DOI:
10.1039/D0RA00060D
(Paper)
RSC Adv., 2020,
10, 3832-3836
Single crystals of mixed Br/Cl and Sn-doped formamidinium lead halide perovskites via inverse temperature crystallization†
Received
7th August 2019
, Accepted 13th January 2020
First published on 22nd January 2020
Abstract
Hybrid organic–inorganic perovskite mixed halides of FAPbBr3−xClx and doped FAPb1−xSnxBr3 were synthesized using a generalized inverse temperature crystallization (ITC) method. With an appropriate choice of solvents and crystallization temperatures we show that large millimeter sized single crystals of these hybrid perovskites can be grown in a matter of hours to days using ITC. The structural and optical properties of these single crystals were characterized systematically. The mixed metal and mixed halide perovskites displayed a compositional bandgap tuneability in the region of 2.05 eV to 2.57 eV. The electrical properties of the perovskite single crystals were determined using a space-charge limited current (SCLC) method. The trap density determined from SCLC was between 109 and 1011 cm−3 for all perovskites which is exceptionally low. The mobility was found to increase by one order of magnitude on the addition of only 3% Sn for FAPb1−xSnxBr3 based perovskites which shows promise for enhancing the electrical properties. This demonstrates the generalizability of the ITC method to grow large high-quality perovskite single crystals with enhanced optical and electrical properties. In addition, it was observed for FAPbBr3−xClx based perovskites that initially degraded surfaces with suppressed PL emission could be repaired by using an anti-solvent treatment re-enabling the PL emission. Other perovskite compounds did not display any degraded surfaces and exhibited excellent stability in ambient conditions.
Introduction
In recent years, organic–inorganic halide perovskites (AMX3) have been the focus of intense research due to their remarkable optical and electrical properties.1–6 Recently, single crystal organic–inorganic halide perovskites have been shown to exhibit significantly longer diffusion lengths, higher mobilities and lower trap densities than polycrystalline thin films.7–9 These enhanced properties make single crystals attractive for further improving optoelectronic device performance. Typically single crystals have been grown using cooling induced precipitation (CIP),10,11 seeded solution growth,3 or vapour-assisted crystallisation,8 however these methods typically take weeks to months to prepare high quality crystalline samples.
Inverse temperature crystallisation (ITC) is method which takes advantage of the observed retrograde solubility of perovskites, allowing rapid growth of high-quality, millimetre-sized perovskites in a matter of days.7,12,13 Based on the ITC approach single crystal materials of MAPbX3 (MA = methylammonium and X = halide),7,13,14 FAPbX3 (FA = formamidinium),12,15,16 CsPbBr3,17–19 MAPbBr3−xClx,20–23 MAPbBr3−xIx21,23–26 and heterovalent doped MAPbBr3
27 and MAPbCl3
28 have been reported. It has been shown that substitution of MA for FA can lead to improved stability in solar cells29 and enhanced charge transport properties in thin films30,31 and single crystals16 compared to their MAPbX3 counterparts. However, formamidinium based mixed single crystals have yet to be reported. Here we report the successful synthesis of large, millimetre-sized, single crystal perovskites containing mixed halides of FAPbBr3−xClx and doped metals of FAPb1−xSnxBr3 with low trap densities using ITC. The incorporation of both mixed halides and doped metals into the single crystal perovskites permits tuning of the band gap across the visible spectrum and alters its electrical properties. While investigating air stability of these materials, FAPbBr3−xClx based single crystals were found to exhibit a degraded surface species that could be repaired using an anti-solvent treatment (see ESI†).
Experimental
Chemicals and reagents
Lead bromide (PbBr2, ≥98%), lead chloride (PbCl2, 98%), N,N-dimethylformamide (DMF, anhydrous, 99.8%), dimethyl sulfoxide (DMSO, anhydrous, ≥99.9%), tin(II) bromide (SnBr2) and methylamine solution (33 wt% in absolute ethanol) were purchased from Sigma-Aldrich. Methylammonium bromide (MABr) and formamidinium bromide (FABr) were purchased from Dyesol Limited. γ-Butyrolactone (GBL, 99+%) was purchased from Acros Organics. All precursors were used as received without any further purification.
