DOI:
10.1039/D0RA00417K
(Paper)
RSC Adv., 2020,
10, 14965-14971
Reaction-diffusion model as framework for understanding the role of riboflavin in “eye defence” formulations
Received
14th January 2020
, Accepted 4th April 2020
First published on 16th April 2020
Abstract
Analysis of UV-visible spectra, performed on commercial riboflavin-based eye drops, showed that absorbance is a saturating function of vitamin concentration. This implies a threshold concentration, Ct, such that for riboflavin concentration > Ct the absorbance remains constant and the effectiveness of the eye drops is independent of the dose used. These experimental results were combined with a diffusion–reaction model to elucidate the mechanism of action within the cornea. The model predicts that the eye drops have a low effectiveness on UVB and UVC, while they have a good performance for UVA. Indeed, at the center of the cornea the transmittance is significantly reduced and after 1 h it is reduced by about 70% compared to a cornea devoid of eye drops.
1 Introduction
Ultraviolet (UV) radiation is the part of the electromagnetic spectrum located between X-radiation and visible light. Based on physiological effects it is divided into three primary regions. UV-A 315–400 nm, UV-B 315–280 nm and UV-C 280–100 nm. The ability of UV rays to penetrate biological tissues increases with decreasing wavelength. A prolonged exposure of the eyes to UV radiation may cause acute, or chronic, effects on the cornea, lens and retina. The World Health Organization estimates that about 20% of the 12–15 million people who every year risk blindness is caused by sun exposure. Most of UV radiation incident on the eyes is absorbed by cornea and lens, while only 4% reaches the retina. Awareness of UV radiation damage to the eyes has risen substantially over recent years, this has encouraged pharmaceutical companies to look for formulations protecting stressed ocular tissues. Due their easy use, eye drops are generally the preferred means to apply medications when treating ocular disorders.1
It is well-known that aqueous solutions of Riboflavin (RBF) (vitamin B2) protects the eye against UV radiation. However, the outermost epithelium, an hydrophobic layer of 50–100 μm, is impermeable to hydrophilic drugs, so that 90% of the instilled dose is lost almost immediately. In order to increase the corneal permeability, compounds such as hyaluronic acid, D-α-tocopheryl polyethylene glycol 1000 succinate, L-proline, methyl sulfonyl methane and many other additives are added to increase the formulation hydrophobicity.2 The resulting chemical complexity of the formulation makes difficult to understand the mechanism of action of riboflavin in corneal tissue. Furthermore, RBF is degraded by light and lumichrome is formed by UVA rays or blue light under physiologically neutral conditions.3 From what has been said about UV protection by RBF it is clear that the elucidation of the basic mechanism can be attained only by studying how the molecular diffusion and RBF oxidation are related to cornea structure and how, in turn, the protection process is related to the physicochemical properties of eye drops. The goal of this study is to clarify how to use experimental results from UV-visible absorption spectra to predict the behavior of the eye, treated with RBF-based eye drops, when it is exposed to UV radiation. We find that UV-spectra provide useful data to be processed in a diffusion–reaction model to predict the amount of RBF present in the cornea and thus to trace tissue-transmittance characteristics. The mathematical complexity inherent in a diffusion–reaction model is overcomes with isolating two regimes of reaction which to a great extent are independent which lead to simple phenomenological equations.4 In this paper absorption spectra of a commercial eye drop are used to elaborate a diffusion–reaction model to evaluate the degree of UVA UVB absorption RBF. Although the model is applied to corneal tissue, it is mathematically presented in a dimensionless form to be extended to other types of RBF administrations a diffusion–reaction model to evaluate the efficacy of RBF in commercial eye drops is discussed. The model is presented in dimensionless form to be applied for different administrations, although in the paper it is applied for the protection of the corneal tissue.
2 Theoretical aspects
2.1 Diffusion model
When a drop of drug-solution is posed on biological tissue, as in the in vivo case, it becomes wetted, triggering a liquid–solid mass transfer process, providing a means for the drug diffusion in the tissue.
