas was used as a reducing agent for reducing GO, the oxygen content of the product RGO obtained by this method was similar to that using hydrazine as the reduction agent, and the sulfur-containing compound could promote the dispersion of the product in aqueous solution.67 It was also found that the product RGO was a single layer having a thickness of only 0.87 nm, but the surface had significant wrinkles. When the number of layers was below 10 layers, a large area of wrinkles appears in GO,68 as shown in Fig. 6.
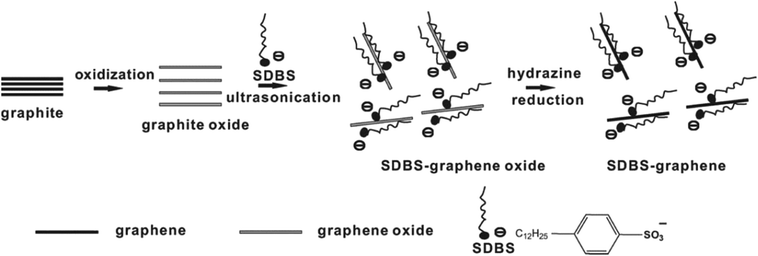 |
| Fig. 6 Schematic of solution-processible SDBS–graphene synthesis by in situ reduction with SDBS as the stabilizing agent. This figure has been reproduced from ref. 53 with permission from Nano Lett., Copyright 2007. | |
Ultraviolet radiation reduction and electrochemical methods are also environmentally friendly methods for the preparation of GNS, and they have a great potential in the large-scale preparation of GNS. Williams et al.69 conducted an in-depth study on the ultraviolet (UV) irradiation reduction method, and a schematic of the TiO2–graphene composite and its response under UV-excitation is shown in Fig. 7.
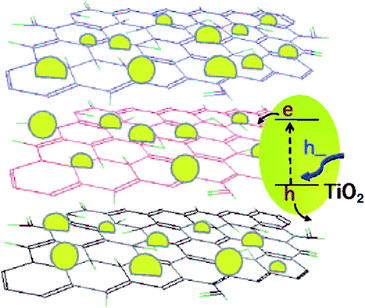 |
| Fig. 7 TiO2–graphene composite and its response under UV-excitation. This figure has been reproduced from ref. 69 with permission from ACS Nano, Copyright 2008. | |
They obtained stable RGO by reducing the GO suspension by UV irradiation. Compared with the use of a photocatalyst or chemical reducing agent, this method is not only simple and easy, but also inexpensive, and it provides a new possible way for the mass preparation of GNS.
5. Functionalized graphene and graphene oxide
The proper functionalization of graphene and graphene oxide prevents their agglomeration during the reduction process,59 and preserves their inherent properties. The functional modification of graphene and graphene oxide not only maintains their excellent characteristics, but also introduces new functional groups to give them new characteristics. Also, different functional groups give different characteristics. Presently, the methods for the functionalization of graphene and graphene oxide mainly include covalently functionalization, non-covalent functionalization, and elemental doping.70
5.1 Covalent functionalization
Covalent bond functionalization of graphene involves combining graphene with newly introduced groups in the form of covalent bonds to improve and enhance its performance. The oxygen-containing groups on the surface of graphene oxide makes covalent bond functionalization easier than that on graphene. The surface of graphene oxide contains a large amount of hydroxyl groups, carboxyl groups, and epoxy groups. These groups can be used for common chemical reactions such as isocyanation, carboxylic acylation, epoxy ring opening, diazotization, and addition.71 The covalent bond functional modification of graphene and graphene oxide is illustrated below according to the functional group. Covalent bond modification increases the processability and brings new functions. There are multiple strategies to covalently functionalize GO. For example, Man et al.72 using polystyrene particles “armoured” with nanosized graphene oxide (GO) sheets, which were prepared by aqueous mini-emulsion polymerization of styrene, exploiting the amphiphilic properties of GO in the absence of conventional surfactants. The nanoscale GO sheets were prepared from graphite nanofibers with a diameter of approximately 100 nm based on a novel procedure, thus effectively ensuring the absence of larger sheets.
5.1.1 Carbon skeleton functionalization. The functional modification of the carbon skeleton is mainly carried out using the C
C bond in the aromatic ring of graphene or graphene oxide. The graphene oxide diazotization reaction and Diels–Alder reaction have been reported.73–75Zhong et al.76 used solution-phase graphene as a raw material dispersed in 2% sodium cholate (as surfactants) aqueous solution, and stirred it with 4-propargyloxydiazobenzenetetrafluoroborate at 45 °C for about 8 h, to obtain 4-propargyloxyphenyl graphene (GC
CH). Then, a click chemistry reaction with the azido polyethylene glycol carboxylic acid (as shown in Fig. 8) was carried out to realize an addition reaction to the graphene carbon skeleton, thereby further functionalizing the graphene. This method is flexible and convenient, and can be used to prepare graphene composite materials and biosensors by changing the functionalized modified groups connecting graphene.
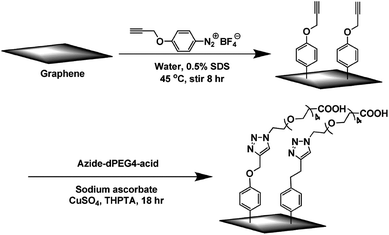 |
| Fig. 8 Diazonium reaction and subsequent click chemistry functionalization of graphene sheets. This figure has been reproduced from ref. 77 with permission from Chem. Mater., Copyright 2011. | |
This, it can be concluded that the basic process is a diazonium salt or a diazo compound formed by diazotization of an aromatic amine-containing substance having a reactive functional group, and an electron is removed to form a free radical after deaeration.77 Then, a double bond addition reaction with C
C is carried out to form a new C–C single bond, which is linked to the derivative of benzene having a reactive functional group by a sigma bond, distributed on the surface, and then further, graphene with is reactive functional groups undergo functional modification with graphene oxide.
5.1.2 Hydroxy functionalization. Graphene oxide contains a large amount of reactive hydroxyl groups on its sheet layer, where hydroxyl-based functional modification generally involves hydroxy-reaction of amide or isocyanate and graphene oxide to produce esters, and then further functional modification using different groups.78 Yang et al.79 prepared azidolated graphene oxide via esterification and substitution of the hydroxyl groups on the surface of graphene oxide. Firstly, graphene oxide and 2-bromoisobutyryl bromide were stirred at room temperature for 48 h. After esterification, the product was dispersed in dimethylformamide, and NaN3 was added at room temperature for 24 h to obtain azido-modified graphene oxide (GO–N3). Finally, alkyne-functionalized polystyrene (HC
C–PS) was used to graft polystyrene onto the surface of the oxide by esterification to obtain graphene-based polystyrene. The modified graphene oxide had good solubility in polar solvents such as tetrahydrofuran, dimethylformamide and chloroform, and the distance between GO layers could be controlled by the length of PS. This method can be extended to the functionalization of other graphite polymer composites. The preparation route and reaction conditions for the target hydroxy-based functionalization of graphene oxide is shown in Fig. 9.