Synthesis
In general solutions were prepared by dissolving AX and MX2 precursors in a suitable solvent (DMF/DMSO/GBL). After the precursors have dissolved the solution is filtered using a 0.22 μm PTFE hydrophilic filter. 2 mL of the solution is than placed into a glass vial with a 21G needle and heated in oil bath. Further details on specific temperatures, reaction conditions and initial precursor concentrations can be found in the ESI.†
Measurement and characterization
Powder X-ray diffraction was performed on a Panalytical X'Pert Pro diffractometer using a Cu-Kα radiation. The steady state absorption was carried out on a Varian Cary 50 UV-vis spectrophotometer. Photoluminescence emission and decay were carried out on a Horiba Jobin Yvon Fluorolog/Fluorohub setup, a xenon lamp was used as the source for PL emission and a 265 nm NanoLed was used as the source for TCSPC. EDS elemental analysis was carried out using a Jeol JSM-6610 SEM. I–V characteristics were carried out in the dark under ambient conditions with an Agilent 4156C Precision Semiconductor Parameter Analyser.
Results and discussion
Synthesis and characterization
Unlike MAPbBr3−xClx there are no reports of FAPbBr3−xClx single crystals as far as we are aware. In order to grow large FAPbBr3−xClx single crystals, a 1
:
1 v/v mixture of DMF–GBL can still be used similar to pure FAPbBr3.12 However, due to the lower solubility of PbCl2 in DMF, the concentration and crystallisation temperature must be decreased with the increasing PbCl2 inclusion to optimise the crystal growth. Using the optimised reaction conditions (Table S1, ESI†), large single crystals of FAPbBr3−xClx were grown in just a few days. Photographs of the single crystals with a total synthesis time of 20 hours and a total synthesis time > 48 hours can be found in Fig. 1A and B, respectively. It was observed that for reactions with a total synthesis time of 20 hours the resulting single crystals were orange, yellow and pale yellow for FAPbBr2.69Cl0.31, FAPbBr2.23Cl0.77 and FAPbBr1.33Cl1.67 respectively. These results are similar to those observed for MAPbBr3−xClx based perovskites (Fig. S2, ESI†). However, if the total synthesis times were increased to >48 hours then the single crystals were observed to be very dark orange. This suggests that an increase in the synthesis time under ambient conditions can lead to degradation of FAPbBr3−xClx. Powder XRD spectra were collected for samples with a total synthesis time of 20 hours and are shown in Fig. 1D and the corresponding lattice constants are shown in Table 1. For FAPbBr3−xClx perovskites the diffraction peaks shift to higher angles with increasing Cl content thus indicating a decrease in the lattice constant due to the inclusion of the smaller Cl atomic radius. The powder XRD spectra were also collected for the degraded samples (Fig. S3, ESI†). The powder XRD spectra showed no difference between the samples with the same nominal Cl inclusion irrespective of the total synthesis time. Furthermore, upon grinding the samples for XRD the resulting powders were observed to have a similar colour regardless of the total synthesis time (Fig. S4, ESI†). This suggests that the increased synthesis time has resulted in the formation of degraded surface species, see the ESI† for a more detailed discussion on the degraded surface and how it can be repaired using a simple anti-solvent treatment. Energy dispersive X-ray spectroscopy (EDS) was used to identify the actual composition of the mixed halides and mixed metals present in the single crystals. The elemental compositions are summarised in Table 1. We can see that the nominal Cl inclusion is close to that determined using EDS. This also resembles the observations for MAPbBr3−xClx analogues grown using ITC (Table S2, ESI†), where no preferential incorporation of Br or Cl is observed. Single crystals of MAPb1−xSnxBr3 have been recently reported via the TSSG method,32 motivated by this report we also attempted to synthesize mixed Pb/Sn single crystals using ITC. Using DMF–GBL (1