At time t = 0 a volume V (typically 0.05 cm3) of Drop Defence containing N molecules of RBF is placed on the cornea, so that a C0 concentration of vitamin B2 is present on its outer surface. Such solution is assumed to be uniform, namely there are no concentration gradients,5 and it remains constant until the next instillation. A rate balance over an element in the cornea gives
|
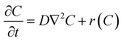 | (1) |
where
D is the diffusion coefficient of RBF in the cornea,
t is the time, ∇
2 is the Laplacian operator. The reaction term,
r(
C) is an empirical expression. In general, the Laplacian operator involves all three spatial coordinates, but we will be concerned mainly with systems where the symmetry allows this diffusion term to be written as a function of a single spatial coordinate. Furthermore, it is experimentally ascertained that UV radiation causes damage even to the retina,
6 that is to say at a distance much greater than the corneal thickness. Therefore, we model the system as a infinite cylinder in which the diffusion in the radial direction may be neglected. Interestingly if oxygen is present in the tissue the oxidative degradation of RBF may take place.
7 The rate of photolysis of RBF molecules depends on state of ionization of the molecule and its susceptibility to excitation and subsequent degradation on exposure to light. This reaction, studied under different experimental conditions, was found to be a pseudo first order kinetics.
8
Thus concentration at position x and time t assumes the form
|
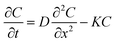 | (2) |
subject to the conditions
|
 | (3) |
which ensure a
C0 concentration of RBF at the outer corneal surface. Both the diffusion coefficient and the first pseudo-first order constant are assumed to be independent of concentration.
9 By applying the Laplace transform
10 method one can calculate the following solution
|
 | (4) |
Depending on the relative rates of diffusion and chemical reaction one of them would control the overall kinetics. Specifically diffusion will control if reaction rate is fast. To delineate these regions, we introduce a diffusion lifetime,11
and a reaction lifetime, tR = 1/K, where
is a characteristic system length. Then we convert eqn (4) to non-dimensional form adopting as unit of length
and time
In these units, with appropriately scaled quantities
|
 | (5) |
concentration profile as an equation containing only one control parameter,
12 
is obtained
|
 | (6) |
2.2 Electromagnetic-radiation absorption
An electromagnetic wave incident on a material undergoes an attenuation and its intensity is reduced by absorption.13 This latter phenomenon is physically described by absorption coefficient, σ, defined as the ratio between the intensity absorbed in the unit volume and the intensity incident per unit area. With reference to a light beam propagating along x direction, the fraction of intensity lost
in the volume element, dV, is |
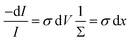 | (7) |
where Σ is the cross section of the beam, I is the impinging intensity and dV = Σdx.
Integrating eqn (7), Lambert–Beer law is obtained
|
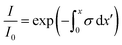 | (8) |
The variation of intensity passing through the volume element, therefore, is in eqn (8) the coefficient σt takes into account the overall attenuation suffered by the radiation, in the considered volume. However, if the system is composed of two or more phases, each individual contributes has to be explained. In our model, phases consist of a liquid solution (eye drops) and a solid medium (cornea) so that absorption coefficient can be rewritten as
where
σl and
σs are liquid and solid absorption contributes, respectively. The solid term depends only the wavelength of the radiation passing through the material and it represents the inverse of the penetration depth,
δp of that specific radiation in the material:
|
 | (10) |
The problem of calculating σl(λ, C) is equivalent to the problem of establishing a reliable equation characterizing the molecular UV absorption; once such an equation exists, the transmittance can be found by straightforward computation. Therefore, to establish the composition dependence of the absorption coefficient it is important that σl(λ, C) is written in terms of parameters with clear physical significance, in order that system properties may be related to experimental results. Thus, we write
|
σl(λ, C) = θl(λ, C)C
| (11) |
where the function
θl(
λ,
C) reflects the nonideality of the system. To relate the function
θl(
λ,
C) to the experimental quantities, one must bear in mind that the molar extinction coefficient,
εl(
λ,
C) is defined in terms of decimal logarithm, therefore
|
θl(λ, C) = ln10 εl(λ, C)
| (12) |
Finally, substitution of eqn (11), (12) and (9) into (8) results in
|
 | (13) |
where
|
 | (14) |
are the spectrophotometrically measured absorbance, Δ
p(
λ) the dimensionless penetration length. In addition, the parameter
s takes into account that in a spectrophotometric measurement the length is measured in cm, while in the diffusion process it is arbitrary.
