DOI:
10.1039/D0RA00425A
(Paper)
RSC Adv., 2020,
10, 11624-11633
Preparation of sheep bone collagen peptide–calcium chelate using enzymolysis-fermentation methodology and its structural characterization and stability analysis
Received
30th January 2020
, Accepted 11th March 2020
First published on 24th March 2020
Abstract
In this study, enzymatic hydrolysis and Lactobacillus fermentation were used in combination to prepare collagen peptide with high free calcium content, followed by the addition of anhydrous ethanol to obtain peptide–calcium chelate. The optimal conditions for the fermentation of enzymatic hydrolysate (glucose 3%, inoculum size 6%, 24.5 h, 37 °C and pH 6.5) were determined by response surface methodology (RSM), under which a free calcium content of 2212.58 mg/100 g was obtained. The calcium–chelating capacity was 42.57 ± 0.09%. The results of ultraviolet absorption spectrum, Fourier transform infrared (FT-IR) spectra, differential scanning calorimeter (DSC), X-ray diffraction and amino acid analysis indicated that calcium could be chelated through carboxyl oxygen and amino nitrogen atoms of collagen peptides, forming peptide–calcium chelate. The chelate is stable at 30–80 °C of temperatures and during in simulated gastrointestinal digestion, which could promote calcium absorption in human. The test intended to provide a basis for developing a novel calcium supplement and promoting utilization of sheep bone.
1. Introduction
Meat accounts for a large proportion of food consumption, and the annual bone production increases with the increase of meat consumption in the world.1 In China, the annual output of mutton is up to 4.88 million tons, meanwhile about 20 million tons of bones are produced every year. The bone resources of livestock and poultry are extremely rich.2 Sheep bone which is a by-product of mutton processing, was often discarded or used to produce low value feed as well.3 Bone contains various minerals such as calcium, phosphor, iron, zinc, cuprum, etc., as well as the nitrogenous collagen and amino acids. The collagen which occupies 90% of the bone protein can be hydrolyzed into amino acids and polypeptides. Collagen peptide is bioactive and helps to exert every function of collagen to the full length.4 The average of the components in sheep bone: water: 64.7%, protein: 16.7%, fat: 5.1%, ash: 11.9%. Therefore, it is very important to understand how to make full use of collagen and calcium resources of sheep bone and thus improve the comprehensive utilization efficiency of fresh bone.
Calcium as an essential nutrient, accounts for 1.5–2.2% of the total weight of the human body.5 Calcium is associated with bone health, cellular metabolism, blood clotting, nerve conduction and cardiac function.6,7 Generally speaking, the average daily intake of calcium (RDA) is about 800 mg for adults, and 1200 mg for adolescents and the elderly.8 Calcium deficiency may lead to metabolic bone diseases such as rickets in children and osteoporosis in the elderly, even induce more serious diseases such as osteomalacia, colon cancer and kidney stones.9,10 Calcium can be supplied in salts, metal chelating agents, and calcium-chelating peptides.11 Calcium gluconate, calcium carbonate and calcium lactate are the most common and available ionic calcium in the body.12 But the biggest drawback is that ionized calcium is prone to calcium precipitation in the basic intestinal environment.13 Therefore, dietary calcium absorption and bioavailability are severely reduced. For example, excessive intake of calcium carbonate may cause gastrointestinal side effects, including constipation and flatulence.14 The peptide–calcium chelates remain soluble and electrically neutral during digestion.15 The absorption of calcium in the chelate depends on the ligand, and the absorption of the small peptide has various advantages, such as less energy consumption and faster transport and the carrier is not easy to saturate.16 Peptides can chelate with calcium to form soluble and stable complexes that have been shown to be effective in promoting calcium absorption.17 Therefore, peptide–calcium chelate could be used as calcium nutritional supplement to improve calcium absorption and bioavailability and has potential application value.
Hence, the objective of this study is to utilize sheep bone as a resource to produce calcium-chelating peptides. Peptides and free calcium obtained from bone by enzymolysis-fermentation chelated with anhydrous ethanol to obtain a peptide–chelate, as well as to initially investigate its chelation mechanism and stability analysis.
2. Materials and methods
2.1 Materials
Fresh sheep bones are collected from Tibetan sheep in Oula Township, Maqu County, Gannan Tibetan Autonomous Prefecture, Gansu Province, China, and stored at −20 °C before use. Papain (800 U mg−1), Pepsin (3000 U mg−1) were purchased from Shanghai Yuanye Biotechnology Co., Ltd. All applied chemicals are of analytical grade. Guangdong provincial microbial culture preservation center provided Lactobacillus bulgaricus.
2.2 Preparation of sheep bone powder
Refer to Tan and make appropriate modifications.18 The sheep bones were thawed and cleaned under flowing water, and the large pieces of minced meat, tendons were removed manually, then the clean bones were cut into small pieces. The prepared bone was soaked in hexane for 24 h to remove fat, the sample/hexane ratio was 1
:
10 (w/v) and the residue was washed with deionized water. Then dried and smashed to undecalcified sheep bone powder. On the basis of the above method, add the step of decalcifying with 0.4 mol L−1 hydrochloric acid solution for 24 hours to obtain decalcified powder.
2.3 Enzymatic hydrolysis of sheep bone powder
According to our previous research, 100 g sheep bone powder sample was accurately weighed and dissolved in 1000 mL of distilled water. Sheep bone powder was initially hydrolyzed with papain (55 °C, 3 h, pH 6.0, 5000 U g−1) followed by pepsin (40 °C, 3 h, pH 2.5, 5300 U g−1). Following hydrolysis, the mixture was heated at 100 °C for 15 min to inactivate enzymes. Subsequently, the mixture was centrifuged at 6577 g for 10 min at 4 °C and the supernatant was collected.