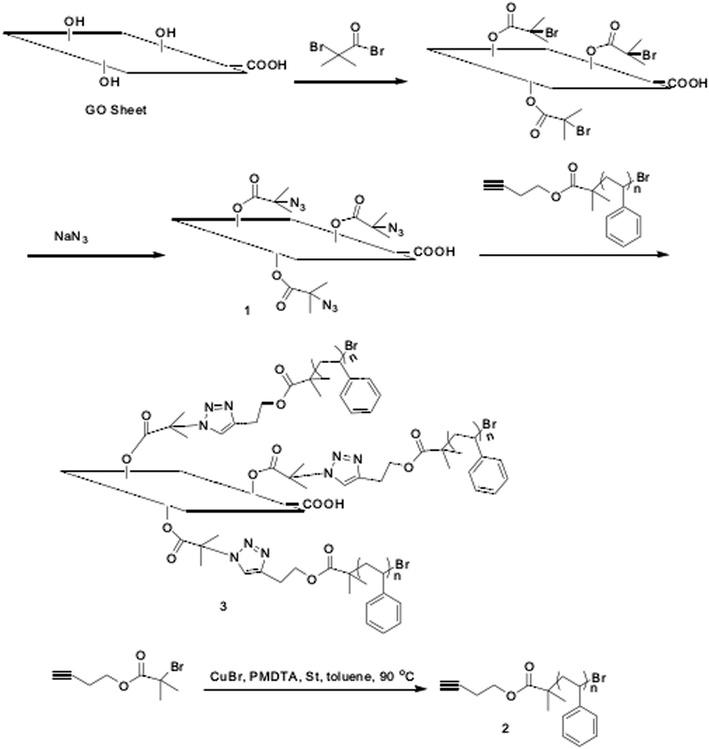 |
| Fig. 9 Synthetic route of polystyrene graft graphite oxide (GO/PS). This figure has been reproduced from ref. 80 with permission from Polymer, Copyright 2011. | |
Namvari et al.80 demonstrated novel reversible addition–fragmentation chain transfer agent (RAFT-CTA)-modified reduced graphene oxide nanosheets (CTA-rGONSs) by crosslinking rGONSs with a RAFT-CTA via esterification reaction. These nano CTA-rGONSs were used to polymerize a hydrophobic amino acid-based methacrylamide (N-acryloyl-L-phenylalanine methyl ester) monomer with different monomer/initiator ratios. The synthetic procedure is shown in detail in Fig. 10.
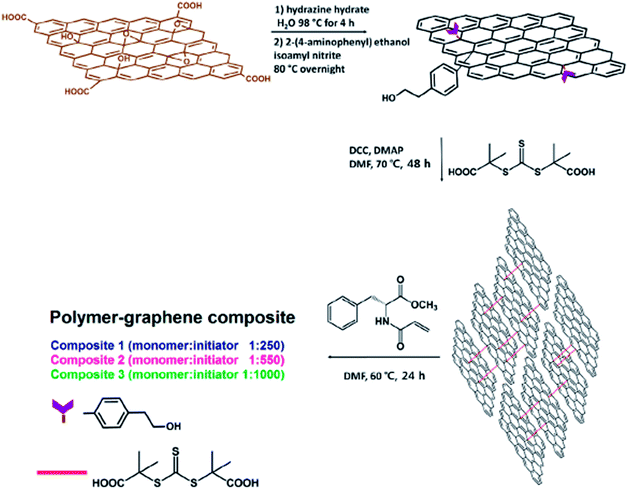 |
| Fig. 10 Preparation of polymer–graphene composites. This figure has been reproduced from ref. 81 with permission from J. Colloid Interface Sci., Copyright 2017. | |
5.1.3 Carboxyl functionalization. There are a large number of carboxyl groups at the edge of graphene oxide, and the carboxyl group is a highly reactive group, and thus has been greatly studied for the functionalization of graphene oxide.81,82 The carboxyl functionalization step is generally the activation of the reaction, and then the group containing an amino group and a hydroxyl group is dehydrated to form an ester or amide bond. The reagents commonly used for carboxyl activation include thionyl chloride (SOCl2),83 2-(7-aza-1H-benzotriazole-1-yl)-1,1,3,3-tetramethyluronium hexafluorophosphate,84 N,N-dicyclohexylcarbodiimide (DCC),85 and 1-ethyl-3-(3-dimethylaminopropyl)-carbodiimide (EDC).86 Ouyang et al.87 demonstrated the chemical functionalization of semiconducting graphene nanoribbons (GNRs) with Stone–Wales (SW) defects by carboxyl (COOH) groups. It was found that the geometrical structures and electronic properties of GNRs changed significantly, and the electrical conductivity of the system could be considerably enhanced by mono-adsorption and double adsorption of COOH, which sensitively depends on the axial concentration of SW defect COOH pairs (SWDCPs). With an increase of the axial concentration of SWDCPs, the system transformed from semiconducting behavior to p-type metallic behavior. This makes GNRs a possible candidate for application in chemical sensors and nanoelectronic devices. The preparation route and reaction conditions for the target hydroxy-based functionalization of graphene oxide are shown in Fig. 11.
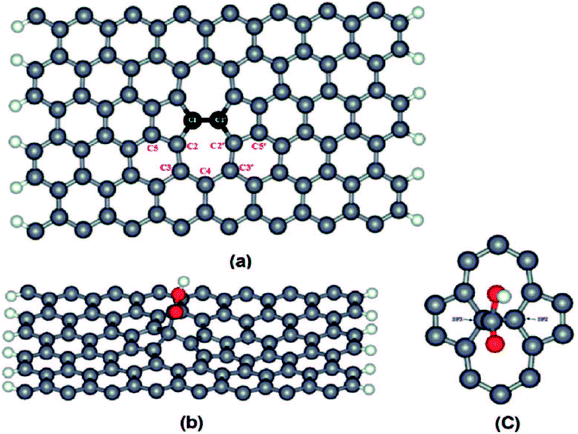 |
| Fig. 11 Preparation route and reaction conditions for the target hydroxy-based functionalization graphene oxide. This figure has been reproduced from ref. 88 with permission from J. Colloid Interface Sci., Copyright 2017. | |
Bonanni et al.88 removed the oxygen-containing groups from GO through a reductive treatment, and consequently reintroduced carboxyl groups onto the graphene surface. Carboxyl groups were inserted based on a free-radical-addition reaction, which occurred not only on the carbon atoms located at the edge plane, but also at the much more abundant basal plane of the graphene sheets. Specifically, chemically reduced graphene oxide (CRGO) was first functionalized with isobutyronitrile groups, which were generated from the thermal decomposition of azobisisobutyronitrile (AIBN) to give CRGO–CN. Subsequent reflux of CRGO–CN in a mixture of methanol and sodium hydroxide aqueous solution resulted in a hydrolysis reaction to provide CRGO enriched with carboxyl groups (CRGO–COOH), as shown in Fig. 12.