:
1 v/v) as a solvent, perovskites of FAPb1−xSnxBr3 were successfully achieved by substituting an equal molar ratio of PbBr2 for SnBr2 and using and inert atmosphere to prevent Sn oxidation. Specific reaction conditions can be found in the ESI.† Photographs of the FAPb1−xSnxBr3 perovskites are shown in Fig. 1C. We can see that with increasing Sn inclusion the crystals appear from orange for pure Pb, to dark red for mixed metals (1% and 3% of Sn). The powder XRD spectra are shown in Fig. 1D. The diffraction peaks for FAPb1−xSnxBr3 based perovskites are shifted to slightly higher angles with increasing Sn inclusion resulting in a smaller lattice constant as summarized in Table 1. The actual composition of Sn in the doped metal perovskites are shown in Table 1. We can see that the actual percentage of Sn incorporated into the perovskite is only between 1–3%. This shows a preferential incorporation of Pb relative to Sn in the FAPb1−xSnxBr3 based perovskites, only allowing dopant level incorporation of Sn to be achieved using ITC. Similar percent level dopants of Sn are also observed for MA analogues that we synthesized using ITC (Table S2, ESI†), and as such this method appears to be generally less useful than TSSG for preparing mixed metal single crystals.
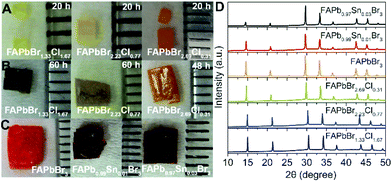 |
| Fig. 1 Photographs of FAPbBr3−xClx (A) based perovskites with a total synthesis time of 20 hours and (B) with a total synthesis time > 48 hours showing a degradation of the surface with increased synthesis time. (C) Photographs of FAPb1−xSnxBr3 based perovskites. (D) Powder XRD spectra of FA based perovskites with different halide and metal compositions. | |
Table 1 Summary of elemental composition determined from EDS and lattice constants determined from XRD for FAPbBr3−xClx and FAPb1−xSnxBr3 single crystals
Samples |
Lattice constant (Å) |
Nominal Cl or Sn inclusion (in solution) |
Actual Cl or Sn inclusion (via EDS) |
Perovskites placed in anti-solvent (dichloromethane) for 4 months to repair the crystal surface. Perovskites placed in anti-solvent (chloroform) for 1 month to repair the crystal surface. |
FAPbBr3 |
6.03 |
— |
— |
FAPbBr2.69Cl0.31a |
5.99 |
16.7% (Cl) |
10.3% (Cl) |
FAPbBr2.23Cl0.77a |
5.90 |
33.3% (Cl) |
25.6% (Cl) |
FAPbBr1.33Cl1.67b |
5.86 |
50.0% (Cl) |
55.7% (Cl) |
FAPb0.99Sn0.01Br3 |
6.02 |
25.0% (Sn) |
1.0% (Sn) |
FAPb0.97Sn0.03Br3 |
5.98 |
50.0% (Sn) |
3.0% (Sn) |
Optical characterization
The absorption and PL spectra for FAPbBr3−xClx single crystals are shown in Fig. 2A. The absorption edges are blue-shifted with increasing Cl content and show a similar trend to the photographs. The absorption edge for perovskites with different synthesis times also appear blue shifted, suggesting that the observed differences in the photographs of the single crystals are indeed a surface effect which is discussed in more detail in the ESI.† Similar to MAPbBr3−xClx based perovskites the PL emissions are blue-shifted but otherwise show similar trends to the absorption spectra with increasing Cl content (Fig. S7, ESI†). The blue shifted (see ESI† for more detail) PL peak is similar to other perovskite single crystals grown using CIP.11 With this in mind, Tauc plots (Fig. S8, ESI†) are used to extract an estimate of the band gaps. The estimated band gaps for mixed Br/Cl single crystals are shown in Fig. 2B. We can see a rough linear trend with increasing Cl content. In general, the FAPbBr3−xClx perovskites show a slight red shift compared to their MAPbBr3−xClx analogues.