2.4 Activation and domestication of Lactobacillus strain
Lactobacillus bulgaricus was inoculated into liquid MRS medium (121 °C, 15 min sterilization) at 1% dose, activated 3 generations, the number of bacteria can reach 107–108 CFU mL−1. The test gradually increase the proportion of enzymatic hydrolyzate in liquid MRS medium for the domestication of strains. MRS: enzymatic hydrolysate volume ratio is 9
:
1, 8
:
2, 7
:
3, 6
:
4, 5
:
5, 4
:
6, 3
:
7, 2
:
8, 1
:
9, 0
:
10. The inoculum size was controlled in 1% and cultured at 37 °C for 30 h.
2.5 Lactobacillus fermentation test of hydrolyzed products
The domesticated Lactobacillus strain was inoculated into the enzymatic hydrolysate to study the calcium ion content. Factor design: amount of glucose (1%, 2%, 3%, 4%, 5%), initial pH value (5.5, 6.0, 6.5, 7.0, 7.5), culture time (6, 12, 18, 24, 30 h) and inoculum size (3%, 4%, 5%, 6%, 7%). In addition to the variables in the experiment, the other factors were as follows: glucose was 3%, inoculum size was 4%, culture temperature was 37 °C, initial pH value was 6.5, culture time was 24 h. The response surface was carried out on the basis of single factor test.
2.6 Preparation experiment of collagen polypeptide and peptide–calcium chelate
Sheep bone was decalcified in 0.4 mol L−1 hydrochloric acid solution for 24 hours before crushing, then sheep bone powder was subjected to enzymatic hydrolysis and fermentation methods to obtain fermentation broth, and finally collagen polypeptide powder was obtained by vacuum freeze drying. Calcium residue was less than 0.5% in decalcified powder samples.
The reaction was placed in a controlled water bath with constant at 37 °C for 15 min after the pH of the solution was adjusted to 7.0 by the addition of 0.1 mol L−1 NaOH. The fermented liquid was added dropwise to anhydrous ethanol (volume ratio 1
:
8), and left to stand for 1 h at room temperature, then centrifuged at 5036 g for 15 min. The precipitate was collected and eluted three times with anhydrous ethanol. The precipitate was freeze-dried to obtain the peptide–calcium chelate powder.
2.7 Ultraviolet-visible absorption spectroscopy analysis
The peptide and peptide–calcium chelate were dissolved in deionized water (1 mg mL−1), respectively. The spectra were recorded by UV spectra using a UV722S (PC) spectrophotometer (Shanghai precision scientific instrument co., Ltd) with the wavelength range of 190–400 nm. Before the measurement of samples, blank calibration of the UV-visible spectrophotometer was done with deionized water.
2.8 FTIR spectroscopy analysis
Collagen peptide and peptide–calcium chelate respectively were mixed with 10 mg of dry KBr, ground and loaded on the FTIR instrument (Nicolet-200SXV, Nicolet, USA). Each sample was scanned from 4000 to 500 cm−1. Spectra were acquired using 64 scans at a resolution of 2 cm−1.
2.9 Differential scanning calorimeter analysis
The thermal transformation properties of samples were measured by differential scanning calorimeter. The sample powder was weighed to about 2.0 mg and sealed in aluminum plate. The scanning was conducted at the heating rate of 10 °C min−1 from 20 °C to 300 °C. The empty plate was used as reference.
2.10 X-ray diffraction analysis
The crystal structures of collagen peptide and peptide–calcium chelate were characterized by X-ray diffract meter (Shimadzu, XRD-6000, Japan). The test conditions were as follows: voltage 40 kV; current 40 mA; scanning speed 2° min−1; scanning step size 0.06°; scanning method: continuous.
2.11 Analysis of amino acids
The collagen peptide and peptide–calcium chelate samples were hydrolyzed with 6 mol L−1 HCl, at 110 °C for 24 h. Then the sample solution was extracted with 0.22 μm microporous membrane and analyzed by automatic amino acid analyzer according to a pre-established method by Yang et al.19
2.12 Scanning electron microscopy analysis
The microstructure of collagen peptide or peptide–calcium chelate was analyzed by a scanning electron microscope (JEOL JSM-6390LV, Tokyo, Japan). A suitable amount of the peptide and the peptide–calcium chelate powder sample were uniformly applied to the sample plate, sprayed with a gold plating film, and the image was acquired at a magnification of 5000 times.
2.13 Stability of peptide–calcium chelate
Stability of peptide–calcium chelate to temperature and in vitro simulated digestion was evaluated according to the method of Liu et al.20 The peptide–calcium chelate was dissolved in deionized water at a final concentration of 2 mg mL−1, and the solution was heated for 3 h at various temperatures (30–80 °C). The stability against in vitro pepsin was assessed by treating 2 mg mL−1 of peptide–calcium chelate solution in 0.1 mol L−1 HCl–KCl buffer (pH 2.0), with 2% (w/w) pepsin for 3 h in a water bath at 37 °C. The reaction was stopped by boiling for 10 min. For stability during digestion with trypsin, 2 mg mL−1 peptide–calcium chelate solution in 0.1 mol L−1 KH2PO4–NaOH buffer (pH = 7.5) was digested with 2% (w/w) trypsin for 3 h in a water bath at 37 °C. The reaction was stopped by boiling for 10 min. For stability against in vitro pepsin digestion and further trypsin digestion, 2 mg mL−1 peptide–calcium chelate solution was first digested by 2% (w/w) pepsin for 3 h and adjusted to pH 7.5 with the addition of 1 mol L−1 NaOH solution. The solution was digested further by 2% (w/w) trypsin at 37 °C for 3 h. Digestion is terminated by deactivating the enzyme by heating the solution at 100 °C for 10 minutes. After digestion, calcium content in chelate was determined by atomic absorption spectrophotometer. The stability of the peptide–calcium chelate was expressed as calcium retention.