Eqn (13) establishes that the transmittance of the UV radiation in the cornea can be calculated if RBF concentration profile, cross the ocular tissue, is known.
3 Materials and methods
3.1 Chemicals
Ophthalmic antioxidant formulation, termed drop defence, was kindly offered by Iros sc S.r.l. (Italy), patent no. EP 2459186, USP 9192594). According to the manufacturer drop defence is a mixture of riboflavin (RBF), D-α-tocopheryl polyethylene glycol (TPGS vitamin E), proline, glycine, lysine and leucine solution, pH 7.2–7.4. Phosphate buffered saline (PBS) solution was prepared dissolving one tablet, purchased by Sigma Aldrich, into 0.2 dm3.
3.2 Solutions preparation
Since the detailed composition of the eye drops is patented, the concentration of the individual constituents was varied by diluting the commercial sample by means of PBS buffer solutions (10 mM, pH 7.2). In order to reduce volume errors due to dilution, all solutions were always prepared by dilution of the eye drops and not by subsequent dilutions. This means that if C0 is the initial concentration of any solution constituent, after dilution the concentration turns out to be |
 | (15) |
α being the dilution factor.
As regard vitamin B2 the initial concentration was found to be C0 = 1.3 mM.
3.3 Methods
3.3.1 Spectrophotometry. The absorption measurements were carried out with a cary 100-Varian UV-Vis equipped with thermostatted cuvettes. Samples were placed in a rectangular quartz cuvettes of 1 cm path length and absorption spectra were recorded at (25 ± 0.5) °C in the 200–800 nm wavelength region.
4 Results and discussion
4.1 Absorption spectra
Fig. 1 shows absorption spectra of a commercial eye defence measured at 25 °C as a function of the dilution factor C/C0. As one can see, each spectrum is characterized by one peak in visible light range at 445 nm and three peaks in UV range at 220, 266, 373 nm, respectively. Absorption of light in the UV-visible region is due to electronic transitions between ground state and excited state valence electrons. The complex shape of RBF spectra displayed in Fig. 1 is due to the superposition of rotational and vibrational transitions with electronic transitions, which gives a jumble of overlapping lines appearing as a continuous absorption band.14 Comparing the wavelengths of experimental peaks with the physiological ranges of UV radiation, one realizes that λ = 371 nm falls in the UVA range while other two peaks lie in the UVC range. Therefore, the effect of RBF concentration was studied by monitoring, as a function of C/C0, absorbance peaks at 370 and 260 nm as well as at 300 nm. Results are displayed in Fig. 2. An detailed numerical analysis indicates that experimental results are well-described by a saturation curve of the type: |
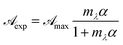 | (16) |
where
and mλ two adjustable parameters and α is given by eqn (15).
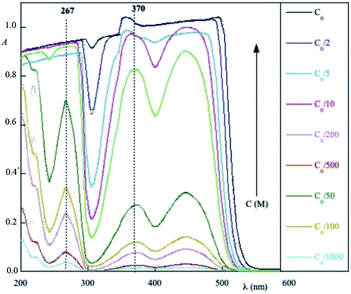 |
| Fig. 1 Experimental absorption spectra of commercial eye defence as a function of the dilution factor. In the column, on the right, the sample concentration expressed as a fraction of the initial concentration C0 = 1.3 mM is indicated. | |
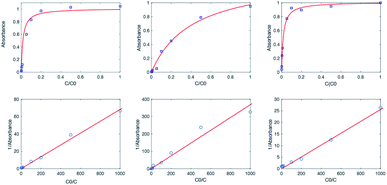 |
| Fig. 2 First row (from left to right): absorbance maximum of RBF molecules in eye defence, at 370, 267, 300 nm; second row (from left to right): reciprocal of vs. reciprocal of α for λ = 370, 267 and 300 nm. | |
The curve shape was further studied by plotting reciprocal of
vs. reciprocal of α, as shown in Fig. 2. As one can see, for all cases investigated, experimental data-points exhibit an excellent linearity. Hence, we are very confident that eqn (16) is a good representation of the experimental data. Curve-parameters computed by means of best-fitting to experimental data-points are collected in Table 1. It is noteworthy that
is independent of λ, while mλ is strongly dependent on the wavelength. Accordingly, experimental curves are substantially described by a single parameter, for any RBF concentration.