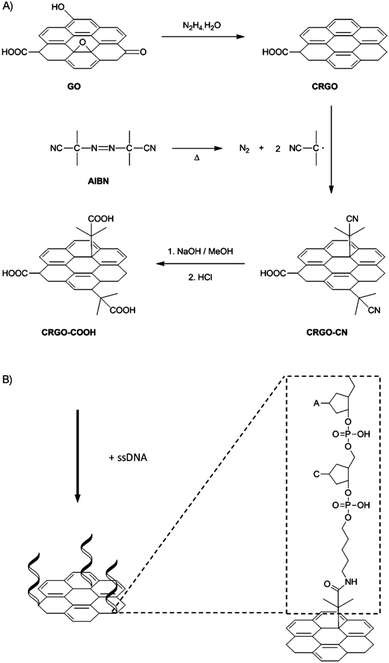 |
| Fig. 12 Schematic of the functionalization method. This figure has been reproduced from ref. 89 with permission from Chem.–Eur. J., Copyright 2014. | |
5.2 Non-covalent functionalization
The non-covalent bond functionalization of graphene or graphene oxide results in the formation of a composite material having a specific function by interaction between hydrogen bonds and electrostatic forces between graphene and functional molecules, the greatest advantage of which is maintaining the bulk structure and excellent properties of graphene or graphene oxide, and also improving the dispersibility and stability of graphene or graphene oxide. The methods for the functional modification of surface non-covalent bonds mainly include π–π bond interaction, hydrogen bonding, ionic bonding, and electrostatic interaction modification. The non-covalent bond functionalization process is simple with mild conditions, while maintaining the structure and properties of graphene. However, the disadvantage of this method is that other components (such as surfactants) are introduced.
5.2.1 π–π bond interaction. Song et al.89 inspired by interfacial interactions of protein matrix and the crystal platelets in nacre, produced a super tough artificial nacre through the synergistic interface interactions of p–p interaction and hydrogen bonding between graphene oxide (GO) nanosheets and sulfonated styrene–ethylene/butylene–styrene copolymer synthesized with multifunctional benzene. The resultant GO-based artificial nacre showed super-high toughness of 15.3 ± 2.5 MJ m−3, superior to that of natural nacre and other GO-based nanocomposites. The ultra-tough property of the novel nacre was attributed to the synergistic effect of the p–p stacking interactions and hydrogen bonding. Thus, this bioinspired synergistic toughening strategy opens a new avenue for constructing high performance GO-based nanocomposites in the near future.Lee et al.90 used a tetradecene derivative with a dendritic polyether branch as a modifier and the synergistic effect of an aromatic cyclic fluorene skeleton interacting with graphite and a polyether chain to induce high hydrophilicity, stripping graphite and stabilizing the graphene layer, as shown in Fig. 13. The same indole derivative did not improve the dispersibility of single-walled carbon nanotubes, indicating that the planar structure of carbon nanomaterials is a key factor in the formation of effective π-stacking. Due to the π–π bond, the absorption spectrum of tetraterpene derivatives appeared red shifted and their fluorescence was also quenched.
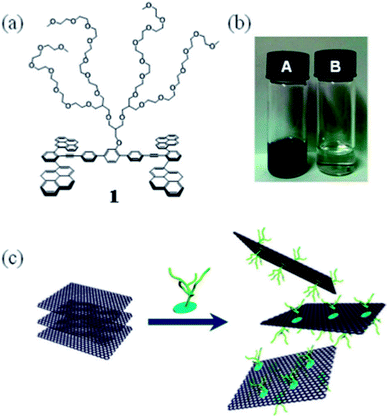 |
| Fig. 13 Exfoliation process of graphite and stabilization of graphene though the π–π interaction with tetrapyrene derivative. This figure has been reproduced from ref. 91 with permission from R. Soc. Chem., Copyright 2016. | |
5.2.2 Hydrogen bond interaction. He et al.91 researched the fabrication of reduced graphene oxide aerogel membranes (rGOAMs) via the reduction-induced self-assembly of rGO through hydrogen bond mediation. Using polyethylene glycol (PEG) as the mediator, the PEG–rGO hydrogen bonding interactions partly replaced the interlayer p–p and hydrophobic interactions during reduction, decreasing the rGO laminate size in 2D stacking and alleviating the structural shrinkage of the rGOA networks. The tight correlation between membrane pore size and porosity was broken, leading to rGOAMs with tunable pore sizes (0.62 to 0.33 mm) and high porosity (95%). The resultant rGOAMs could effectively reject oil-in-water emulsions with different sizes and exhibited ultrahigh water flux (up to 4890 L m−2 h−1) under 0.10 bar as well as a persistent anti-oil-fouling performance for up to 6 cycles.Patil et al.92 realized the surface functionalization of graphene by hydrogen bonding between graphene and DNA, which improved the hydrophilicity of graphene and stabilized it in water. On the other hand, the loading of organic molecules occurred on the surface of graphene. The functional modification of the graphene surface by hydrogen bonding does not introduce impurities, which is safe and reliable, and has important potential application prospects in biomedical field. A schematic illustration of aqueous dispersions of (a) GO, (b) GO–PDI and (c) GO–PyS through π–π interaction is shown in Fig. 14.
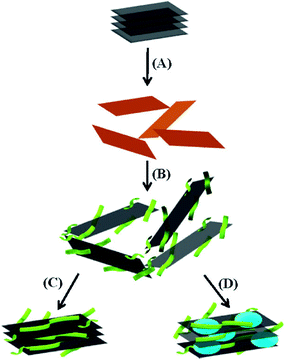 |
| Fig. 14 Schematic illustration of aqueous dispersions of (A) GO, (B) GO–PDI and (C) GO–PyS through π–π interaction and (D) co-assembly of negatively charged ssDNA-G sheets and positively charged cytochrome c produces co-intercalated multifunctional layered nanocomposites. This figure has been reproduced from ref. 93 with permission from Adv. Mater., Copyright 2009. | |
A novel graphene oxide–doxorubicin hydrochloride nanohybrid (GO–DXR) was prepared via a simple noncovalent method, and the loading and release behaviors of DXR on GO were investigated by Patil et al.93 The efficient loading of DXR on GO was as high as 2.35 mg mg−1 at the initial DXR concentration of 0.47 mg mL−1. The loading and release of DXR on GO showed strong pH dependence, which may be due to the hydrogen-bonding interaction between GO and DXR. The fluorescent spectrum and electrochemical results indicate that strong π–π stacking interaction exists between them, as shown in Fig. 15.
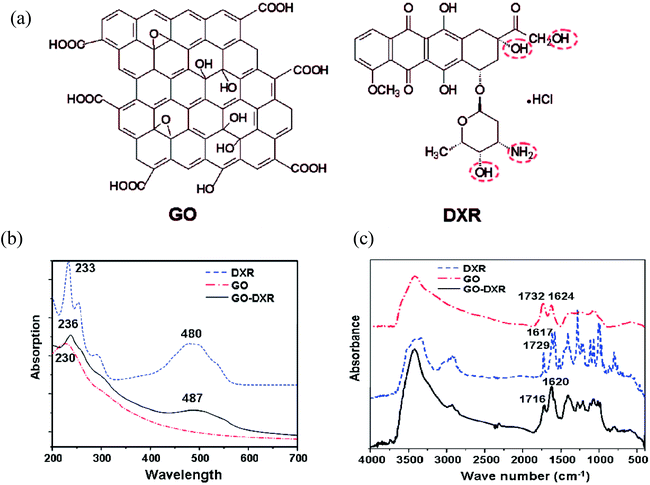 |
| Fig. 15 (a) Structure of graphene oxide (GO) and DXR, UV visible spectra (b) and FTIR spectra (c) of DXR, GO, and GO–DXR. This figure has been reproduced from ref. 94 with permission from Nano Lett., Copyright 2011. | |
5.2.3 Ion interaction. Choi et al.94 reported that a stable dispersion of reduced graphene was achieved in various organic solvents via noncovalent ionic interaction functionalization with amine-terminated polymers. An aqueous dispersion of reduced graphene was prepared via the chemical reduction of graphene oxide in aqueous media and vacuum filtered to generate reduced graphene sheets. Thorough FTIR and Raman spectroscopy investigation verified that the protonated amine terminal group of polystyrene underwent noncovalent functionalization with the carboxylate groups on the graphene surface, providing high dispersibility in various organic media, as shown in Fig. 16.