2.14 Statistical analysis
Response surface test design and result analysis were carried out by means of designer-expert 8.0.6. IBM SPSS statistics 22.0 software was used for statistical analysis, and Origin 8.5 software was used for drawing.
3. Results and discussion
3.1 Single factor test results
As can be seen from Fig. 1, with the increase of glucose addition amount, inoculum size, initial pH value and fermentation time, the free calcium content first increases and then decreases. When the amount of glucose added is 3%, the free calcium content reaches the maximum value of 2138.234 mg/100 g (Fig. 1A). According to Fig. 1B, when the inoculum size is 6%, the free calcium content reaches a maximum value of 2191.121 mg/100 g. However, the size of inoculum continued to increase, the free calcium content decreased. It may be due to the limitation of the nutrient composition of the medium, which could not supply more nutrients to the microorganisms, and the growth of the microorganisms is restricted, so the free calcium content is decreased. In addition, considering the factors of excessive size of inoculum on the material consumption of fermentation system and the influence of autolysis of bacteria, 6% is determined as the appropriate inoculum size. Fig. 1C shows that at the beginning of fermentation pH 6.5, the free calcium content reaches the maximum value of 2135.531 mg/100 g. Fig. 1D shows that when the fermentation time is 24 h, the free calcium content reaches the maximum value of 2201.898 mg/100 g. After that, the free calcium content decreases due to the decrease of nutrients such as carbon source and nitrogen source in the fermentation liquid and the decay of the bacteria. The optimal glucose addition amount, inoculum size, initial pH value and fermentation time obtained by single factor test are 3%, 6%, 6.5 and 24 h, respectively.
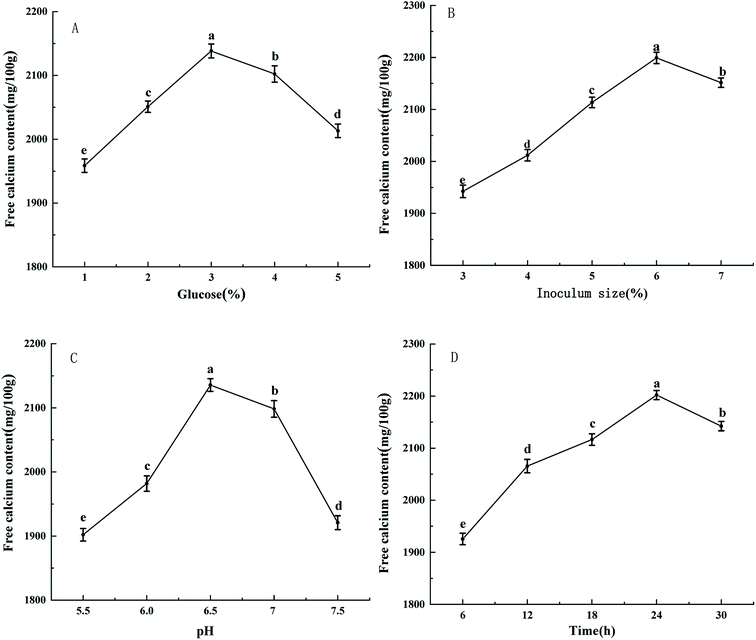 |
| Fig. 1 Effects of glucose (A), inoculum size (B), pH (C), time (D) on free calcium content. Small letters indicate significant differences within the group (P < 0.05). | |
3.2 Response surface optimization result
Table 1 shows the design scheme and results of the Box–Behnken center combination test by using the design-expert 8.0 Design software.
Table 1 Response surface design and results
Run number |
A, Time (h) |
B, Glucose (%) |
C, pH |
D, Inoculum size (%) |
Free calcium (mg/100 g) |
1 |
18 |
2 |
6.5 |
6 |
1917.68 |
2 |
30 |
2 |
6.5 |
6 |
1887.43 |
3 |
18 |
4 |
6.5 |
6 |
1887.82 |
4 |
30 |
4 |
6.5 |
6 |
2025.46 |
5 |
24 |
3 |
6 |
5 |
1864.44 |
6 |
24 |
3 |
7 |
5 |
1923.05 |
7 |
24 |
3 |
6 |
7 |
1986.61 |
8 |
24 |
3 |
7 |
7 |
1957.53 |
9 |
18 |
3 |
6.5 |
5 |
1943.43 |
10 |
30 |
3 |
6.5 |
5 |
2033.01 |
11 |
18 |
3 |
6.5 |
7 |
1986.62 |
12 |
30 |
3 |
6.5 |
7 |
1994.01 |
13 |
24 |
2 |
6 |
6 |
1845.12 |
14 |
24 |
4 |
6 |
6 |
1898.96 |
15 |
24 |
2 |
7 |
6 |
1871.32 |
16 |
24 |
4 |
7 |
6 |
1913.46 |
17 |
18 |
3 |
6 |
6 |
1851.47 |
18 |
30 |
3 |
6 |
6 |
1982.51 |
19 |
18 |
3 |
7 |
6 |
2008.46 |
20 |
30 |
3 |
7 |
6 |
1895.62 |
21 |
24 |
2 |
6.5 |
5 |
1888.62 |
22 |
24 |
4 |
6.5 |
5 |
1862.21 |
23 |
24 |
2 |
6.5 |
7 |
1856.12 |
24 |
24 |
4 |
6.5 |
7 |
1916.82 |
25 |
24 |
3 |
6.5 |
6 |
2181.6 |
26 |
24 |
3 |
6.5 |
6 |
2199.64 |
27 |
24 |
3 |
6.5 |
6 |
2191.84 |
28 |
24 |
3 |
6.5 |
6 |
2231.61 |
29 |
24 |
3 |
6.5 |
6 |
2218.11 |
The fermentation process parameters were optimized by time, glucose, pH value and inoculum size to obtain the quadratic regression equation.