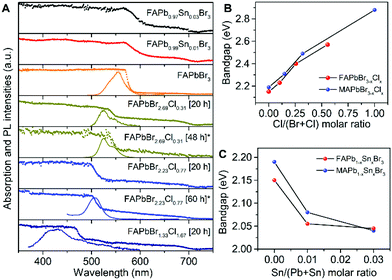 |
| Fig. 2 Absorption and PL spectra for (A) FA based perovskites with mixed halides or mixed metals. *Absorption and PL spectra collected for samples with degraded surface (total synthesis time > 48 hours). Band gap of (B) mixed Br/Cl perovskites and (C) mixed Pb/Sn perovskites extrapolated from Tauc plots. | |
UV-vis absorption spectra are shown in Fig. 2A for the tin-doped FAPb1−xSnxBr3 single crystals. We can see that the incorporation of only 1–3% Sn results in a much broader absorption edge which is red-shifted relative to pure FAPbBr3. We observe a similar trend for MA analogues as shown in Fig. 2C. No PL emission was observed for the doped Sn single crystals, ostensibly due to an increased number of trap states (relative to pure FAPbBr3, see Fig. 3) causing non-radiative recombination.
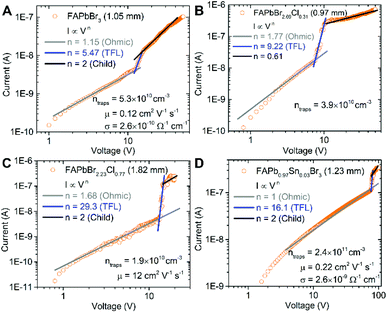 |
| Fig. 3 I–V curves of (A) FAPbBr3, (B) FAPbBr2.69Cl0.31, (C) FAPBr2.23Cl0.77, and (D) FAPb0.97Sn0.03Br3 perovskite single crystals with regions corresponding to SCLC theory. The crystal thickness is givens in the parenthesis and the conductivities are calculated from the ohmic regime, the trap densities are calculated from the TFL and the mobilities are determined from the Child's regime. | |
Electrical characterization
The current–voltage (I–V) properties were measured using a sandwich type device (Au/perovskite/Au) structure with two Au (100 nm) electrodes. Under an applied bias the dark current should follow the space charge limited current model (SCLC),33,34 which displays three distinct regimes. It should be noted that not all the single crystal perovskite materials exhibited all three distinct regions, as is shown in Fig. 3. Nevertheless the SCLC method has been consistently used to measure single crystal properties in the literature, despite some limitations.3,7,8,13–16,19,35 See the ESI† for a more detailed discussion on SCLC theory and extracting electrical properties.
Conductivities are largely comparable with literature with the exception of FAPbBr3, which has a conductivity of 2.0 × 10−10 S cm−1, about two orders of magnitude lower than previous reports on other FA single crystals.13,16 This may be due to degradation of the perovskite surfaces upon exposure to humid conditions. The addition of 1–3% Sn in FAPb1−xSnxBr3 has resulted in up to a 2 order of magnitude increase in the conductivity, and this trend is also observed for MAPb1−xSnxBr3 analogues prepared by ITC (Fig. S10, ESI†). In general, the trap density of our single crystal perovskites is between 109–1011 cm−3 which is significantly lower than their thin film counterparts and comparable to previous reports on perovskite single crystals (Fig. S11 and Table S4, ESI†).3,7,8,13–16,19,35,36 For FAPb1−xSnxBr3 based perovskites the inclusion of only several percent of Sn has resulted in an increase in trap density by 1 order of magnitude and MAPb1−xSnxBr3 perovskites also exhibit similar trends (Fig. S10, ESI†). The hole mobilities for FAPbBr3 were found to be 0.12 cm2 V−1 s−1 which appears to be lower than that determined previously,16 perhaps in part related to the observed degradation at the surface of these FA based perovskites. In the case of FAPbBr2.23Cl0.77 the mobility is 12 cm2 V−1 s−1 which is two orders of magnitude higher than pure FAPbBr3. This suggests that Cl inclusion may be a useful approach to enhance the mobility. In the case of FAPb0.5Sn0.5Br3, we find a mobility of 0.22 cm2 V−1 s−1. This is a moderate increase compared to MA based analogues which exhibits a two order of magnitude increase for 3% Sn inclusion (Fig. S10, ESI†).