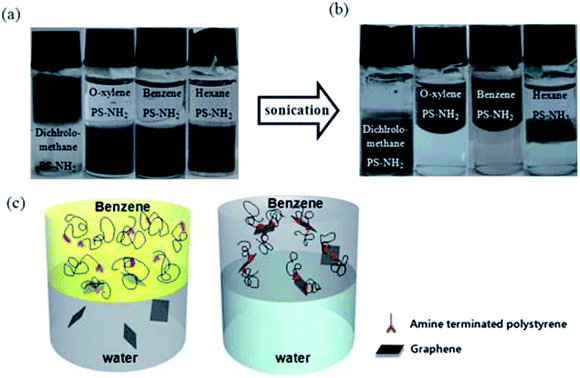 |
| Fig. 16 Transformation of the hydrophilic rGO into an organophilic rGO/polymer composite using an amine terminated polystyrene. This figure has been reproduced from ref. 95 with permission from J. Mater. Chem., Copyright 2010. | |
Ge et al.95 improved the mechanical and barrier properties of a starch film with reduced graphene oxide (RGO) modified by sodium dodecyl benzene sulfonate (SDBS). The hydrophilia of the modified RGO (r-RGO) was improved, which resulted in its good dispersion in the oxidized starch (OS) matrix. The tensile strength of the r-RGO-4/OS film increased to 58.5 MPa, which was more than three times that of the OS film (17.2 MPa). Besides, both the water vapor and oxygen barrier properties of the r-RGO/OS film improved greatly compared with that of the OS and GO/OS films. Moreover, the r-RGO/OS film could protect against UV light effectively due to its lightproof performance. In conclusion, the r-RGO/OS composite film has great potential applications in the packaging industry.
5.2.4 Electrostatic interaction. Electrostatic repulsion between the same type of charge is another strategy to improve the dispersion of graphene. Bhunia et al.60 used hydrazine as a reducing agent to control the reduction, while removing the functional groups such as hydroxyl groups and epoxy bonds of graphene oxide and retaining the carboxyl anion, which is well dispersed by charge repulsion. The chemical conversion of graphene can be conducted in water. Graphene oxide is soluble in water because its surface negative charge repels each other and forms a stable colloidal solution, as shown in Fig. 17.
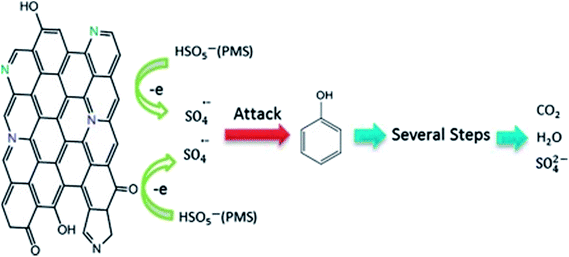 |
| Fig. 17 Synthesis of well-dispersed graphene by electrostatic repulsion. This figure has been reproduced from ref. 96 with permission from Nature Publishing Group, Copyright 2008. | |
Ge X. and Li H.96 prepared novel environmentally friendly Gemini surfactants, i.e. each with two hydrophilic and two hydrophobic groups. The reaction was conducted in hydrogen peroxide for the epoxidation of the carbon–carbon double bond. Methylene was used as the spacer group, and the nonionic hydrophilic head group was then introduced by ring-opening reaction. The targeted surfactants were synthesized via the reaction of chlorine sulfonic acid and hydroxy esterification. The results were compared with that for mixtures of the standard surfactants sodium decylsulfate and octaoxyethyleneglycol mono n-decyl ether under equivalent conditions. The surfactants were shown to exhibit improved performance over the mixed system both in terms of micellization and surface tension lowering.
5.3 Element doping
Element doping modification usually adopts annealing heat treatment, ion bombardment, arc discharge and other means to incorporate different elements into graphene, thereby resulting in the substitution of defects and vacancy defects in graphene, and maintaining the intrinsic two-dimensional structure of graphene. Simultaneously, its surface properties change to give new performances.97–100 Element doping adjusts the energy band structure of graphene, but the doping process is difficult to control quantitatively.
Duan et al.100 used thermal annealing to treat graphene and ammonium nitrate to prepare 6.54 at% nitrogen-doped graphene, which catalyzed the oxidative degradation of phenol by 5.4 times that of undoped graphene. The synergistic effect of B-, P- or N-doped graphene was studied. The detailed growth process of N-doped graphene by thermal annealing treatment is shown in Fig. 18.
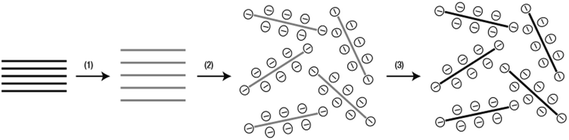 |
| Fig. 18 Detailed growth process of N-doped graphene by thermal annealing treatment. This figure has been reproduced from ref. 101 with permission from Catalysis, Copyright 2015. | |
Wei et al.101 reported a CVD technique to produce N-doped graphene, which was the first experimental example of substitutionally doped graphene, which was hard to produce using other methods. The CVD method is a nondestructive route to produce graphene and realizes substitutional doping since the doping is accompanied by the recombination of the carbon atoms in graphene during the CVD process. By using SEM, TEM, Raman, XPS and EDS, they demonstrated the existence of N-doped graphene. The CVD method can not only be used to produce N-doped graphene, but also has potential to produce the graphene doped with other elements. Moreover, they measured the electrical properties of the N-doped graphene. This research provides a new type of graphene experimentally, which is required for the further application of graphene. The synthesis of N-doped graphene by electrostatic repulsion is shown in Fig. 19.
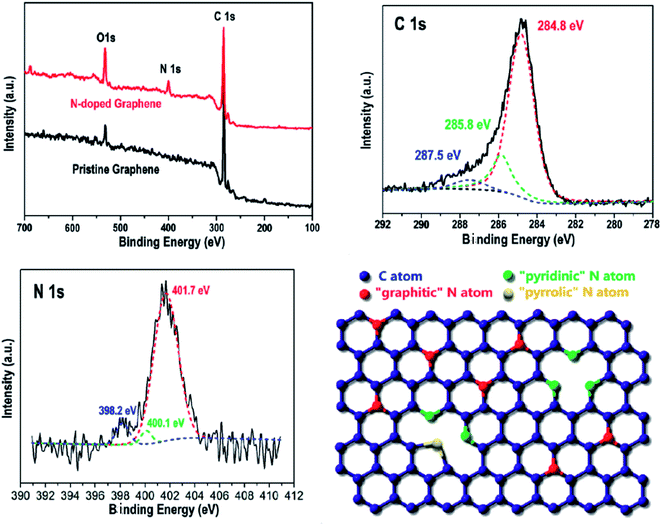 |
| Fig. 19 Synthesis of N-doped graphene by electrostatic repulsion. This figure has been reproduced from ref. 102 with permission from Nano Lett., Copyright 2009. | |
6. Summary of the performance and application of functionalized graphene
The functional modification of graphene is of great significance for the application of graphene composites. According to previously mentioned literature, the surface functional modification of graphene and graphene oxide is used to obtain related products and the interactions and reactions of the modification types have been studied, briefly explaining the functional characteristics and application fields of graphene surface-functionalized composites, as summarized in Table 2. Obviously, this table will be supplemented and improved as the research on graphene continues.