R2 = 2204.56 − 18.55A − 19.87B + 11.69C + 15.25D + 41.97AB − 60.97AC − 20.55AD − 2.93BC + 21.78BD − 21.92CD − 100.5A2 − 180.81B2 − 152.37C2 − 125.63D2 |
Meanwhile, the significance test of variance analysis was carried out (Table 2).
Table 2 Analysis of variance for the quadratic regression model
Sources |
Sum of squares |
DF |
Mean square |
F-value |
P-value |
Significant |
Model |
3.93 × 105 |
14 |
28 074.5 |
36.01 |
<0.0001 |
** |
A Time |
4127.41 |
1 |
4127.41 |
5.29 |
0.0373 |
* |
B Glucose |
4738.48 |
1 |
4738.48 |
6.08 |
0.0272 |
* |
C pH |
1640.67 |
1 |
1640.67 |
2.1 |
0.1689 |
|
D Inoculum size |
2789.1 |
1 |
2789.1 |
3.58 |
0.0795 |
|
AB |
7046.51 |
1 |
7046.51 |
9.04 |
0.0094 |
** |
AC |
14 869 |
1 |
14 869 |
19.07 |
0.0006 |
** |
AD |
1688.76 |
1 |
1688.76 |
2.17 |
0.1632 |
|
BC |
34.23 |
1 |
34.23 |
0.044 |
0.837 |
|
BD |
1896.86 |
1 |
1896.86 |
2.43 |
0.1411 |
|
CD |
1922.34 |
1 |
1922.34 |
2.47 |
0.1387 |
|
A2 |
65 508.82 |
1 |
65 508.82 |
84.01 |
<0.0001 |
** |
B2 |
2.12 × 105 |
1 |
2.12 × 105 |
271.96 |
<0.0001 |
** |
C2 |
1.51 × 105 |
1 |
1.51 × 105 |
193.13 |
<0.0001 |
** |
D2 |
1.02 × 105 |
1 |
1.02 × 105 |
131.29 |
<0.0001 |
** |
Residual |
10 916.25 |
14 |
779.73 |
|
|
|
Lack of fit |
9287.67 |
10 |
928.77 |
2.28 |
0.2216 |
|
Pure error |
1628.58 |
4 |
407.14 |
|
|
|
Total |
4.04 × 105 |
28 |
|
|
|
|
Std. dev. |
27.92 |
|
|
R-Squared |
0.9730 |
|
C.V.% |
1.42 |
|
|
Adj R-squared |
0.9460 |
|
Analysis of variance is implemented to validate the model accuracy. The quadratic regression model selected by the test is highly significant (P < 0.0001), indicating that there is a significant linear relationship between the dependent variable and the independent variable of the regression equation. The lack of fit test having p-value 0.2216 greater than 0.01 suggested that lack of fit is not significant. Adjusted-R2 with 0.9460 reveals 94.60% of the change in response value can be explained by the model. R2 with 0.9730 indicates close agreement between the predicted and experimental values. A low value of the coefficient of the variation (C.V., 1.42%), indicates a high degree of precision and a good deal of reliability with the experimental values. The model satisfactorily fitted to the experimental data and accounted all the contribution in the regression response relationship.21
The optimal technological parameters of Lactobacillus fermentation obtained by this model were: glucose 3.07%, time 24.58 h, pH 6.51, inoculum size 6.06%, and the free calcium content reached 2206.67 mg/100 g. In the validation test, considering the feasibility of operation, the optimal process parameters were adjusted as glucose 3%, time 24.5 h, pH 6.50, inoculum size 6%, 3 times in parallel, and the free calcium content was 2212.58 mg/100 g. The calcium-chelating capacity was 42.57 ± 0.09%. Atlantic salmon (Salmo salar L.) bones were used to prepare salmon ossein oligopeptides (SOOP). The calcium-chelating capacity of SOOP–Ca was 52.47 ± 0.08%. Pig bones were used to prepare collagen peptide–calcium chelate with calcium chelating rate of 78.38%.22 These results are higher than that of this experiment, which may be related to the properties of raw materials and purification process. The ability of a casein hydrolysate rich in casein phosphopeptides (CPPs) to form calcium complexes was studied by Recio et al., which was similar to the study in this study.23 The calcium content of the calcium peptide complex is 15.81%. The peptide content of the calcium peptide complex is 74.25%.