Table 1 Fitting parameters obtained by nonlinear least squares procedure applied to spectrophotometric results
λ (nm) |

|
mλ |
370 |
1.0 ± 0.2 |
58 ± 6 |
300 |
1.3 ± 0.5 |
2.9 ± 0.2 |
267 |
1.1 ± 0.1 |
21 ± 3 |
Introducing eqn (15) into (16),
is rewritten as
|
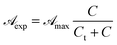 | (17) |
where
In this form it is immediately seen that there exist a threshold concentration which determines two regimes. For C < Ct,
increases linearly with slope
while for C > Ct it remains practically constant. Likely, at high concentrations, riboflavin molecules associate in dimers, trimers, n-mers forms, making the electrons less available to pass in excited states. Finally for C = Ct,
From the definition of Ct, it follows that threshold concentration depends on λ and C0. At first glance, therefore, one might be tempted to conclude that, at high C0, the eye drops should give an excellent UV absorption. A second look, however, shows that this conclusion is not justified because from Ct onwards the absorbance is constant, a large excess of RBF implies a large amount of unused substance. If, then, RBF undergoes oxidative degradation with formation of free radicals, one realizes that RBF quantity to be used must be calibrated in order that have small Ct and low probability of the oxidation reaction.
In this regard, it can seen from Fig. 1 that absorption spectra exhibit two minima around λ = 420 and 320 nm, which correspond to a greater transmittance. Since sunshine has a high emission at 420 nm, one could deduce that eye defence formulation is not very protective. However, it is equally evident from Fig. 1 how the spectrum is dependent on the riboflavin concentration, i.e. it is dose-dependent. This means that a suitable dosage of the formulation minimizes the transmittance effect at 420 nm. Particularly, for RBF concentration C = 0.5 g L−1 the absorption minima disappear almost completely. Furthermore, even if, at these wavelengths, the refractive index between the formulation and the eye surface were such as to reduce reflection the transmitted light would be negligibly low.
In concluding this section, we should note that the energy stored in the absorption process may be released by emitting a longer wavelength radiation. In short, absorbent molecules behave like fluorophores and the material is seen to be fluorescent. With regard to eye defence formulation, preliminary measurements on material samples have shown that an excitation at 450 nm produces a high intensity of fluorescence emission at 526 nm, strongly dependent on RBF concentration. Obviously, such emission represents a new source of radiation. Fortunately, 526 nm wavelength corresponds to the green color, which is not harmful to the eye, indeed may use to visualize some corneal lesions. That is to say, a eye treated with this formulation, when irradiated with white light, the green light emitted by fluorescence reveals a corneal lesions. Clinical research has been initiated on this point.
4.2 Numerical results
It is easy to convince oneself that whenever diffusion of RBF molecules occurs in cornea, it has a marked effect on the UV-transmittance of ocular tissue. It, perhaps, not quite so obvious that oxidative effects, when they occur are of major importance. The reason for this is that the extent of degradation is a strong function of the RBF concentration. Thus, the combined action of these two effects determines the UV-exposure response of the cornea treated with RBF-based eye drops. To model both actions we only have to introduce eqn (6) and (17) into (13) and to solve the integral. In order to apply the model numerically it is necessary to know the external parameters.
McQuaid et al.15 analyzed the penetration rate of RBF molecules through the stroma. Their measurements provide a diffusion coefficient D = 6.5 × 10−7 cm2 s−1. In a kinetic study of RBF photolysis in aqueous solution by UV-visible radiation, the apparent first-order rate constant, k, at different pH values was measured.16 Such experimental results were used to interpolate tR = 1/k = 0.91 × 105 s. By means of these experimental data the control parameter at p = 0.23 was determine, then the concentration profile as function of X,
can be calculated. Nevertheless, in order to evaluate the total transmittance it is necessary to know the penetration length of UV rays in cornea.17 From data by Kolozsvalári et al.17 was computed δp = 0.19 cm, for λ = 373 nm; δp = 0.0090 cm, for λ = 300 nm; and δp = 0.0269 cm, for λ = 266 nm. Finally, making use of results in Table 1, the transmittance was numerically calculated as a function which maps a pair of values (X,
) inside the cornea to the UVA-transmission. Results, visualized in Fig. 3 as three-dimensional plots, indicate that UVB radiation is rapidly attenuated and completely absorbed within X = 0.4, i.e. 40% of cornea length. UVC radiation is less rapidly attenuated, but anyhow it is absorbed within X = 0.90, that is to say 90% of cornea length. At investigated wavelengths, Δp is very small so that the pre-integral term in eqn (13) causes a very strong damping, regardless of the integral value. In other words, the chemical composition of the cornea protects it against UVB and UVC radiation. Accordingly, RBF-based eye drops have a negligibly small effectiveness in this UV range. In Fig. 3 one can see that UVA attenuation occurs along the whole cornea and absorption is significantly dependent on the RBF concentration. Such an analysis is general and may always be applied to determine the absorptive properties of the system under investigation. However, it is not practical to do so because the necessary integrations require that an analysis of 3D-plots be available. A more useful and alternative equation is needed for predictive calculation of absorbance in the cornea. Such equation is obtained by taking into account that Ct identifies two regimes with different absorptive characteristics.