Table 2 Properties and applications of functionalized graphene and graphene oxide
Modification type |
Modified group |
Modification agent |
Interaction type |
Property |
Application |
Ref. |
Covalent functionalization |
–C C– |
4-Propargyloxydia-zobenzenetetrafluoroborate |
Diazotization |
Water soluble |
Biosensors |
77 |
–OH |
2-Bromoisobutyryl bromide, NaN3, HC C–PS |
Esterification |
Good solubility |
Polymer composites |
79 |
–COOH |
SOCl2 |
Esterification |
Conductive |
Conducted membrane |
84 |
–OH |
N2H4, DNA |
Addition esterification |
Good solubility |
Biosensors |
89 |
Non-covalent functionalization |
Carbon six-membered |
Sulfonated styrene–ethylene/butylene–styrene copolymer |
Copolymerization |
Conductive |
Nanocomposites |
90 |
Carbon six-membered ring |
Tetrapyrene derivative |
π–π |
Stable and dispersed, conductive |
Sensors |
91 |
–OH |
DNA |
Hydrogen bond interaction |
Stable and dispersed, good solubility |
Biomedicine |
93 |
–OH |
DXR |
Hydrogen bond interaction |
Stable and dispersed, good solubility |
Drug carriers |
94 |
–COOH |
Amine-terminated polymers |
Ion interaction |
Stable and dispersed, good solubility |
— |
95 |
–COOH |
SDBS |
Ion interaction |
Stably dispersed, conductive |
Packaging |
96 |
–COO– |
Hydrazine |
Electrostatic interaction |
Stably dispersed |
— |
71 |
Element doping |
–C– |
B, P, and N |
— |
Band structure change |
Electronic devices |
101 |
7. Future prospects
Graphene has many unique and prominent physical and chemical properties (large specific surface area, high transparency and excellent mechanical/thermal/electrical/electrochemistry properties) due to its special structure, and thus has wide application potential in many fields, such as heterojunction solar cells,102 medicine,103 energy,104 water splitting, biosensing, bioimaging, environmental studies, catalysis, photocatalysis, and biomedical technologies.105,106 However, a coin has two sides. Because of the unique structure of graphene, it is difficult to disperse in water and organic solvents, which is known as the agglomeration phenomenon of graphene. This problem limits the application of graphene. Therefore, it is important to functionalize graphene and graphene oxide to expand their various applications.
Currently, the study of these functional methods is still in the experimental stage, and researchers are mainly focused on the development of new product varieties and their characterization and application. However, there are several problems as follows. (1) Functionalized target groups difficult to control, (2) the synthetic procedures are complicated, and (3) separation and purification are very difficult.
Thus, considering the above-mentioned superior performance, we should pay attention to the relationship between the structure and properties of graphene and graphene oxide in future work. For example, according to the quantitative structure and property relationship principle, functional groups are introduced into molecules based on functionalized target group design. Functionalized target group design is carried out by molecular simulation technology, and the structure property model is set up to guide the synthesis of new products. However, the synthetic process of different types of graphene and graphene oxide is complex, which involves the introduction of many other special groups, thus it is difficult to control the reaction and obtain the target products. Also, some reaction conditions are extremely harsh. Nevertheless, considering the optimization of reaction procedures and environmentally friendly synthetic routes, graphene and graphene oxide will achieve industrialization. Of course, other new functional modification methods should be developed, such as non-alkali carbonylation and carbon–carbon multi-bond addition reactions, which are rarely reported in functional applications.
8. Concluding remarks
Graphene-based composites are involved in many fields with the development of science, such as graphene-based drug carriers to improve the stability, toxicity, metabolic kinetics of drugs, and many aspects are still unclear, pending further research and exploration by scientists. Accordingly, graphene and graphene oxide will be researched completely and use widely in the future.
Conflicts of interest
There are no conflicts to declare.
Acknowledgements
This work reported in this paper was supported by the Sichuan Science and Technology Program, Sichuan Youth Scientific and Technological Innovation Research Team of Agricultural Machinery Equipment (2017TD0023); Project of Sichuan Provincial Department of Education, Robot and Intelligent Equipment Research and Innovation Team of Sichuan Universities (15TD0016).
References
- A. K. Geim and K. S. Novoselov, The rise of graphene, Nat. Mater., 2007, 6(3), 183–191 CrossRef CAS PubMed.
- R. F. Curl and R. E. Smalley, Probing C60, Science, 1988, 242(4881), 1017–1022 CrossRef CAS PubMed.
- W. Krätschmer, L. D. Lamb and K. Fostiropoulos, et al., Solid C60: a new form of carbon, Nature, 1990, 347(6291), 354–358 CrossRef.
- S. Lijima, Helical microtubules of graphitic carbon, Nature, 1991, 354(6348), 56–58 CrossRef.
- K. S. Novoselov, Electric Field Effect in Atomically Thin Carbon Films, Science, 2004, 306(5696), 666–669 CrossRef CAS PubMed.
- J. C. Slonczewski and P. R. Weiss, Band structure of graphite, J. Chem. Phys., 1958, 21(12), 2238–2239 Search PubMed.
- Y. Hang, Y. W. Tan and H. L. Stormer, et al., Experimental observation of the quantum Hall effect and Berry's phase in graphene, Nature, 2005, 438(7065), 201–204 CrossRef PubMed.
- K. S. Novoselov, Z. Jiang and Y. Zhang, et al., Room-temperature Quantum Hall Effect in graphene, Science, 2007, 315(5817), 1379 CrossRef CAS PubMed.
- K. S. Novoselov, A. K. Geim and S. V. Morozov, et al., Two-dimensional gas of massless Dirac fermions in graphene, Nature, 2005, 438(7065), 197–200 CrossRef CAS PubMed.
- K. Wang, M. Xu and Y. Gu, et al., Low-temperature plasma exfoliated n-doped graphene for symmetrical electrode supercapacitors, Nano Energy, 2017, 31, 486–494 CrossRef CAS.
- V. Singh, D. Joung and Z. Lei, et al., Graphene based materials: past, present and future, Prog. Mater. Sci., 2011, 56(8), 1178–1271 CrossRef CAS.
- A. Mehta, A. Mishra and S. Basu, et al., Band gap tuning and surface modification of carbon dots for sustainable environmental remediation and photocatalytic hydrogen production - A review, J. Environ. Manage., 2019, 250(15), 109486 CrossRef CAS PubMed.
- N. R. Reddy, U. Bhargav and M. M. Kumari, et al., Highly efficient solar light-driven photocatalytic hydrogen production over Cu/FCNTs-titania quantum dots-based heterostructures, J. Environ. Manage., 2020, 254, 109747 CrossRef CAS PubMed.
- Q. Liu, J. H. Sun and K. Gao, et al., Graphene quantum dots for energy storage and conversion: from fabrication to applications, Mater. Chem. Front., 2020, 4(2), 421–436 RSC.