3.3 Ultraviolet and FTIR spectroscopy analysis
As seen in Fig. 2A, ultraviolet spectroscopy is an analytical method that can be used to study changes in matter and determine whether new substances are produced.24 The complexes formed by metal ions and organic ligands may lead to the emergence of new absorption peaks or the displacement/disappearance of the original absorption peaks.25 The UV-visible spectrum of collagen peptide–calcium chelate showed a distinct difference from collagen peptide. The specific carboxyl groups, amide bonds and carbonyl groups in the peptide observed a maximum absorption peak near 210 nm in the spectrum of the collagen peptide.26 When the peptide chelates with calcium, the maximum absorption peak moves to short wavelength as a whole. Ca2+ forms the complex bond with N and O in the peptide, which affects the C
O and –NH2 electronic transition on the peptide bond.27
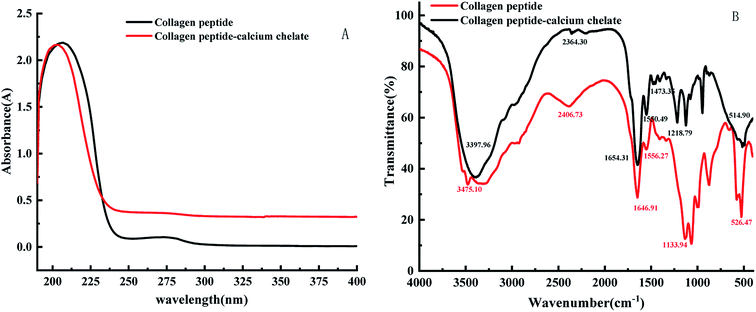 |
| Fig. 2 Ultraviolet (A) and FTIR (B) spectra of collagen peptide and peptide–calcium chelate. | |
The characteristic change of FTIR absorption peak of calcium-chelating peptides were useful evidence to reflect the interactions between metal ions and organic ligand groups in peptides.28–30 As shown in Fig. 2B, the FTIR spectrum of peptide was dramatically changed after adding calcium ions. The changes of FTIR absorption peaks of carboxylates and amide compounds could reflect the interaction between calcium ions and peptides.31,32 After the sheep collagen peptide chelated calcium, specifically speaking, the wave number shifted from 3397.96 cm−1 to 3475.10 cm−1, might be caused by collagen peptide was coordinated to Ca2+ inducing a stretch and hydrogen bonds replaced. The peak of the amide-I band (C
O stretching vibration) decreased from 1654.31 cm−1 to 1646.91 cm−1, which were associated with the stretching vibrations of the carbonyl group (C
O) of the amide (peptide bond). It indicates that the carboxylate group was involved in the formation of the peptide–calcium complex. At the same time, the peak of the amide-II band (N–H bending) increased from 1550.49 cm−1 to 1556.27 cm−1, indicating that the amino group of the peptide contributed to the formation of the peptide–calcium chelate. In addition, the 1473.35 cm−1 peak which representing the symmetric stretching mode of –COOC– has disappeared in the FTIR spectrum of peptide–calcium chelate. It suggested that the carboxyl groups of peptides have bound with Ca2+, and then turned into the calcium-chelating form (–COO–Ca).33 When a metal ion interacts with only one oxygen atom of a –COO– group, the coordination structure is regarded as unidentate. In the bidentate coordination mode, the metal ion interacts equally with the two oxygen atoms of a –COO– group. In the bridging coordination mode, one metal ion binds to one of the two oxygens in a –COO– group and another metal ion to the other oxygen atom.34 The absorption band at 1218.79 cm−1 corresponds to the amide-III band in the peptide, which chelates with calcium ions and then moves to the lower wave number 1133.94 cm−1, indicating that calcium ions could bind to the peptide and cause some changes in secondary structure. To sum up, it was inferred that the principal binding sites of sheep collagen peptide were the carboxyl oxygen, hydroxyl oxygen, amino nitrogen and oxygen of the peptide bonds, these groups were involved in coordination with Ca2+ to form the peptide–calcium chelates. Similar changes in the FTIR spectra were found in pacific cod bone peptide–calcium complex.35
3.4 Differential scanning calorimeter and X-ray diffraction analysis
As seen in Fig. 3A, the endothermic peaks of the collagen peptide were 107.4 °C and 132.7 °C, respectively. The endothermic peaks of the peptide–calcium chelate were 150.4 °C and 184.1 °C, respectively. When the collagen peptide chelates the calcium ions, the thermal denaturation temperature increases as a whole. The reason is that the structure of chelate is more stable than collagen peptide, the energy of chemical bond is larger, and more energy is required to break, so the breaking temperature is increased. Different endothermic peaks are caused by C–N bond that breaked at high temperatures. The C–N bond is located at different positions in collagen polypeptide and calcium peptide chelate, so the energy and temperature required for fracture are different, and the exact location of absorption peak is determined through software analysis. The change in DSC also indicates that peptide–calcium chelate is a substance different from peptide.
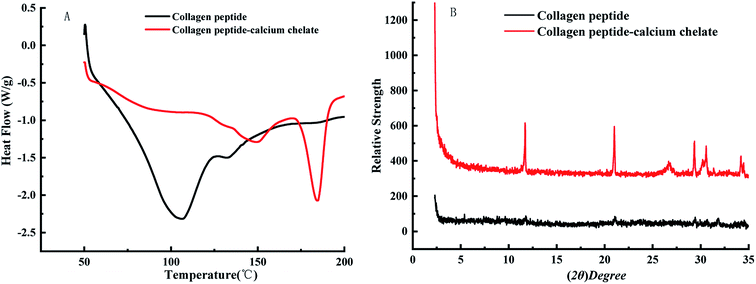 |
| Fig. 3 DSC (A) and X-ray (B) diffraction diagram of collagen peptide and peptide–calcium chelate. | |
As can be seen from the Fig. 3B, there is no strong diffraction peak in the collagen peptide, indicating that the collagen protein after enzymolysis-fermentation has been degraded to a large extent, and the collagen peptide basically presents an irregular amorphous form. After the peptide chelated with calcium, the pattern changed significantly. It indicates that the collagen peptide binds to calcium and produces a new interaction force in the spatial structure, which leads to the emergence of new diffraction peaks, indicating that the collagen peptide chelate with calcium.