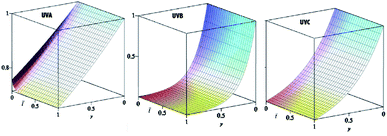 |
| Fig. 3 3D-plot of transmittance as a function of (X, ). | |
4.2.1 Low concentrations. In this concentration regime C(X,
) < Ct and eqn (17) is simplified into |
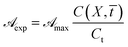 | (19) |
accordingly eqn (16) is analytically solved and results in |
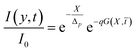 | (20) |
where |
 | (21) |
and |
 | (22) |
In eqn (20) the first exponential is the cornea contribution to UVA attenuation, the second one is the effect of eye drops. This latter depends both on the molecular properties of RBF and on its spatio-temporal evolution. Such contribution was studied by analyzing separately both the dependence on X and on
. In order to evaluate behavior as a function of time, we first divide X-axis in the region [0, 1] into intervals of size 0.1. Then Xn = n/10 defines points which span the X-axis contained within the cornea. The next step consists of applying eqn (20) for each fixed Xn. Results collected in Fig. 4 shown that, for each n, I/I0 decreases until it reaches a plateau, where the attenuation is saturated, For τD/τR = p2 ≪ 1 photo-dissociation reaction is slow compared with diffusion, implying that molar density of RBF inside the cornea is governed by on molecular diffusion. For example, with aid of Fig. 4, one finds that for n = 1 the attenuation saturates in 7 min, while for n = 0.5 after 30 min.
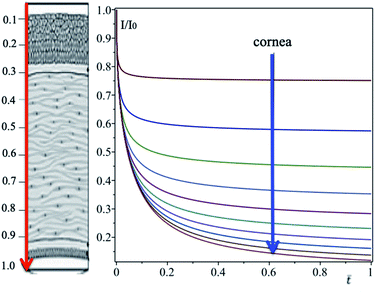 |
| Fig. 4 Corneal profile, from outside (epithelium) to inside (endothelium) according to the arrow, is divided into intervals of size X = 0.1. The transmittance on each interval is determined with eqn (20). | |
In order to calculate the transmittance as a function of X, the time
is probed at intervals of 0.1. Results displayed into Fig. 5 indicate that the transmittance saturates to large distances X. For long times all curves converge in a limit curve (blue curve in Fig. 5). This empirical result can also be analytically predicted by verifying that
|
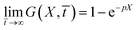 | (23) |
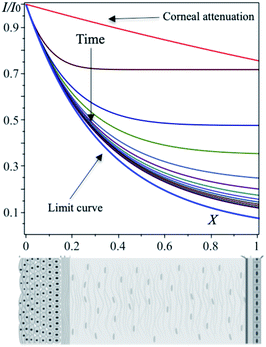 |
| Fig. 5 Corneal profile, from outside (epithelium) to inside (endothelium) according to the arrow, is divided into intervals of size X = 0.1. The transmittance on each interval is determined with eqn (20). | |
In Fig. 5, the attenuation of the cornea without eye drops is also plotted for comparison (red curve). Careful analysis of the plot makes it clear that eye drops significantly reduce transmittance. Indeed, at the center of the cornea, in the absence of eye drops, I/I0 = 0.85, while in the presence, after 1.2 h the transmittance becomes 0.21, with a net gain of 75%.