Conclusion
In summary, the ITC method was successfully generalized to synthesize large single crystals of FAPbBr3−xClx and doped FAPb1−xSnxBr3 mixed perovskites with only minor variations in preparation technique. Structural and optical characterizations were carried out to obtain a highly tuneable bandgap for mixed halide and doped metal single crystal perovskites grown using ITC. In addition, FAPbBr3−xClx single crystals with degraded surfaces were successfully repaired using a simple anti-solvent treatment, leading to enhanced PL emissions. The conductivity was found to increase with the inclusion of only 1–3% Sn for FAPb1−xSnxBr3 based perovskites, similar to their MA analogues. Overall the trap density for our single crystals perovskites is between 109–1011 cm−3 which is exceptionally low. The mobility of the mixed halide and doped metal perovskites increased upon both Cl and Sn inclusion, suggesting that Cl and Sn inclusion could lead to an enhancement in the charge transport properties for FA based single crystals. This demonstrates that ITC can be generalized to grow a wide variety of high quality formamidinium perovskite single crystals in a matter of days.
Conflicts of interest
There are no conflicts to declare.
Acknowledgements
The authors acknowledge funding from HKUST via fund R9398 and via the School of Science (SSCI) fund IGN17SC05. Authors also recognize the MacDiarmid Institute for Advanced Materials and Nanotechnology, as well the Royal Society of New Zealand via grant E2646/3416. JEH acknowledges funding via a Rutherford Discovery Fellowship, E2675/2990.
Notes and references
- G. C. Xing, N. Mathews, S. Y. Sun, S. S. Lim, Y. M. Lam, M. Gratzel, S. Mhaisalkar and T. C. Sum, Science, 2013, 342, 344–347 CrossRef CAS PubMed.
- S. D. Stranks, G. E. Eperon, G. Grancini, C. Menelaou, M. J. P. Alcocer, T. Leijtens, L. M. Herz, A. Petrozza and H. J. Snaith, Science, 2013, 342, 341–344 CrossRef CAS PubMed.
- Q. F. Dong, Y. J. Fang, Y. C. Shao, P. Mulligan, J. Qiu, L. Cao and J. S. Huang, Science, 2015, 347, 967–970 CrossRef CAS PubMed.
- C. C. Stoumpos, C. D. Malliakas and M. G. Kanatzidis, Inorg. Chem., 2013, 52, 9019–9038 CrossRef CAS PubMed.
- C. S. Ponseca, T. J. Savenije, M. Abdellah, K. B. Zheng, A. Yartsev, T. Pascher, T. Harlang, P. Chabera, T. Pullerits, A. Stepanov, J. P. Wolf and V. Sundstrom, J. Am. Chem. Soc., 2014, 136, 5189–5192 CrossRef CAS PubMed.
- S. De Wolf, J. Holovsky, S. J. Moon, P. Loper, B. Niesen, M. Ledinsky, F. J. Haug, J. H. Yum and C. Ballif, J. Phys. Chem. Lett., 2014, 5, 1035–1039 CrossRef CAS PubMed.
- M. I. Saidaminov, A. L. Abdelhady, B. Murali, E. Alarousu, V. M. Burlakov, W. Peng, I. Dursun, L. F. Wang, Y. He, G. Maculan, A. Goriely, T. Wu, O. F. Mohammed and O. M. Bakr, Nat. Commun., 2015, 6, 7586 CrossRef PubMed.