3.5 Amino acid composition
The amino acid compositions of collagen peptide and peptide–calcium chelate are listed in Table 3. Peptide–calcium chelate was rich in Ala, Glu, Asp and Gly, and low in Phe, His, and Ser, which was similar to that reported by Bae et al.36 The contents of Glu, Asp and Gly in collagen peptide were 7.79%, 6.45%, 20.31%, respectively. After chelating calcium, the contents of these amino acids in peptide–calcium chelate were 14.76%, 10.85%, 12.64%, respectively. These results indicated that the calcium-chelating capacity of acidic amino acids, Glu acid and Asp acid was better than that of other amino acids. This may be due to the carboxyl groups of Glu and Asp residues on their side chain which might exert a stronger calcium-chelating capacity during the reaction, which was similar to that reported by soybean peptides.37 It can be seen from Table 3 that with the decrease of Gly content, the molecular weight of the prepared collagen polypeptide will also decrease, but whether it will lead to the deterioration of calcium chelating ability needs further confirmation. Studies have shown that the molecular weight of collagen polypeptide is related to the calcium chelating ability, but the specific variation of different raw materials is different. The chelating ability of collagen polypeptide with larger molecular weight is not necessarily strong. Jiang showed that when the phosphopeptide fragment is less than 1 kDa, it does not bind calcium to any significant degree, while 1–3 kDa phosphopeptide fragments have a higher ability to bind soluble calcium.38 Bao et al. studied the calcium binding capacity of soybean proteolytic hydrolysates (SPHs), and the results showed that the peptide fractions exhibiting high Ca binding capacity had average molecular weights of either 14.4 kDa or 8 to 9 kDa.39 Therefore, the calcium binding ability of sheep bone collagen is not only related to the structure of amino acid residues, but also related to the molecular weight. However, the specific relationship needs further study.40
Table 3 Amino acid composition of collagen peptide and peptide–calcium chelate (%, w/w)
Amino acid |
Collagen peptide |
Peptide–calcium chelate |
Lys |
4.92 |
2.94 |
Ala |
12.87 |
11.45 |
Cys |
0.45 |
1.78 |
Val |
2.26 |
1.65 |
Glu |
7.79 |
14.76 |
Ile |
1.50 |
1.34 |
Asp |
6.45 |
10.85 |
Leu |
5.13 |
2.25 |
Tyr |
2.19 |
1.28 |
Phe |
1.57 |
1.05 |
His |
1.83 |
0.89 |
Arg |
4.92 |
1.87 |
Pro |
6.53 |
7.52 |
Ser |
3.67 |
0.98 |
Met |
2.02 |
1.20 |
Gly |
20.31 |
12.64 |
Thr |
2.56 |
2.41 |
Total |
86.97 |
75.66 |
3.6 Scanning electron microscope
As shown in the Fig. 4, it can be seen that the surface of the collagen peptide is smooth with small cracks on the surface, which may be generated during the rapid vacuum freeze-drying process of fermentation liquid. However, the surface of peptide–calcium chelate was more roughly, loosely, and with many spherical aggregates. During the chelation process, the initial structure of the peptide is altered due to the interaction between the peptide and the calcium ions.
 |
| Fig. 4 Scanning electron microscope of collagen peptide (left) and peptide–calcium chelate (right). | |
3.7 Stability of peptide–calcium chelate
The stability of the peptide–calcium chelate at different temperatures and digestion with various proteases were shown in Fig. 5. It is essential to study the stability of the peptide–calcium chelate in the human gastrointestinal environment as calcium ions tend to react with phytic acid or oxalic acid in the stomach to produce insoluble precipitation, or form Ca(OH)2 in the small intestine, resulting in low bioavailability of calcium.41 Therefore, the tolerance of bioactive substances to pepsin and trypsin can be used to evaluate their digestive stability. As temperature increased, the calcium retention of peptide–calcium chelate decreased slightly, but all remained above 80%, which indicated that peptide–calcium chelate was relatively stable at a wide range of temperatures. After being digested with different proteases, the calcium content of peptide–calcium chelate decreased (p < 0.05) to 87.3 ± 2.3%, 73.5 ± 4.2% and 57.6 ± 3.8%, respectively, relative to the control. The results indicated that the peptide–calcium chelate was stable in the gastrointestinal environment, which could possibly promote calcium absorption.
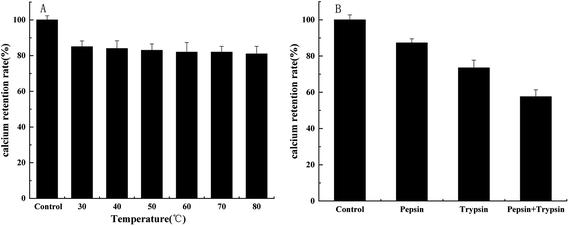 |
| Fig. 5 Stability of peptide–calcium chelate after 3 h incubation at various temperatures (A) and digestion with various proteases (B). | |
4. Conclusions
Sheep bone powder, as raw material, was used to produce peptide–calcium chelate by enzymolysis-fermentation methodology. The calcium-chelating capacity was 42.57 ± 0.09%. All the results of UV-vis absorption spectroscopy, FT-IR spectroscopy, differential scanning calorimeter, X-ray diffraction, amino acid analysis and SEM showed that the structure of peptide–calcium chelate was different from that of peptides, and calcium bound to peptides mainly by interacting with the amino nitrogen atoms and the oxygen atoms of carboxylic acid groups. The findings also demonstrated that peptide–calcium chelate was stable and maintained certain resistance against temperature and in vitro digestion. Based on these results, peptide–calcium chelate could be used as a novel calcium supplement to improve calcium absorption. This study provides a practical way to utilize sheep bone. However, further researches are required to focus on the gastrointestinal tract and the exact absorption mechanism in vivo of sheep bone peptide–calcium chelate.