4.2.3 Transition point. This point is identified by the relationship C(X,
) = Ct. As one can derive from eqn (17), such relationship represents the condition for which within the cornea
Hence the transmittance becomes time independent, i.e. a steady-state is triggered. Eqn (7) and experimental results of Table 1 allow us to obtain Ct = 0.065 mM. Solutions of the equation C(X,
) = 0.065 determines a curve representing the contour line connecting those points where RBF concentration 0.065 nM. Numerical results are plotted in Fig. 6. As it can be seen, along the cornea, the limit value is reached at longer times. An equality of the form C(X,
) = CtCt. Fig. 6 shows that the limit concentration along the cornea is reached at different times, as a result of the molecular diffusion. From plot it is easily deduced that the end of the cornea experiences the limit value after 1.2 hours. In other words, in an eye drops-loaded the steady-regime is reached in 1 hour. Interestingly, the actual attenuation coefficient is s/2 and the corresponding transmittance becomes from this plot it is easily deduced that the end of the cornea experiences the limit value after 1.2 hours. In other words, in an eye drops-loaded the steady-regime is reached in 1 hour. Interestingly, in this point the eye drops-attenuation is q/2 so that the steady-attenuation region can be uniquely outlined: |
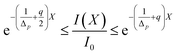 | (25) |
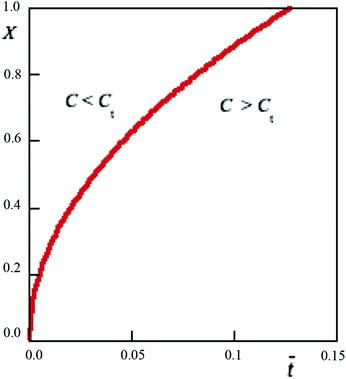 |
| Fig. 6 Limit curve separating two concentration regimes in which there are two different effect against UVA-rays. | |
5 Conclusions
When UV radiation strikes a corneal surface, some will be reflected in the air interface without entering the cornea. As radiation is propagated within the interior of cornea, it is attenuated by absorption of the corneal tissue. Thus the presence of RBF-based eye drops will decrease penetration of UV radiation. Mainly because of the presence of the UV-screening pigments, the gradients of UV radiation are steeper than those for visible radiation. The leaf epidermis is the first layer to be directly exposed to solar UV radiation. The penetration of UVB radiation within cornea is largely attenuated by the epidermal layer. UVA, UVB and UVC exert different effects on biological tissue, determined by their respective wavelengths. RBF-based eye drops are generally used to protect the eyes from UV radiation. Diffusion of RBF into cornea plays an important role in the eyes-protection both against UVA-radiation and oxidation process of the ocular tissue due to air-oxygen or a specific diet.18 In order to understand the mechanism of cornea protection exerted by vitamin B2 not only measurements of molecular absorption are sufficient, but it is necessary to develop an appropriate mathematical model to evaluate the level of attenuation of UV radiation in the ocular tissue. The diffusion–reaction approach herein developed combines experimental results with molecular diffusion model. Indeed, spectrophotometric measurements reveal that the commercial eye defence is ineffective for UVB and UVC. On the contrary, it is efficient for UVA protection.
A detailed numerical investigation of the experimental data shows that the attenuation as a function of RBF concentration is a saturation curve. This implies that there exist a threshold concentration, Ct, that identify two operating regimes. For C > Ct the system reaches the steady-state, which is reached at different times along the entire cornea. In practical terms this point is very important as it allows to predict the behavior of the eye drops and what will be its maximum protection against UVA rays.
One of the requirements for a successful model in physical-chemistry science is judicious simplification. If one wishes to do justice to all aspects of the problem, one very soon find oneself in a hopelessly complicated situation and thus, in order to make progress, it is necessary to ignore certain aspects of a chemical situation and to retain others; the wise execution of the choice often makes the difference between a result which is realistic and one which is merely academic. The semiempirical model herein discussed, is an attempt to choose simplifying assumptions which make the problem tractable and yet do not greatly violate chemical-physical reality. Indeed, from the kinetic results of photodegradation of RBF in aqueous solution, which takes place with the formation of free radicals, we estimate the half-life t = 18 h. In addition, the eye defence formulation contains sodium hyaluronate and vitamin E, which act as electron scavengers,19,20 therefore the residence time of RBF molecules in the reaction environment is even longer. Anyhow, such a time is certainly longer than the actual time of operating of the formulation, as experienced on patients. For any substance, regardless of mechanism, all kinetic properties of interest can be calculated from lifetime measurements. Finally, model tries to describe, in terms of basic concepts of physical chemistry, some results experienced in the ophthalmological field. We assume the diffusion as a driving force of RBF molecules through the cornea and their average residence time in the biological tissue is used to model side reactions, which can reduce the absorbent effect of the chromophores.