- H. J. Li, D. J. Qian and M. Chen, Template less Infrared Heating Process for Fabricating Carbon Nitride Nanorods with Efficient Photocatalytic H2 Evolution, ACS Appl. Mater. Interfaces, 2015, 7(45), 25162–25170 CrossRef CAS PubMed.
- K. R. Reddy, C. H. V. Reddy and M. N. Nadagouda, et al., Polymeric graphitic carbon nitride (g-C3N4)-based semiconducting nanostructured materials: synthesis methods, properties and photocatalytic applications, J. Environ. Manage., 2019, 238, 25–40 CrossRef CAS PubMed.
- H. Enamul, Y. Yusuke and M. Victor, et al., Nanoarchitectured Graphene-Organic Frameworks (GOFs): Synthetic Strategies, Properties, and Applications, Chem.–Asian J., 2018, 13, 3561–3574 CrossRef PubMed.
- T. Ueno, T. Yoshioka and J. i. Ogawa, et al., Highly thermal conductive metal/carbon composites by pulsed electric current sintering, Synth. Met., 2009, 159, 2170–2172 CrossRef CAS.
- A. K. Geim and K. S. Novoselov, The rise of graphene, Nat. Mater., 2007, 6(3), 183–191 CrossRef CAS PubMed.
- D. R. Dreyer, A. D. Todd and C. W. Bielawski, Harnessing the chemistry of graphene oxide, Chem. Soc. Rev., 2009, 39(1), 228–240 RSC.
- S. Stankovich, D. A. Dikin and R. D. Piner, et al., Synthesis of graphene-based nanosheets via chemical reduction of exfoliated graphite oxide, Carbon, 2007, 45(7), 1558–1565 CrossRef CAS.
- R. R. Nair and A. K. Geim, Unimpeded Permeation of water through helium-leak-tight graphene-based membrane, Science, 2012, 335(6067), 442–444 CrossRef CAS PubMed.
- Z. Liu, J. T. Robinson and X. Sun, et al., PEGylated Nano-Graphene Oxide for Delivery of Water Insoluble Cancer Drugs, J. Am. Chem. Soc., 2008, 130(33), 10876–10877 CrossRef CAS PubMed.
- Y. Feng, Z. Wang and R. Zhang, et al., Anti-fouling graphene oxide based nanocomposites membrane for oil-water emulsion separation, Water Sci. Technol., 2018, 77(5), 1179–1185 CrossRef CAS PubMed.
- Q. Li, F. Fei and W. Yang, et al., Enzyme Immobilization on Carboxyl-Functionalized Graphene Oxide for Catalysis in Organic Solvent, Ind. Eng. Chem. Res., 2013, 52(19), 6343–6348 CrossRef CAS.
- J. Liu, Y. Xue and Y. Gao, et al., Hole and electron extraction layers based on graphene oxide derivatives for high-performance bulk heterojunction solar cells, Adv. Mater., 2012, 24(17), 2228–2233 CrossRef CAS PubMed.
- C. Murat, R. R. Kakarla and A. M. Fernando, et al., Advanced electrochemical energy storage supercapacitors based on the flexible carbon fiber fabric-coated with uniform coral-like MnO2 structured electrodes, Chem. Eng. J., 2017, 309, 151–158 CrossRef.
- S. Kumar, S. D. Bukkitgar and S. Singh, et al., Electrochemical Sensors and Biosensors Based on Graphene Functionalized with Metal Oxide Nanostructures for Healthcare Applications, ChemistrySelect, 2019, 4, 5322–5337 CrossRef CAS.
- N. P. Shettia, S. J. Malodea and D. S. Nayaka, et al., A novel biosensor based on graphene oxide-nanoclay hybrid electrode for the detection of Theophylline for healthcare applications, Microchem. J., 2019, 149, 103985 CrossRef.
- C. Berger, Z. Song and T. Li, et al., Ultrathin Epitaxial Graphite: 2D Electron Gas Properties and a Route toward Graphene-based Nanoelectronics, J. Phys. Chem., 2004, 108(52), 19912–19916 CrossRef CAS.
- Y. Zhang, L. Zhang and C. Zhou, Review of Chemical Vapor Deposition of Graphene and Related Applications, Chem. Res., 2013, 46(10), 2329–2339 CrossRef CAS PubMed.
- S. Stankovich, R. D. Piner, X. Chen, N. Wu, S. T. Nguyen and R. S. Ruoff, Stable Aqueous Dispersions of Graphitic Nanoplatelets via the Reduction of Exfoliated Graphite Oxide in the Presence of Poly(sodium 4-styrenesulfonate), J. Mater. Chem., 2006, 16(2), 155–158 RSC.
- J. I. Paredes, S. Villar-Rodil and P. Solis-Fernandez, et al., Preparation, characterization and fundamental studies on graphenes by liquid-phase processing of graphite, J. Alloys Compd., 2012, 536(supp_S1), S450–S455 CrossRef CAS.
- C. R. Dean, A. F. Young and I. Meric, et al., Boron nitride substrates for high-quality graphene electronics, Nat. Nanotechnol., 2010, 5(10), 722–726 CrossRef CAS PubMed.
- A. S. Mayorov, R. V. Gorbachev and S. V. Morozov, et al., Micrometer-scale ballistic transport in encapsulated graphene at room temperature, Nano Lett., 2011, 11, 2396 CrossRef CAS PubMed.
- R. R. Nair, P. Blake and A. N. Grigorenko, et al., Fine Structure Constant Defines Visual Transparency of Graphene, Science, 2008, 320, 1308 CrossRef CAS PubMed.
- H. He, T. Riedl and A. Lerf, et al., Solid-state NMR studies of the structure of graphite oxide, J. Phys. Chem., 1996, 100(51), 19954–19958 CrossRef CAS.
- A. Lerf, H. He and M. Forster, et al., Structure of graphite oxide revisited, J. Phys. Chem. B, 1998, 102(23), 4477–4482 CrossRef CAS.
- K. Erickson, R. Erni and Z. Lee, et al., Determination of the local chemical structure of graphene oxide and reduced graphene oxide, Adv. Mater., 2010, 22(40), 4467–4472 CrossRef CAS PubMed.
- S. Eigler and A. Hirsch, Chemistry with graphene and graphene oxide-challenges for synthetic chemists, Angew. Chem., Int. Ed., 2014, 53(30), 7720–7738 CrossRef CAS PubMed.
- A. Dimiev, D. V. Kosynkin and L. B. Alemany, et al., Pristine graphite oxide, J. Am. Chem. Soc., 2012, 134(5), 2815–2822 CrossRef CAS PubMed.
- J. P. Rourke, P. A. Pandey and J. J. Moore, et al., The real graphene oxide revealed: stripping the oxidative debris from the graphene-like sheets, Angew. Chem., 2011, 123(14), 3231–3235 CrossRef.
- V. Georgakilas, J. N. Tiwari and K. C. Kemp, et al., Noncovalent Functionalization of Graphene and Graphene Oxide for Energy Materials, Biosensing, Catalytic, and Biomedical Applications, Chem. Rev., 2016, 116, 5464–5519 CrossRef CAS PubMed.
- B. C. Brodie, On the Atomic Weight of Graphite, Philos. Trans. R. Soc. London, 2009, 149(1), 249–259 Search PubMed.