Conflicts of interest
The authors declare there are no conflicts of interest regarding the publication of this paper.
Acknowledgements
This work was financially supported by the China-Malaysia Halal Food and Related Industries International Innovation Cooperation (1504WKCA094); China-Malaysia Cooperative Study on Biotechnology and Standard Systems in Halal Foods (17YF1WA166); Development of Essential Oil Preservative and Its Application to Gannan Tibetan Mutton Preservation (2019B-077); Basic Business Project of Colleges and Universities of Finance Department of Gansu Province (1011JKCA179).
References
- J. Pagán, A. Ibarz, V. Falguera, D. Fabrizio, F. Amelia and C. Laura, et al., Enzymatic hydrolysis kinetics and nitrogen recovery in the protein hydrolysate production from pig bones, J. Food Eng., 2013, 119(3), 655–659 CrossRef.
- Y. Tian, Potential assessment on biogas production by using livestock manure of large-scale farm in China, J. Agric. Food Chem., 2012, 28(8), 230–234 Search PubMed.
- P. Zhan, H. Tian, X. Zhang and L. Wang, Contribution to aroma characteristics of mutton process flavor from the enzymatic hydrolysate of sheep bone protein assessed by descriptive sensory analysis and gas chromatography olfactometry, J. Chromatogr., 2013, 921–922(6), 1–8 CrossRef CAS PubMed.
- H. Yang, Y. Liu, L. Ma and B. Kong, Hydrolyzing Condition and Immunocompetence of Sheep Bone Protein Enzymatic Lysates, Agric. Sci. China, 2009, 8(11), 1332–1338 CrossRef.
- W. Daengprok, W. Garnjanagoonchorn, O. Naivikul, P. Pornsinlpatip, K. Issigonis and Y. Mine, et al., Chicken Eggshell Matrix Proteins Enhance Calcium Transport in the Human Intestinal Epithelial Cells, Caco-2, J. Agric. Food Chem., 2003, 51(20), 6056–6061 CrossRef CAS PubMed.
- J. K. Bass and G. M. Chan, Calcium nutrition and metabolism during infancy, Nutrition, 2006, 22(10), 1057–1066 CrossRef CAS PubMed.
- Y. Mine and F. Shahidi, Nutraceutical Proteins and Peptides in Health and Disease, CRC Press, 2006, vol. 56, (1), pp. 96–108 Search PubMed.
- T. Hou, Y. Liu, D. Guo, B. Li and H. He, Collagen peptides from crucian skin improve calcium bioavailability and structure characterization by HPLC-ESI-MS/MS, J. Agric. Food Chem., 2017, 34(13), 74–83 Search PubMed.
- V. Centeno, G. Barboza, A. Marchionatti, R. Valeria and T. Nori, et al., Molecular mechanisms triggered by low-calcium diets, Nutr. Rev., 2009, 22(02), 163 CrossRef CAS PubMed.
- S. Osborne and A. Ellington, Incorporating disulfide cross-links at the terminus of oligonucleotides via solid-phase nucleic acid synthesis, Cheminform, 2010, 28(6), 278–286 CrossRef.
- L. Guo, H. Hou, B. Li, Z. Zhang, S. Wang and X. Zhao, Preparation, isolation and identification of iron-chelating peptides derived from Alaska pollock skin, Process Biochem., 2013, 48(5–6), 988–993 CrossRef CAS.
- D. Straub, Calcium Supplementation in Clinical Practice: A Review of Forms, Doses, and Indications, Nutr. Clin. Pract., 2007, 22(3), 286–296 CrossRef PubMed.
- M. Vavrusova and L. Skibsted, Calcium nutrition Bioavailability and fortification, LWT--Food Sci. Technol., 2014, 59(2), 1198–1204 CrossRef CAS.
- D. Straub, Calcium Supplementation in Clinical Practice: A Review of Forms, Doses, and Indications, Nutr. Clin. Pract., 2007, 22(3), 286–296 CrossRef PubMed.
- N. Sun, H. Wu, M. Du, Y. Tang, H. Liu and Y. Fu, et al., Food protein-derived calcium chelating peptides: a review, Trends Biochem. Sci., 2016, 34(4), 67–74 Search PubMed.
- A. Rerat, N. Simoes, F. Mendy and L. Roger, Amino acid absorption and production of pancreatic hormones in non-anaesthetized pigs after duodenal infusions of a milk enzymic hydrolysate or of free amino acids, Br. J. Pharmacol., 1988, 60(1), 16 Search PubMed.
- S. Perego, F. Del, L. De, D. Fabrizio, F. Amelia and C. Laura, et al., Calcium bioaccessibility and uptake by human intestinal like cells following in vitro digestion of casein phosphopeptide-calcium aggregates, Food Funct., 2015, 6(6), 1796–1807 RSC.
- X. Tan, L. Qi, F. Fan, Z. Guo, Z. Wang and W. Song, et al., Analysis of volatile compounds and nutritional properties of enzymatic hydrolysate of protein from cod bone, Food Chem., 2018, 264(4), 350–357 CrossRef CAS PubMed.
- Y. Yang, G. Tao, P. Liu and J. Liu, Peptide with Angiotensin I-Converting Enzyme Inhibitory Activity from Hydrolyzed Corn Gluten Meal, J. Agric. Food Chem., 2007, 55(19), 7891–7895 CrossRef CAS PubMed.
- W. Liu, J. Lu, F. Gao, R. Gu, F. Lin and D. Ren, et al., Preparation, characterization and identification of calcium-chelating Atlantic salmon (Salmo salarL.) ossein oligopeptides, Eur. Food Res. Technol., 2015, 241(6), 851–860 CrossRef CAS.