Conflicts of interest
There are no conflicts to declare.
Acknowledgements
We are grateful the Iros sc S.r.l. (Italy) for providing us with the eye drops Drop Defence used in this study.
Notes and references
- C. Caruso, R. L. Epstein, C. Ostacolo, L. Pacente, S. Troisi and G. Barbaro, Cornea, 2017, 36, 600–604 CrossRef PubMed.
- C. Ostacolo, C. Caruso, D. Tronino, S. Troisi, S. Laneri, L. Pacente, A. D. Prete and A. Sacchi, Int. J. Pharm., 2013, 440, 148–153 CrossRef CAS PubMed.
- S. Chaudhuri, S. Batabyal, N. Polley and S. Pal, J. Phys. Chem. A, 2014, 118, 3934–3943 CrossRef CAS PubMed.
- A. Budroni, C. Thomas and A. De Wit, Phys. Chem. Chem. Phys., 2017, 19, 7936–7949 RSC.
- L. Ambrosone, Phys. B, 2000, 292, 136–152 CrossRef CAS.
- C. Caruso, G. Barbaro, R. L. Epstein, D. Tronino, C. Ostacolo, A. Sacchi, L. Piacente, A. D. Prete, M. L. Sala and S. Troisi, Cornea, 2016, 35, 659–662 CrossRef PubMed.
- J. Francis and D. Dickton, J. Nutr. Health Food Eng., 2015, 2, 221–223 Search PubMed.
- M. A. Sheraz, S. H. Kazi, S. Ahmed, Z. Anwar and I. Ahmad, Beilstein J. Org. Chem., 2014, 10, 1999–2012 CrossRef PubMed.
- L. Ambrosone, V. Vitagliano, C. D. Volpe and R. Sartorio, J. Solut. Chem., 1991, 20, 17199–17207 CrossRef.
- S. Alterovitz and M. Gershenson, J. Comput. Phys., 1975, 19, 121–133 CrossRef.
- F. Venditti, G. Bufalo, F. Lopez and L. Ambrosone, Chem. Eng. Sci., 2011, 66, 5922–5929 CrossRef CAS.
- C. Yang, J. Qiu and J. Wang, Abstr. Appl. Anal., 2014, 36, 1–8 CAS.
- R. B. White and F. F. Chen, Plasma Phys., 1974, 16, 565–587 CrossRef.
- A. Puglisi, T. Giovannini, L. Antonov and C. Cappelli, Phys. Chem. Chem. Phys., 2019, 21, 15504–15514 RSC.
- R. McQuaid, M. Mrochen and B. Vohnsen, J. Cataract Refract. Surg., 2016, 42, 462–468 CrossRef PubMed.
- I. Ahmad, Q. Fasihullah, A. Noor, I. A. Ansari and Q. M. Ali, Int. J. Pharm., 2004, 280, 199–208 CrossRef CAS PubMed.
- L. Kolozsvalári, A. Nógrádi, B. Hopp and Z. Bor, Investig. Ophthalmol. Vis. Sci., 2002, 43, 2165–2168 Search PubMed.
- M. Mosca, L. Ambrosone, F. Semeraro, D. Casamassima, F. Vizzarri and C. Costagliola, Int. J. Food Nutr. Sci., 2014, 65, 235–240 CrossRef CAS PubMed.
- C. Ke, L. Sun, D. Qiao, D. Wang and X. Zeng, Food Chem. Toxicol., 2011, 49, 2670–2675 CrossRef CAS PubMed.
- H.-Y. Zhang and H.-F. Ji, New J. Chem., 2006, 30, 503–504 RSC.
|
This journal is © The Royal Society of Chemistry 2020 |
Click here to see how this site uses Cookies. View our privacy policy here.