- L. Staudenmaier, Verfahren zur Darstellung der Graphitsäure, Ber. Dtsch. Chem. Ges., 1898, 31(2), 1481–1487 CrossRef CAS.
- W. S. Hummers and R. E. Offeman, Preparation of Graphitic Oxide, J. Am. Chem. Soc., 1958, 80, 1339 CrossRef CAS.
- L. D. David, V. Mercedes and S. Maria, et al., The Role of Oxidative Debris on Graphene Oxide Films, ChemPhysChem, 2013, 14(17), 4002–4009 CrossRef PubMed.
- S. Eigler and A. Hirsch, Chemistry with Graphene and Graphene Oxide-Challenges for Synthetic Chemists, Angew. Chem., Int. Ed., 2014, 53(30), 7720–7738 CrossRef CAS PubMed.
- L. Peng, Z. Xu and Z. Liu, et al., An iron-based green approach to 1-h production of single-layer graphene oxide, Nat. Commun., 2015, 6, 5716 CrossRef CAS PubMed.
- D. C. Marcano, D. V. Kosynkin and J. M. Berlin, et al., Improved synthesis of graphene oxide, ACS Nano, 2010, 4(8), 4806–4814 CrossRef CAS PubMed.
- P. Vineeta, K. Arvind and S. Raghuvir, et al., Nickel-Decorated Graphene Oxide/Polyaniline Hybrid: A Robust and Highly Efficient Heterogeneous Catalyst for Hydrogenation of Terminal Alkynes, Ind. Eng. Chem. Res., 2015, 54(45), 11493–11499 CrossRef.
- J. Shen, Y. Hu and M. Shi, et al., Fast and facile preparation of graphene oxide and reduced graphene oxide nanoplatelets, Chem. Mater., 2009, 21(15), 3514–3520 CrossRef CAS.
- N. I. Kovtyukhova, P. J. Ollivier and B. R. Martin, et al., Layer-by-Layer Assembly of Ultrathin Composite Films from Micron-Sized Graphite Oxide Sheets and Polycations, Chem. Mater., 1999, 11(3), 771–778 CrossRef CAS.
- L. Staudenmaier, Verfahren zur darstellung der graphitsaure, Eur. J. Inorg. Chem., 2010, 32(2), 1394–1399 CrossRef.
- D. R. Dreyer, S. Park and C. W. Bielawski, et al., The chemistry of graphene oxide, Chem. Soc. Rev., 2010, 39(1), 228–240 RSC.
- C. Botas, P. Álvarez and C. Blanco, et al., The effect of the parent graphite on the structure of graphene oxide, Carbon, 2012, 50(1), 275–282 CrossRef CAS.
- S. Eigler, M. Enzelberger-heim and S. Grimm, et al., Wet chemical synthesis of graphene, Adv. Mater., 2013, 25(26), 3583–3587 CrossRef CAS PubMed.
- S. Eigler, C. Dotzer and A. Hirsch, et al., Formation and decomposition of CO2 intercalated graphene oxide, Chem. Mater., 2012, 24(7), 1276–1282 CrossRef CAS.
- C. I. Wang, A. P. Periasamy and H. T. Chang, Photoluminescent C-dots@RGO probe for sensitive and selective detection of acetylcholine, Anal. Chem., 2013, 85(6), 3263–3270 CrossRef CAS PubMed.
- P. Bhunia, E. Hwang and M. Min, et al., A non-volatile memory device consisting of graphene oxide covalently functionalized with ionic liquid, Chem. Commun., 2011, 48(6), 913–915 RSC.
- Y. Si and E. T. Samulski, Synthesis of water soluble graphene, Nano Lett., 2008, 8(6), 1679–1682 CrossRef CAS PubMed.
- Y. S. Choi, C. S. Yeo and S. J. Kim, et al., Multifunctional reduced graphene oxide-CVD graphene core–shell fibers, Nanoscale, 2019, 11, 12637 RSC.
- C. Y. Su, Y. Xu and W. Zhang, et al., Highly efficient restoration of graphitic structure in graphene oxide using alcohol vapors, ACS Nano, 2010, 4(9), 5285–5292 CrossRef CAS PubMed.
- G. Wang, J. Yang and J. Park, et al., Facile synthesis and characterization of graphene nanosheets, J. Phys. Chem. C, 2008, 112(22), 8192–8195 CrossRef CAS.
- H. Kim, A. Abdala and C. W. Macosko, Graphene/Polymer Nanocomposites, Macromolecules, 2010, 43, 6515–6530 CrossRef CAS.
- Y. Fadil, V. Agarwal and F. Jasinski, Electrically conductive polymer/rGO nanocomposite films at ambient temperature via miniemulsion polymerization using GO as surfactant, Nanoscale, 2019, 11, 6566 RSC.
- S. Stankovich, D. A. Dikin and R. D. Piner, et al., Synthesis of graphene-based nanosheets via chemical reduction of exfoliated graphite oxide, Carbon, 2007, 45(7), 1558–1565 CrossRef CAS.
- S. Gilje, S. Han and M. Wang, et al., A chemical route to graphene for device application, Nano Lett., 2007, 7(11), 3394–3398 CrossRef CAS PubMed.
- G. Williams, B. Seger and P. V. Kamat, TiO2-graphene nanocomposite. UV-assisted photocatalytic reduction of graphene oxide, ACS Nano, 2008, 2(7), 1487–1491 CrossRef CAS PubMed.
- P. Bhunia, E. Hwang and M. Min, et al., A non-volatile memory device consisting of graphene oxide covalently functionalized with ionic liquid, Chem. Commun., 2011, 48(6), 913–915 RSC.
- C. K. Chua and M. Pumera, Covalent chemistry on graphene, Chem. Soc. Rev., 2013, 42(8), 3222 RSC.
- S. H. C. Man, S. C. Thickett and M. R. Whittaker, et al., Synthesis of polystyrene nanoparticles “Armoured” with nanodimensinol Graphene oxide sheets by miniemulsion polymerization, Chem. Soc. Rev., 2012, 51, 47–58 Search PubMed.
- A. Sinitskii, A. Dimiev and D. A. Corley, et al., Kinetics of Diazonium Functionalization of Chemically Converted Graphene Nanoribbons, ACS Nano, 2010, 4(4), 1949–1954 CrossRef CAS PubMed.
- Z. Xia, F. Leonardi and M. Gobbi, et al., Electrochemical Functionalization of Graphene at the Nanoscale with Self-Assembling Diazonium Salts, ACS Nano, 2016, 10(7), 7125 CrossRef CAS PubMed.
- D. Bouša, O. Jankovsky and D. Sedmidubsky, et al., Mesomeric Effects of Graphene Modified with Diazonium Salts: Substituent Type and Position Influence its Properties, Chemistry, 2015, 21(49), 17728–17738 CrossRef PubMed.
- Z. Jin, T. P. Mcnicholas and C. J. Shih, et al., Click Chemistry on Solution-Dispersed Graphene and Monolayer CVD Graphene, Chem. Mater., 2011, 23(14), 3362–3370 CrossRef CAS.
- A. K. Farquhar, H. M. Dykstra and M. R. Waterland, et al., The Spontaneous Modification of Free-Floating Few-Layer Graphene by Aryldiazonium Ions: Electrochemistry, AFM and Infrared Spectroscopy from Grafted Films, J. Phys. Chem. C, 2011, 120(14), 7543 CrossRef.