- L. Tiago, M. Francislene and M. Naise, Optimization of the protein extraction method of goat meat using factorial design and response surface methodology, Food Chem., 2018, 134(3), 1267–1278 Search PubMed.
- W. Wu, L. He, Y. Liang, L. Yue, W. Peng and G. Jin, et al., Preparation process optimization of pig bone collagen peptide-calcium chelate using response surface methodology and its structural characterization and stability analysis, Food Chem., 2019, 284(30), 80–89 CrossRef CAS PubMed.
- R. T. Recio, N. P. Gueera and A. Torrado, Interaction between calcium and casein hydrolysates: stoichiometry, binding constant, binding sites and thermal stability of casein phosphopeptide complexes, Int. J. Biochem., 2019, 88(7), 25–33 CAS.
- D. Blat, L. Weiner, M. Youdim and M. Fridkin, A Novel Iron-Chelating Derivative of the Neuroprotective Peptide NAPVSIPQ Shows Superior Antioxidant and Antineurodegenerative Capabilities, J. Med. Chem., 2008, 51(1), 126–134 CrossRef CAS PubMed.
- N. Sun, P. Cui and S. Lin, Characterization of sea cucumber (stichopus japonicus) ovum hydrolysates: calcium chelation, solubility, and absorption into intestinal epithelial cells, J. Sci. Food Agric., 2017, 97(13), 76–86 Search PubMed.
- D. Chen, Z. Liu, W. Huang, Y. Zhao, S. Dong and M. Zeng, Purification and characterisation of a zinc-binding peptide from oyster protein hydrolysate, J. Funct. Foods, 2013, 5(2), 689–697 CrossRef CAS.
- L. Zhao, Q. Huang, S. Huang, J. Lin, S. Wang and Y. Huang, et al., Novel Peptide with a Specific Calcium-Binding Capacity from Whey Protein Hydrolysate and the Possible Chelating Mode, J. Agric. Food Chem., 2014, 62(42), 10274–10282 CrossRef CAS PubMed.
- M. Nara, H. Morii and M. Tanokura, Coordination to divalent cations by calcium-binding proteins studied by FTIR spectroscopy, Biophys. Chem., 2013, 1828(10), 2319–2327 CAS.
- M. Nara and M. Tanokura, Infrared spectroscopic study of the metal-coordination structures of calcium-binding proteins, Biochem. Biophys. Res. Commun., 2008, 369(1), 239 CrossRef PubMed.
- D. Chen, X. Mu, H. Huang, R. Nie, Z. Liu and M. Zeng, Isolation of a calcium-binding peptide from tilapia scale protein hydrolysate and its calcium bioavailability in rats, J. Funct. Foods, 2014, 6(13), 575–584 CrossRef CAS.
- Z. Zhang, Z. Han, X. A. Zeng and M. S. Wang, The preparation of Fe-glycine complexes by a novel method (pulsed electric fields), Food Chem., 2017, 219(43), 468–476 CrossRef CAS PubMed.
- L. Zhao, S. Huang, X. Cai, J. Hong and S. Wang, A specific peptide with calcium chelating capacity isolated from whey protein hydrolysate, J. Funct. Foods, 2014, 10(5), 46–53 CrossRef CAS.
- X. X. Cai, Q. Yang, J. Li, N. Fang and S. Wu, A Specific Peptide with Calcium-Binding Capacity from Defatted Schizochytrium sp. Protein Hydrolysates and the Molecular Properties, Mol. Cell. Biochem., 2017, 22(4), 544 Search PubMed.
- G. B. Deacon and R. J. Phillips, Relationships between the carbon-oxygen stretching frequencies of carboxylato complexes and the type of carboxylate coordination, Coord. Chem. Rev., 2008, 33(3), 227–250 CrossRef.
- Z. Peng, H. Hou, K. Zhang and B. Li, Effect of calcium-binding peptide from Pacific cod (Gadus macrocephalus) bone on calcium bioavailability in rats, Food Chem., 2017, 221(43), 373–378 CrossRef CAS PubMed.
- I. Bae, K. Osatomi, A. Yoshida, K. Osako, A. Yamaguchi and K. Hara, Biochemical properties of acid-soluble collagens extracted from the skins of underutilised fishes, Food Chem., 2008, 108(1), 49–54 CrossRef CAS.
- H. Kumagai, A. Koizumi and N. Sato, Effect of phytate-removal and deamidation of soybean proteins on calcium absorption in the in situ rats, Biofactors, 2010, 22(1–4), 21–24 Search PubMed.
- B. Jiang and Y. Mine, Phosphopeptides Derived from Hen Egg Yolk Phosvitin: Effect of Molecular Size on the Calcium-binding Properties, J. Agric. Food Chem., 2001, 65(5), 1187–1190 CAS.
- X. L. Bao, Y. Lv, B. Yang, C. Ren and S. Guo, A Study of the Soluble Complexes Formed during Calcium Binding by Soybean Protein Hydrolysates, J. Food Sci., 2008, 73(3), C117–C121 CrossRef CAS PubMed.
- X. Wang, A. Gao, Y. Chen, X. Zhang, S. Li and Y. Chen, Preparation of cucumber seed peptide-calcium chelate by liquid state fermentation and its characterization, Food Chem., 2017, 229(35), 487–494 CrossRef CAS PubMed.
- M. Vavrusova and L. Skibsted, Calcium nutrition bioavailability and fortification, LWT--Food Sci. Technol., 2014, 59(2), 1198–1204 CrossRef CAS.
|
This journal is © The Royal Society of Chemistry 2020 |