- D. Shi, V. Adinolfi, R. Comin, M. J. Yuan, E. Alarousu, A. Buin, Y. Chen, S. Hoogland, A. Rothenberger, K. Katsiev, Y. Losovyj, X. Zhang, P. A. Dowben, O. F. Mohammed, E. H. Sargent and O. M. Bakr, Science, 2015, 347, 519–522 CrossRef CAS PubMed.
- Y. H. Shao, Z. G. Xiao, C. Bi, Y. B. Yuan and J. S. Huang, Nat. Commun., 2014, 5, 5784 CrossRef CAS PubMed.
- Y. Y. Dang, Y. Liu, Y. X. Sun, D. S. Yuan, X. L. Liu, W. Q. Lu, G. F. Liu, H. B. Xia and X. T. Tao, CrystEngComm, 2015, 17, 665–670 RSC.
- Y. J. Fang, Q. F. Dong, Y. C. Shao, Y. B. Yuan and J. S. Huang, Nat. Photonics, 2015, 9, 679–686 CrossRef CAS.
- M. I. Saidaminov, A. L. Abdelhady, G. Maculan and O. M. Bakr, Chem. Commun., 2015, 51, 17658–17661 RSC.
- G. Maculan, A. D. Sheikh, A. L. Abdelhady, M. I. Saidaminov, M. A. Hague, B. Murali, E. Alarousu, O. F. Mohammed, T. Wu and O. M. Bakr, J. Phys. Chem. Lett., 2015, 6, 3781–3786 CrossRef CAS PubMed.
- Y. C. Liu, Z. Yang, D. Cui, X. D. Ren, J. K. Sun, X. J. Liu, J. R. Zhang, Q. B. Wei, H. B. Fan, F. Y. Yu, X. Zhang, C. M. Zhao and S. Z. Liu, Adv. Mater., 2015, 27, 5176–5183 CrossRef CAS PubMed.
- Q. F. Han, S. H. Bae, P. Y. Sun, Y. T. Hsieh, Y. Yang, Y. S. Rim, H. X. Zhao, Q. Chen, W. Z. Shi, G. Li and Y. Yang, Adv. Mater., 2016, 28, 2253–2258 CrossRef CAS PubMed.
- A. A. Zhumekenov, M. I. Saidaminov, M. A. Haque, E. Alarousu, S. P. Sarmah, B. Murali, I. Dursun, X. H. Miao, A. L. Abdelhady, T. Wu, O. F. Mohammed and O. M. Bakr, ACS Energy Lett., 2016, 1, 32–37 CrossRef CAS.
- P. K. Nayak, D. T. Moore, B. Wenger, S. Nayak, A. A. Haghighirad, A. Fineberg, N. K. Noel, O. G. Reid, G. Rumbles, P. Kukura, K. A. Vincent and H. J. Snaith, Nat. Commun., 2016, 8, 13303 CrossRef PubMed.
- Y. Rakita, N. Kedem, S. Gupta, A. Sadhanala, V. Kalchenko, M. L. Bohm, M. Kulbak, R. H. Friend, D. Cahen and G. Hodes, Cryst. Growth Des., 2016, 16, 5717–5725 CrossRef CAS.
- M. I. Saidaminov, M. A. Haque, J. Almutlaq, S. Sarmah, X. H. Miao, R. Begum, A. A. Zhumekenov, I. Dursun, N. Cho, B. Murali, O. F. Mohammed, T. Wu and O. M. Bakr, Adv. Opt. Mater., 2017, 5, 1600704 CrossRef.
- T. Y. Zhang, M. J. Yang, E. E. Benson, Z. J. Li, J. van de Lagemaat, J. M. Luther, Y. F. Yan, K. Zhu and Y. X. Zhao, Chem. Commun., 2015, 51, 7820–7823 RSC.