- Y. Xiong, Y. Xie and F. Zhang, et al., Reduced graphene oxide/hydroxylated styrene–butadiene–styrene tri-block copolymer electroconductive nanocomposites: preparation and properties, Mater. Sci. Eng., B, 2012, 177(14), 1157–1163 CrossRef CAS.
- X. Yang, L. Ma and S. Wang, et al., “Clicking” graphite oxide sheets with well-defined polystyrenes: a new Strategy to control the layer thickness, Polymer, 2011, 52(14), 3046–3052 CrossRef CAS.
- M. Namvari, C. S. Biswas and Q. Wang, et al., Crosslinking hydroxylated reduced graphene oxide with RAFT-CTA: a nano-initiator for preparation of well-defined amino acid-based polymer nanohybrids, J. Colloid Interface Sci., 2017, 504, 731–740 CrossRef CAS PubMed.
- H. K. Choi, Y. Oh and H. Jung, et al., Influences of carboxyl functionalization of intercalators on exfoliation of graphite oxide: a molecular dynamics simulation, Phys. Chem. Chem. Phys., 2018, 20(45), 28616 RSC.
- F. Ouyang, B. Huang and Z. Li, et al., Chemical Functionalization of Graphene Nanoribbons by Carboxyl Groups on Stone-Wales Defects, J. Phys. Chem. C, 2008, 112(31), 12003–12007 CrossRef CAS.
- Y. Cao, Z. Lai and J. Feng, et al., Graphene oxide sheets covalently functionalized with block copolymers via click chemistry as reinforcing fillers, J. Mater. Chem., 2011, 21(25), 9271 RSC.
- N. Mohanty and V. Berry, Graphene-Based Single-Bacterium Resolution Biodevice and DNA Transistor: Interfacing Graphene Derivatives with Nanoscale and Microscale Biocomponents, Nano Lett., 2008, 8(12), 4469–4476 CrossRef CAS PubMed.
- J. Liu, Z. Liu and C. J. Barrow, et al., Molecularly engineered graphene surfaces for sensing applications: a review, Anal. Chim. Acta, 2015, 859, 1–19 CrossRef CAS PubMed.
- Z. Liu, J. T. Robinson and X. Sun, et al., PEGylated Nano-Graphene Oxide for Delivery of Water Insoluble Cancer Drugs, J. Am. Chem. Soc., 2008, 130(33), 10876–10877 CrossRef CAS PubMed.
- F. Ouyang, B. Huang and Z. Li, et al., Chemical Functionalization of Graphene Nanoribbons by Carboxyl Groups on Stone-Wales Defects, J. Phys. Chem. C, 2008, 112(31), 12003–12007 CrossRef CAS.
- A. Bonanni, C. K. Chua and M. Pumera, Rational Design of Carboxyl Groups Perpendicularly Attached to a Graphene Sheet: A Platform for Enhanced Biosensing Applications, Chem.–Eur. J., 2014, 20(1), 217–222 CrossRef CAS PubMed.
- P. A. Song, Z. G. Xu and Y. P. Wu, et al., Super-tough artificial nacre based on graphene oxide via synergistic interface interactions of π-π stacking and hydrogen bonding, Carbon, 2017, 111, 807–812 CrossRef CAS.
- D. W. Lee, T. Kim and M. Lee, An amphiphilic pyrene sheet for selective functionalization of graphene, Chem. Commun., 2011, 47(29), 8259 RSC.
- M. He, R. Zhang and K. Zhang, et al., Reduced graphene oxide aerogel membranes through hydrogen bond mediation for highly efficient oil/water separation, J. Mater. Chem. A, 2019, 7, 11468 RSC.
- A. J. Patil, J. L. Vickery and T. B. Scott, et al., Aqueous Stabilization and Self-Assembly of Graphene Sheets into Layered Bio-Nanocomposites using DNA, Adv. Mater., 2009, 21(31), 3159–3164 CrossRef CAS.
- A. S. Mayorov, R. V. Gorbachev and S. V. Morozov, et al., Micrometer-Scale Ballistic Transport in Encapsulated Graphene at Room Temperature, Nano Lett., 2011, 11(6), 2396–2399 CrossRef CAS PubMed.
- E. Y. Choi, T. H. Han, J. Hong, J. E. Kim, S. H. Lee, H. W. Kim and S. O. Kim, Noncovalent Functionalization of Graphene with End Functional Polymers, J. Mater. Chem., 2010, 20, 1907–1912 RSC.
- X. Ge, H. Li and L. Wu, et al., Improved mechanical and barrier properties of starch film with reduced graphene oxide modified by SDBS, J. Appl. Polym. Sci., 2017, 134(22), 258–264 CrossRef.
- D. Li, M. B. Müller and S. Gilje, et al., Processable aqueous dispersions of graphene nanosheets, Nat. Nanotechnol., 2008, 3(2), 101–105 CrossRef CAS PubMed.
- H. Bai, Y. Xu and L. Zhao, et al., Non-covalent functionalization of graphene sheets by sulfonated polyaniline, Chem. Commun., 2009, 13, 1667 RSC.
- X. Wang and G. Shi, Introduction to the chemistry of graphene, Phys. Chem. Chem. Phys., 2015, 17(43), 28484–28504 RSC.
- B. W. Yao, C. Li, J. Ma and G. Q. Shi, Porphyrin-based graphene oxide frameworks with ultra-large d-spacings for the electrocatalyzation of oxygen reduction reaction, Phys. Chem. Chem. Phys., 2015, 17(29), 9538–19545 RSC.
- X. Duan, S. Indrawirawan and H. Sun, et al., Effects of nitrogen-, boron-, and phosphorus-doping or codoping on metal-free graphene catalysis, Catalysis, 2015, 249, 184–191 CAS.
- D. Wei, Y. Liu and Y. Wang, et al., Synthesis of N-Doped Graphene by Chemical Vapor Deposition and Its Electrical Properties, Nano Lett., 2009, 9(5), 752–1758 CrossRef PubMed.
- P. H. Ho, Y. T. Liou and C. H. Chuang, et al., Self-Crack-Filled Graphene Films by Metallic Nanoparticles for High-Performance Graphene Heterojunction Solar Cells, Adv. Mater., 2015, 27(10), 1724–1729 CrossRef CAS PubMed.
- T. Nezakati, B. G. Cousins and A. M. Seifalian, Toxicology of chemically modified graphene-based materials for medical application, Arch. Toxicol., 2012, 88(11), 1987–2012 CrossRef PubMed.
- Y. W. Son, M. L. Cohen and S. G. Louie, Energy Gaps in Graphene Nanoribbons, Phys. Rev. Lett., 2006, 97(21), 216803 CrossRef PubMed.
- A. D. Sanctis, S. Russo and M. F. Craciun, et al., New routes to the functionalization patterning and manufacture of graphene-based materials for biomedical applications, J. R. Soc., Interface, 2018, 8(3), 20170057 Search PubMed.
- V. Georgakilas, J. N. Tiwari and K. C. Kemp, et al., Noncovalent Functionalization of Graphene and Graphene Oxide for Energy Materials, Biosensing, Catalytic, and Biomedical Applications, Chem. Rev., 2016, 116(9), 5464 CrossRef CAS PubMed.
|
This journal is © The Royal Society of Chemistry 2020 |