- Y. C. Liu, X. D. Ren, J. Zhang, Z. Yang, D. Yang, F. Y. Yu, J. K. Sun, C. M. Zhao, Z. Yao, B. Wang, Q. B. Wei, F. W. Xiao, H. B. Fan, H. Deng, L. P. Deng and S. Z. Liu, Sci. China: Chem., 2017, 60, 1367–1376 CrossRef CAS.
- H. T. Wei, D. DeSantis, W. Wei, Y. H. Deng, D. Y. Guo, T. J. Savenije, L. Cao and J. S. Huang, Nat. Mater., 2017, 16, 826–833 CrossRef CAS PubMed.
- W. F. Wang, J. Su, L. Zhang, Y. Lei, D. Wang, D. Lu and Y. Bai, CrystEngComm, 2018, 20, 1635–1643 RSC.
- Y. X. Zhang, Y. C. Liu, Y. J. Li, Z. Yang and S. Z. Liu, J. Mater. Chem. C, 2016, 4, 9172–9178 RSC.
- H. R. Byun, D. Y. Park, H. M. Oh, G. Namkoong and M. S. Jeong, ACS Photonics, 2017, 4, 2813–2820 CrossRef CAS.
- F. Y. Zhang, B. Yang, X. Mao, R. X. Yang, L. Jiang, Y. J. Li, J. Xiong, Y. Yang, R. X. He, W. Q. Deng and K. L. Han, ACS Appl. Mater. Interfaces, 2017, 9, 14827–14832 CrossRef CAS PubMed.
- A. L. Abdelhady, M. I. Saidaminov, B. Murali, V. Adinolfi, O. Voznyy, K. Katsiev, E. Alarousu, R. Comin, I. Dursun, L. Sinatra, E. H. Sargent, O. F. Mohammed and O. M. Bakr, J. Phys. Chem. Lett., 2016, 7, 295–301 CrossRef CAS PubMed.
- Z. Zhang, L. X. Ren, H. Yan, S. J. Guo, S. H. Wang, M. Wang and K. X. Jin, J. Phys. Chem. C, 2017, 121, 17436–17441 CrossRef CAS.
- G. E. Eperon, S. D. Stranks, C. Menelaou, M. B. Johnston, L. M. Herz and H. J. Snaith, Energy Environ. Sci., 2014, 7, 982–988 RSC.
- F. C. Hanusch, E. Wiesenmayer, E. Mankel, A. Binek, P. Angloher, C. Fraunhofer, N. Giesbrecht, J. M. Feckl, W. Jaegermann, D. Johrendt, T. Bein and P. Docampo, J. Phys. Chem. Lett., 2014, 5, 2791–2795 CrossRef CAS PubMed.
- W. Rehman, R. L. Milot, G. E. Eperon, C. Wehrenfennig, J. L. Boland, H. J. Snaith, M. B. Johnston and L. M. Herz, Adv. Mater., 2015, 27, 7938–7944 CrossRef CAS PubMed.
- D. X. Ju, Y. Y. Dang, Z. L. Zhu, H. B. Liu, C. C. Chueh, X. S. Li, L. Wang, X. B. Hu, A. K. Y. Jen and X. T. Tao, Chem. Mater., 2018, 30, 1556–1565 CrossRef CAS.
- A. Rose, Phys. Rev., 1955, 97, 1538–1544 CrossRef CAS.
- R. H. Bube, J. Appl. Phys., 1962, 33, 1733–1737 CrossRef CAS.
- H. S. Rao, W. G. Li, B. X. Chen, D. B. Kuang and C. Y. Su, Adv. Mater., 2017, 29, 1602639 CrossRef PubMed.
- B. Wenger, P. K. Nayak, X. M. Wen, S. V. Kesava, N. K. Noel and H. J. Snaith, Nat. Commun., 2017, 5, 590 CrossRef PubMed.
Footnote |
† Electronic supplementary information (ESI) available. See DOI: 10.1039/d0ra00060d |
|
This journal is © The Royal Society of Chemistry 2020 |